DOI:
10.1039/D1NR01190A
(Review Article)
Nanoscale, 2021,
13, 7879-7896
Multi-stimuli-responsive aggregation of nanoparticles driven by the manipulation of colloidal stability
Received
22nd February 2021
, Accepted 11th April 2021
First published on 13th April 2021
Abstract
The capacity to control the dispersed or aggregated state of colloidal particles is particularly attractive for facilitating a diverse range of smart applications. For this reason, stimuli-responsive nanoparticles have garnered much attention in recent years. Colloidal systems that exhibit multi-stimuli-responsive behaviour are particularly interesting materials due to the greater spatial and temporal control they display in terms of dispersion/aggregation status; such behaviour can be exploited for implant formation, easy separation of a previously dispersed material or for the blocking of unwanted pores. This review will provide an overview of the recent publications regarding multi-stimuli-responsive microgels and hybrid core–shell nanoparticles. These polymer-based nanoparticles are highly sensitive to environmental conditions and can form aggregated clusters due to a loss of colloidal stability, triggered by temperature, pH and ionic strength stimuli. We aim to provide the reader with a discussion of the recent developments in this area, as well as an understanding of the fundamental concepts which underpin the responsive behaviour, and an exploration of their applications.
1. Introduction
Nanoparticle (NP) suspensions which are sensitive to changes in the chemical and physical environment are one of the latest developments of so-called smart materials. The colloidal stability of smart NPs can be manipulated through changes in various stimuli, allowing for an often reversible transition between stable dispersion and aggregated clusters. Stimuli-responsive NP aggregation while often a property that is deliberately avoided in many colloidal systems, is actually an appealing behaviour for many applications. For example triggered aggregation behaviour has been exploited in applications such as drug delivery,1–3 membrane blocking,4 recovery/responsive control of colloidal catalysts,5 nanosensing,6,7 and enhancing oil recovery.8,9 In these application areas, ability to form aggregates or large assemblies of nanoparticles on demand offers the potential for implant formation, easy removal of a previously dispersed system or the blocking of unwanted pores. Temperature-responsive NPs have received particular interest as the stimulus is easily applied and the behaviour of temperature-responsive polymers is typically well-understood, particularly in the case of one of the most common temperature-responsive polymers, poly(N-isopropylacrylamide) (PNIPAM).10 Recently, there has been a drive towards multi-stimuli-responsive NPs whose temperature-responsive behaviour is modulated by a secondary/tertiary stimuli such as pH and ionic strength. The multi-stimuli-responsive character of NPs provides an increased degree of control over the response of the NPs, affording increased versatility and suitability to deliver more complex behaviour and thus benefits for a range of applications.11–13 A particular application area of interest for responsive NPs is in biomedical applications, where stimuli such as temperature, pH and ionic strength have significance, as these stimuli vary across physiological environments.
The stimuli-responsive nature of dispersed NPs is derived from changes in the colloidal stability of the particles (which will be discussed in more detail in section 3). The colloidal stability of NPs is derived from the surface chemistry of the particles, as such, a common feature of all multi-stimuli-responsive NPs is a particle architecture that facilitates surface changes of the particles. There are two typical particle structures that are common for stimuli-responsive NPs, core–shell NPs and microgel particles. Core–shell NPs are hybrid particles consisting of a solid, hard-sphere core (e.g. metal, metal oxide, silica, glassy polymer) and a dense polymer brush layer forming the shell.14 The polymer brush layer is usually formed through covalent bonding to the surface of the core by graft-to15 or graft-from16 methodologies. On the other hand, microgel particles are cross-linked polymer networks which swell in a good solvent but can reversibly de-swell in response to changes in the surrounding conditions.17,18 All the NPs discussed in this review are responsive to changes in temperature, pH and/or ionic strength, and as a result can undergo an often reversible transition between a dispersed and aggregated state. This review article will provide the reader with an overview of recent developments and examples of their application, underpinned by discussions of the interactions between colloidal NPs and the processes of destabilisation which result in aggregation.
2. Stimuli-responsive polymers
Stimuli-responsive polymers undergo changes in their physicochemical properties as a function of external stimuli. Polymers have been shown to exhibit responsive behaviour to a variety of stimuli such as temperature,19–22 pH,23,24 ionic strength,25 light,26,27 enzymes28,29 and redox conditions.30–32 For the purpose of this review, only polymers responsive towards temperature and pH stimuli shall be discussed. Ionic strength is also a stimulus which influences polymer properties, and its effect on the colloidal stability of NPs through ionic screening will be covered in this review. We direct readers to a recent review by Zhao et al.30 for a broader discussion of a range of stimuli-responsive polymers for smart NPs.
2.1. Temperature-responsive polymers
Temperature-responsive polymers undergo significant changes in solubility as a critical solution temperature is passed. At the critical solution temperature, polymers experience an abrupt shift in the balance of hydrophilic/hydrophobic interactions, resulting in a shift in solubility. This solubility change can be observed in various solvents but is most commonly observed in aqueous conditions. Therefore, discussion of solubility throughout this review should be assumed as aqueous solubility unless stated otherwise. Polymers with a lower critical solution temperature (LCST) hydrogen bond with water at low temperatures.33 However, upon heating, polymer–polymer interactions become more favourable than the polymer–water interactions, and begin to dominate above the LCST. As a result, polymer chains undergo conformational change from a solvated coil to a collapsed globule structure (coil-to-globule transition) and precipitate from the aqueous continuous phase. Polymers with an upper critical solution temperature (UCST) display opposing behaviour, switching from hydrophobic at low temperatures to hydrophilic above the UCST. LCST polymers have broadly received more interest as many possess a transition temperature close to body temperature, hinting potential suitability for a range of biomedical applications.19,34 For this reason, PNIPAM is by far the most intensely studied temperature-responsive polymer, with an LCST of ∼32 °C.10 However, there are a wide variety of temperature-responsive polymers with varying LCSTs, such as: poly(N-vinylcaprolactam) (PVCL), LCST ∼ 32 °C;35 poly(vinyl methyl ether) (PVME), LCST ∼ 37 °C;36 poly(N-ethyl oxazoline) (PEtOx), LCST ∼ 65 °C;37 and poly(oligo(ethylene glycol)methyl ether methacrylate) (POEGMA), LCST 26–90 °C38 (the LCST is highly dependent on the length of the oligo(ethylene glycol) side chains). Cross-linked polymer networks, such as microgels, undergo swelling and conformational changes at the volume phase transition temperature (VPTT) – a parameter which can be considered analogous to the LCST of linear chains.39 Below the VPTT, the polymer network is hydrophilic and is swollen in water. As the temperature increases above the VPTT polymer–polymer interactions become dominant and water is expelled as the cross-linked network dramatically de-swells.18
2.2. pH-Responsive polymers
pH-Responsive polymers can be regarded as either weak polyacids or weak polybases, whose degree of ionisation is dependent on their pKa and the solution pH.23 In a highly ionised state, the charged groups along the polymer chain promote an extended coil conformation due to enhanced hydrophilicity and intra-chain electrostatic repulsion. As the degree of ionisation decreases in response to changes in pH or ionic strength, hydrophobic interactions begin to dominate, with the loss of intra-chain repulsion allowing polymers to collapse into a globule conformation. Thus, through the pH-modulated degree of ionisation, the polymer solubility and conformation can be regulated. Weak polyacids typically contain carboxylic or phosphoric acid functionalities, which are protonated at low pH and dissociate to become the anionic analogue as pH increases above the pKa. The inverse behaviour is observed with weak polybases, which predominantly utilise tertiary amine functionalities to accept protons and become polycationic at low pH. Therefore, the pH range where the coil-to-globule transition occurs is largely determined by the balance of hydrophilic/hydrophobic interactions and the pKa of the ionisable functionality.40
3. Dispersed and aggregated states of colloidal systems
3.1. Colloidal stability of nanoparticle suspensions
The stimuli-responsive aggregation behaviour of NPs is driven by control of colloidal stability and by utilising the natural propensity of NPs to aggregate. This tendency for nanoparticles to aggregate is due to the innate attractive interactions (London dispersion force) between particles and their high specific surface area, which may be associated with a considerable contribution to the free energy. Therefore, understanding the interaction of forces which dictate the colloidal stability is central to the design of these responsive systems. Colloidal stability describes a system where NPs remain well dispersed in the continuous phase, avoiding aggregation and sedimentation. The dispersed or aggregated state of a colloidal NP can generally be attributed to the pair interaction energy, the value of which is equal to the sum of attractive and repulsive forces between two NPs. Derjaguin–Landau–Verwey–Overbeek (DLVO) theory considers the pair interaction energy to be the balance of the attractive London dispersion force and the repulsive electrostatic force of the ionic double layer.41,42 In actuality, the delicate interplay of several additional forces must be considered, including steric and electro-steric repulsion, as well as solvation and hydrophobic interactions.43 Core–shell NPs attain colloidal stability through the swollen polymer brush layer providing steric stabilisation when well-solvated by the continuous phase. Upon Brownian collision of two sterically stabilised particles, interpenetration and compression of solvated polymer chains is met with a steep entropic energy barrier, due to a localised increase in osmotic pressure.44 By this mechanism, core–shell NPs can remain well-dispersed over long periods of time. Swollen microgel particles often also assume a core–shell structure,17,45,46 possessing a dense and highly cross-linked core and a more loosely cross-linked outer shell with chain ends extending into the continuous phase – so called “dangling ends”.47 The structure of microgels naturally imparts good colloidal stability. The low density of the particles results in a weak attractive potential,17,48 whilst dangling chain ends facilitate steric stabilisation. The presence of ionised groups, e.g. polyelectrolyte, produces the electro-steric stabilisation of NPs. Ionised groups provide an electrostatic charge and promote a swollen microgel or brush layer through inter-/intra-chain repulsion and increased solubility.
3.2. Driving forces for aggregation
The stimuli-responsive aggregation is discussed in this review is induced by triggering destabilisation of colloidal NPs, whereby the repulsive force(s) which previously stabilised the NPs are suppressed and a shift in the balance of interactions results in attractive forces emerging dominant. Fig. 1 provides an illustration of the various stimuli-responsive destabilisation mechanisms discussed in this section.
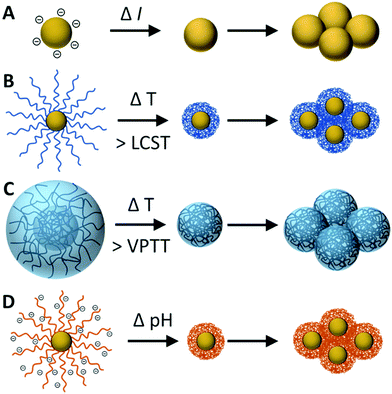 |
| Fig. 1 Illustration of various responsive NP aggregation processes: (A) ionic screening of electrostatically stabilised NP (I = ionic strength); (B) temperature-responsive loss of steric stabilisation and subsequent aggregation of core–shell NPs above LCST (T = temperature); (C) pH-responsive loss of electro-steric stabilisation and subsequent aggregation of core–shell NPs; (D) temperature-responsive de-swelling and aggregation of microgels above VPTT. | |
NPs which primarily rely on electrostatic repulsion to remain dispersed often result in metastable suspensions, with only a low kinetic energy barrier preventing aggregation. As a result, these systems can encounter problems with maintaining stability at high particle concentration and upon shearing.49 Electrostatic stabilisation is also heavily influenced by the presence of ions, as the electrostatic charge can be effectively screened at sufficient ionic strength. The critical coagulation concentration (CCC) describes the threshold ionic concentration at which electrostatic stabilisation is screened, resulting in a loss of colloidal stability and NP aggregation,50–52 see Fig. 1A.
To provide effective steric stabilisation, polymer chains must remain well-solvated in the continuous phase to provide a sufficiently thick barrier.53 Therefore, steric stabilisation can be switched “on” and “off” by a stimuli-responsive coil-to-globule transition. For example, core–shell NPs with a temperature-responsive polymer brush layer are sterically stabilised by a swollen brush layer at low temperatures. As the LCST is surpassed, the polymer brush collapses as a result of the coil-to-globule transition and steric stabilisation is lost (Fig. 1B).
Similarly, temperature-responsive microgels dramatically de-swell as temperature increases above the VPTT (Fig. 1C). Steric stabilisation is lost as dangling chain ends collapse,54 whilst the increased density of the de-swollen particle results in an increased attractive potential between particles.18 If the suppression of the steric component results in a loss of colloidal stability, aggregation shall follow.
Electro-steric stabilisation can be regulated in a comparable manner through the use of pH-responsive polymers. At a pH where the polymer is highly ionised and hydrophilic, NPs are stabilised by a swollen and charged brush layer or microgel structure. Upon pH-induced loss of these ionised moieties, hydrophobic interactions begin to dominate, leading to polymer collapse and simultaneous loss of electro-steric stabilisation, see Fig. 1D.
4. Multi-stimuli-responsive nanoparticle aggregation
The multi-stimuli-responsive behaviours of NPs that provide aggregation as a primary response to the stimuli (shown in Fig. 1) thus use a combination of the different mechanisms of colloidal stability. Such systems thus require the combination of two or more different stimuli to remove colloidal stability and result in NP aggregation. In the following sections, we will discuss the examples of systems that use different combinations of stimuli and their behaviour. Examples of the polymers that are commonly used in the multi-stimuli-responsive nanoparticle aggregation systems are shown in Table 1.
Table 1 Chemical structures of some of the common polymers used in multi-stimuli-responsive nanoparticle aggregation systems
Structure |
Name |
Abbr. |
Stimuli |
|
Poly(ethylene glycol) |
PEG |
Temperature |
|
Poly(N-isopropylacrylamide) |
PNIPAM |
Temperature |
|
Poly(2-(2-methoxyethoxy)-ethyl methacrylate) |
PMEO2MA |
Temperature |
|
Poly(N-vinyl caprolactam) |
PNVCL |
Temperature |
|
Poly(2-(dimethylamino) ethyl methacrylate) |
PDMAEMA |
Temperature and pH |
|
Poly(acrylic acid) |
PAA |
pH |
|
Poly(methacrylic acid) |
PMAA |
pH |
|
Poly(2-succinyloxyethyl methacrylate) (PSEMA) |
PSEMA |
pH |
4.1. Temperature- and ionic strength-responsive nanoparticle aggregation
The preparation of dual temperature- and ionic strength-responsive NPs involves the incorporation of temperature-responsive polymers and strongly ionised functionalities. Strongly ionised moieties are a requirement as they largely avoid the influence of pH. Below the LCST and at low ionic strength, electro-steric repulsion produces a well-dispersed NP suspension. As temperature increases above the LCST and the ionic strength surpasses CCC, both steric and electrostatic components can be individually suppressed (through the mechanisms discussed in section 3.2.). Therefore, the dual-stimuli-responsive NPs discussed in this section require the influence of both temperature and ionic strength stimuli to lose colloidal stability and aggregate.
Numerous studies have reported the influence of ionic strength on the LCST of temperature-responsive polymers, with findings typically showing an increase in ionic strength results in a decrease of the LCST due to the salting-out effect, i.e. an increased salt concentration results in a poorer solvent environment.55–57 Zámbó et al. utilised this effect to facilitate the aggregation of gold nanoparticles (AuNPs) with a grafted poly(ethylene glycol) (PEG) brush layer (Fig. 2A).58 The temperature-responsivity of PEG homopolymers is often dismissed as the LCST usually far exceeds 100 °C in water, limiting its usability.59 However, they found with a low molecular weight PEG brush layer (<2000 g mol−1) and the addition of ionic strength, the LCST could be reduced. In 0.2 M K2SO4 solution, aggregation of PEG-coated AuNPs was observed at 60 °C, decreasing to 50 °C in 0.3 M solution (Fig. 2B). Rasmusson and Vincent also observed the influence of ionic strength on the LCST when investigating the dual-stimuli-responsivity of PNIPAM microgels with anionic sulfate chain ends, derived from the potassium persulfate (KPS) initiator used in the synthesis.60 They also found the aggregation temperature decreased as salt concentration increased – from 39.5 °C in 0.027 M NaCl solution, to 25.5 °C in 0.8 M NaCl solution. Below the NaCl concentration of 0.025 M, the ionic strength was insufficient to screen the electrostatic charge of the sulfate chain ends, allowing microgels to remain dispersed above the VPTT.61
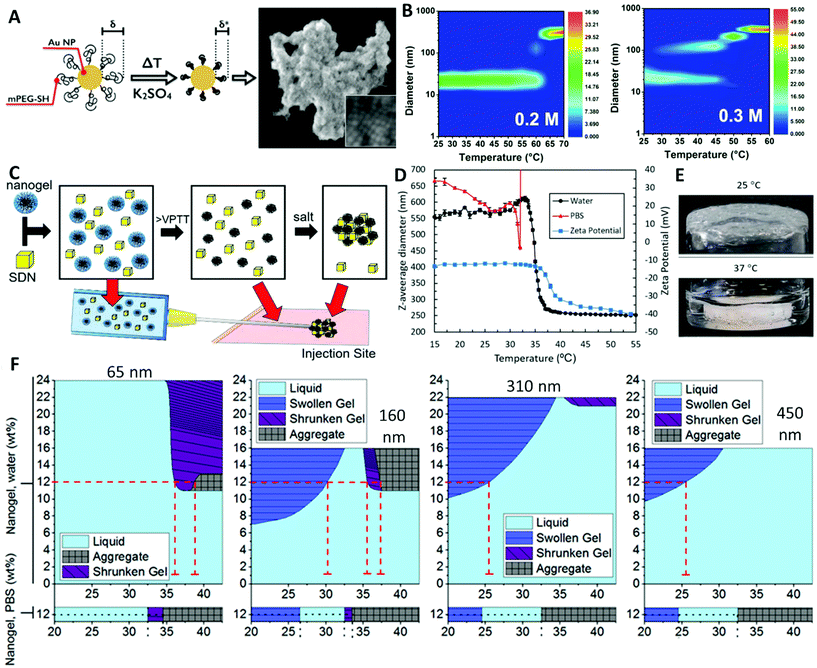 |
| Fig. 2 Examples of systems demonstrating temperature- and ionic strength-responsive nanoparticle aggregation behaviour. (A) AuNPs with a grafted PEG brush layer showing temperature and ionic strength responsive aggregation. (B) DLS size distribution of AuNPs with increasing temperature and ionic strength obtained with either 0.20, or 0.30 M potassium sulfate. The colour bars represent the intensity distribution as measured by DLS; the vertical cuts of these intensity maps give the size distributions of the samples at the corresponding temperature.58 (C) Use of the combined stimuli of temperature and increased ionic strength to trigger the aggregation of PNIPAM microgels that would function as a drug delivery implant. (D) Characterisation of the behaviour of the microgels; mean diameter by DLS in both water and PBS, with the zeta potential of the microgels (in water only). The rapid increase in diameter of the nanogels when heated to ≥32 °C in PBS was due to microgel aggregation. (E) The microgels aggregated into a single monolith upon heating to 37 °C in PBS.3 (F) Study of the effect of temperature, microgel concentration and ionic strength on the responsive behaviour of differently sized microgels (mean diameter at 25 °C 65, 160, 310 and 450 nm). (A) and (B) adapted with permission from Zámbó et al.58 (C)–(F) adapted with permission from Town et al.62 | |
Our group also recently synthesised dual temperature- and ionic strength-responsive PNIPAM-based microgels for use in sustained drug release applications (Fig. 2C)3 – discussed further in section 5 (Application). The microgels were prepared by precipitation polymerisation using a KPS initiator, resulting in anionic sulfate chain ends. Dynamic light scattering (DLS) was used to observe the stimuli-responsive behaviour of the PNIPAM-based microgels by measuring changes in their hydrodynamic diameter. This analysis revealed that the microgels de-swelled above the VPTT (32 °C) but remained dispersed in water due to electrostatic stabilisation provided by the anionic sulfate chain ends. Indeed, the de-swelling of the microgels lead to an increase in the surface charge, with the zeta potential values becoming increasingly negative at the VPTT as the charge was condensed onto a smaller surface area. Repeating these measurements at physiological ionic strength using phosphate buffered saline (PBS) showed slight de-swelling on the fringe of the VPTT, with the average size of the dispersed microgels dramatically increasing as the microgels aggregated above the VPTT (Fig. 2D). Thereby, demonstrating the requirement of both temperature and ionic strength stimuli to lose colloidal stability and induce aggregation, which was crucial for the nature of the application.3 Heating the microgels from 25 °C to 37 °C in the presence of PBS resulted a transition from a water swollen gel to a phase separated gel due to the complete aggregation of the microgels (Fig. 2E). The swollen gel morphology was formed due to the volume blocking and jamming of the swollen microgels, preventing the NPs moving past one another. A subsequent study focused on the effect of the average diameter (65, 160, 310 and 450 nm) and concentration of PNIPAM microgels on their aggregation behaviour and morphological transitions.62 In dilute dispersions (0.1 wt%), the temperature-responsive behaviour of PNIPAM was found to be independent of microgel size, as all samples displayed similar de-swelling behaviour in water and aggregation behaviour in PBS at ∼33 °C by DLS. However, more concentrated microgel dispersions showed varying morphological transitions upon temperature increase, depending on the ionic strength, the microgel concentration and the average particle size (Fig. 2F). If the microgels did not occupy a sufficient volume fraction to lead to volume blocking and jamming of the NPs then the sample was able to flow as a liquid. Therefore, liquids were typically observed at lower concentrations of swollen microgels and up to much higher concentrations above the VPTT of the NPs when the microgels were deswollen/collapsed. The swollen gels were self-supporting gels formed by the volume blocking and jamming of the swollen microgels. Shrunken gels were also self-supporting gels but were formed above the VPTT, when the microgels were in the deswollen/collapsed state. In this case, the self-supporting nature of the shrunken gels occurred when there was close association of the microgels, which was balanced by sufficient electrostatic repulsion to prevent complete aggregation of the microgels, allowing network formation. Finally, if there was insufficient electrostatic repulsion between the particles then complete aggregation occurred which was seen as the formation of phase-separated aggregates, these samples were not self-supporting and had a large excess of water visible. In water, below 7 wt% all the microgel produced were free flowing liquid morphologies, even above the LCST of PNIPAM. Concentrated dispersions (>12 wt% in water) of the two smallest microgel samples (60 and 160 nm) could form partially aggregated shrunken gel networks and phase-separated aggregates when heated above the VPTT in water. Whereas for the larger microgel samples (310 and 450 nm), aggregation was not possible in water at the temperatures and concentrations tested, due to the strong electrostatic stabilisation indicated by their high zeta potential values (−33 mV and −37 mV respectively for 310 and 450 nm microgel samples).63 All microgel samples formed phase-separated aggregates above the VPTT in PBS at 12 wt%, as the ionic strength was sufficient to screen the electrostatic repulsion of the charged chain ends. Oscillatory rheology was used to characterise morphology transitions, through measurements of the storage modulus (G′) and the loss modulus (G′′) that corresponded to the phase and morphology changes that were visually observed. These findings show the controllable morphology and rheological properties of PNIPAM-based microgels which can be achieved through aggregation behaviour in response to temperature and ionic strength stimuli.
Dual-stimuli-responsive core–shell NPs were reported by Zhang et al., who synthesised PNIPAM via reversible addition–fragmentation chain-transfer (RAFT) polymerisation and grafted the polymer chains to citrate-functionalised AuNPs via ligand exchange.64 At room temperature these NPs produced a red suspension due to the localised surface plasmon resonance (LSPR) peak at 529 nm, typical for dispersed AuNPs.65 When heated above the LCST in water aggregation was not observed. It was determined by zeta potential measurements that residual citrate units remained on the AuNP surface, providing electrostatic stabilisation above the LCST. The addition of 0.1 M NaCl was enough to screen this surface charge and result in reversible temperature-responsive aggregation. Aggregation was identified by DLS measurements and a red shift in the LSPR peak, typical for AuNPs within close proximity of each other.65,66 This publication therefore shows how controlled radical polymerization techniques can be useful in generating aggregating particle systems.
4.2. Temperature- and pH-responsive nanoparticle aggregation
NPs which can aggregate as a result of a synergistic interaction between temperature and pH are likely the most common type of multi-responsive NP currently studied. These NPs utilise the intrinsic responsive behaviour of the stabilising polymers to regulate electro-steric repulsion, whilst the interplay between temperature- and pH-responsivity can result in varied transitions between dispersed and aggregated states.
4.2.1. Dual-stimuli-responsive homopolymers for nanoparticle aggregation.
Dual-responsive homopolymers, such as poly(2-(dimethylamino) ethyl methacrylate) (PDMAEMA), possess a pH-modulated LCST which can be utilised to facilitate temperature- and pH-responsive NP aggregation. PDMAEMA contains tertiary amine moieties which are weakly basic, with a pKa of ∼7–7.5.67,68 Accordingly, PDMAEMA is highly protonated at acidic pH and acts as a typical polyelectrolyte, being highly water-soluble and thus lacking temperature-responsivity at this pH.69 As pH increases through neutral to weakly basic conditions, the degree of ionisation declines and the hydrophobic interactions begin to increase. Due to this, PDMAEMA remains water-soluble below LCST but water-insoluble above. At high pH (generally pH ≥ 9) PDMAEMA can be considered entirely non-ionised and as a result, polymer–polymer interactions are dominant and the polymer is water-insoluble.70 Therefore, the LCST of PDMAEMA is highly dependent on the pH and can be used to impart dual-responsive aggregation of colloidal microgels71 and core–shell NPs.72 Dong et al. grafted a PDMAEMA brush layer from silica NPs via surface-initiated atom transfer radical polymerisation (ATRP).72 DLS measurements revealed the expected dual-responsivity, showing a decrease in the aggregation temperature from 48–53 °C at pH 6, to 28–35 °C at pH 8. At pH 9 the hydrophobicity of the PDMAEMA brush layer resulted in the aggregation of PDMAEMA coated silica NPs at room temperature. This large change in VPTT of these particles shows the need to investigate these systems thoroughly.
The molecular weight of PDMAEMA has also been recognised to hold influence over the LCST, and thus must be considered when designing these dual-stimuli-responsive NPs. A comprehensive study of the aqueous solubility properties of PDMAEMA was reported by Bütün et al. who observed the monotonic decrease of the cloud point of free PDMAEMA as molecular weight was increased.73 The cloud point was measured by turbidimetry analysis of PDMAEMA samples with varying number-average molecular weight (Mn). At pH 8, the cloud point could be tuned from 46 °C when Mn = 1450 g mol−1, to 32 °C when Mn = 53
000 g mol−1. This relationship can be utilised to further tune the dual-stimuli-responsive aggregation of PDMAEMA-based NPs. Dong et al. also utilised this relationship between molecular weight and LCST with PDMAEMA coated silica NPs where the temperature responsive behaviour could be tweaked by 5–7 °C by varying Mn between 136
000 g mol−1 and 231
000 g mol−1.72 However, there are limitations to this, as Mohammadi et al. found a low molecular weight PDMAEMA brush layer (Mn = 6280 g mol−1) could not exhibit temperature-responsive behaviour, regardless of pH.74 It is important to note that there are many examples of other homopolymer stabilized NP systems that are likely to aggregate in response to pH and temperature changes, however, many publications focus on the stabilization of these systems.
Another recent example of an aggregating homopolymer system stabilized silica nanoparticles using poly(sulfobetaine methacrylate) (PSBA) brushes.75 ATRP was used to synthesise varying molecular weight PSBA chains with different grafting densities. This had an effect on the temperature response, with UCST-style VPTT values increasing with increasing grafting density and molecular weight of the polymer. These particles were stable at pH 5 to pH 9, with Dh values around 100 nm for all particles, but above pH 9 the particles doubled and tripled in diameter indicating some level of particle flocculation. While at pH 1 the particle diameter increased by 8 times. However, the number of available dual-responsive homopolymers is limited, and as a result, the addition of a comonomer is often needed to achieve dual-stimuli-response.
4.2.2. Dual-stimuli-responsive copolymers for nanoparticle aggregation.
An approach most commonly taken for obtaining dual temperature- and pH-responsivity involves the copolymerisation of two or more monomers with orthogonal responsivity. These copolymers can present unique stimuli-responsive behaviour which can be fine-tuned through alterations in the monomers used, the ratio between monomers and the polymer architecture.70,76 Poly(acrylic acid) (PAA) and poly(methacrylic acid) (PMAA) are pH-responsive polyanions which are commonly copolymerised or interpenetrated with PNIPAM to produce dual-stimuli-responsive microgels,77–80 or help to stabilise Au or Ag nanoparticles.81,82 Al-Manasir et al. investigated the aggregation behaviour of P(NIPAM-co-AA) microgels, finding 6 mol% PAA was sufficient to impart dual-stimuli-responsive behaviour. Analysis by DLS revealed an enhanced swelling behaviour of P(NIPAM-co-AA) microgels below the VPTT, and a reduced de-swelling above the VPTT at pH ≥ 7.83 This behaviour can be attributed to the deprotonated carboxylic acid groups of PAA generating inter-/intra-chain repulsion, enhanced hydrophilicity and electrostatic stabilisation. However, aggregation occurred above the VPTT at pH 2, when the PAA units are protonated.
Aggregating microgels have also been synthesised by copolymerizing N-vinyl caprolactam (NVCL) with AA or itaconic acid (IA).86 DLS data obtained at increasing temperatures displayed a decrease in the Dh, as the microgels deswelled due to the thermoresponsive behaviour of PNVCL. The swelling ratio obtained from this data (ratio of Dh at the highest and lowest temperature values) decreased when the concentration of AA or IA was increased. Additionally, the inclusion of the ionisable groups on the AA or IA resulted in increased in hydrodynamic diameter as the pH was increased, attributed to electrostatic repulsion of AA or IA units. Below pH 4, the microgels aggregated due to the lack of charge from the comonomers (as shown by zeta potential values trending to zero). However, it was possible for the particles to be redispersed when the pH was increased, likely due to the generation of electrostatic interactions between the microgels.
Core–shell NPs can utilise either random copolymer or block copolymer brush layers to produce a varied and diverse collection of dual-responsive systems. Shi et al. grafted a block copolymer of temperature-responsive PNIPAM and pH-responsive poly(2-succinyloxyethyl methacrylate) (PSEMA) – P(NIPAM-b-SEMA) – from AuNPs by surface-initiated ATRP.87 PSEMA contains a carboxylic acid functionality which facilitates its pH-responsive behaviour. At pH ≥ 7.5, the PSEMA block maintained the colloidal stability when the PNIPAM block collapsed above the LCST. Decreasing the pH from 7.5 to 5.5 was accompanied by a change in the zeta potential from −27 mV to 0 mV, indicating the complete protonation of PSEMA at pH 5.5. Consequently, NPs aggregated at pH ≤ 5.5, although this was unexpectedly independent of temperature. This aggregation below the LCST was attributed to the protonated PSEMA which makes up the second block, doubling back and preferentially hydrogen bonding with the PNIPAM block. This preferential inter-block hydrogen bonding minimises the interactions with water, increasing the hydrophobicity and destabilising the NPs. Similar findings of inter-block hydrogen bonding were reported by Chen et al. who grafted P(NIPAM-b-AA) from a poly(styrene) core by a surface-initiated polymerisation.88 They similarly observed temperature-independent NP aggregation at pH 4 as a result of inter-block hydrogen bonding between PNIPAM and protonated PAA blocks. Another interesting example is this inter-block hydrogen bonding has been shown with a copolymer of oligo(ethylene glycol) diacrylate and methacrylic acid.89 This system had a LCST-type VPTT in water, showing pH dependence by aggregation at pH 1 and 3 but not at pH 5 in 150 mM NaCl solution. But these particles also display a UCST-type VPTT in ethanol, allowing the particles to disperse and swell in ethanol above 45 °C. This was also concentration dependant, with broader increases in transmittance seen with higher concentration dispersions. While this is review is focussed on aggregating systems in water, this article shows that the use of other solvent systems is also possible may enable new future applications.
More complex and varied aggregation behaviours have been achieved in recent years through the copolymerisation of dual-responsive polymers such a PDMAEMA and a second stimuli-responsive monomer. Thus, doubly temperature- and pH-responsive NPs can be prepared. Song et al. copolymerised dual-responsive PDMAEMA and temperature-responsive poly(2-(2-methoxyethoxy)-ethyl methacrylate) (PMEO2MA) via ATRP, and compared the responsive behaviour of AuNPs coated with either P(DMAEMA-b-MEO2MA) (Fig. 3A top) or P(MEO2MA-b-DMAEMA) (Fig. 3A bottom).84 As PMEO2MA has a LCST of ∼35 °C and PDMAEMA has a pH-dependent LCST, a two-step collapse of the polymer brush was observed upon heating (Fig. 3B), with maximum NP aggregation occurring close to the isoelectric point; pH 7.7 for AuNPs coated with P(DMAEMA-b-MEO2MA) (Fig. 3C) and 8.1 when the polymer coating was P(MEO2MA-b-DMAEMA) (Fig. 3D). A diverse range of pH-tuneable, double LCST aggregation processes were demonstrated by Chen et al., who grafted random copolymer P(DMAEMA-co-NIPAM) from the surface of silica NPs by one-pot surface-initiated ATRP.85 At pH 5, PDMAEMA units were protonated and heating resulted in a gradual decrease of turbidity, observed by UV-vis spectroscopy. This indicates some degree of polymer brush collapse (confirmed by DLS) but no aggregation due to the electrostatic charge of PDMAEMA (Fig. 3E). Heating in neutral conditions resulted in a three-step transition due to the sequential collapse of PNIPAM and then PDMAEMA units, followed by NP aggregation at higher temperatures (Fig. 3F). However, an unexpected two-step transition was observed at pH 10 as NPs weakly assembled due to interparticle association at ∼35 °C and then fully aggregated above 55 °C (Fig. 3G). This is likely due to a two-phase transition of grafted PNIPAM which has been reported prior.90 Note the DLS data shown in Fig. 3E–G are the measurements recorded for SiO2 NPs coated with P(DMAEMA17-co-NIPAM83), however similar data was found for 50
:
50 monomer ratios. While a comonomer may add more complexity to a system, the ability to have dual-responsive aggregating systems far outweighs this additional complexity.
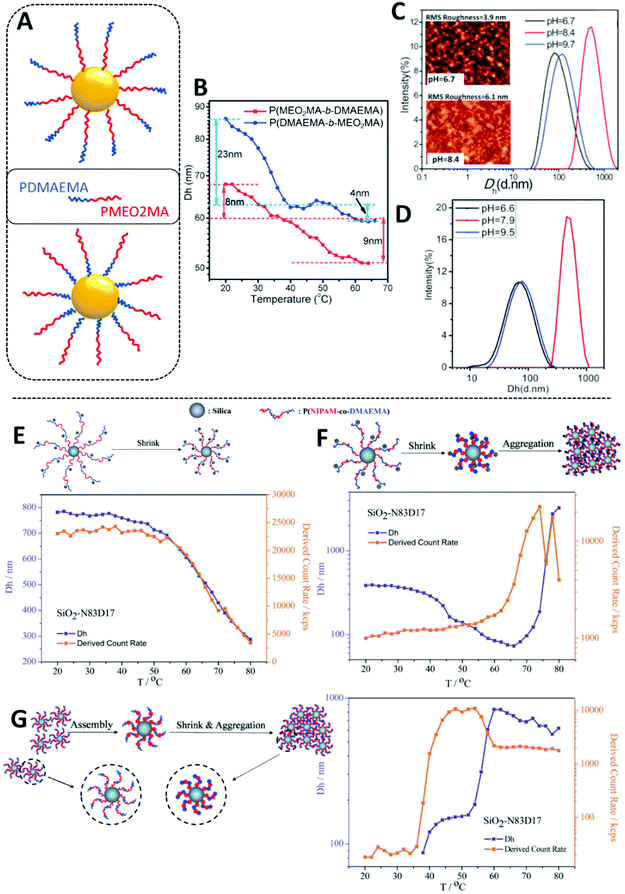 |
| Fig. 3 Examples of systems demonstrating temperature- and pH-responsive nanoparticle aggregation behaviour. (A) Graphical representation of dual-responsive AuNPs coated with either P(DMAEMA-b-MEO2MA) or P(MEO2MA-b-DMAEMA). (B) The correlation between Dh and temperature for P(MEO2MA-b-DMAEMA) and P(DMAEMA-b-MEO2MA) coated Au NPs; (C and D) the pH dependent Dh distributions of P(DMAEMA-b-MEO2MA) and P(MEO2MA-b-DMAEMA), respectively. (C) Includes AFM images inset; (E) temperature dependent hydrodynamic diameters and derived count rates of SiO2-g-P(NIPAM-co-DMAEMA) with proposed schematic illustration of the aggregation behaviour of modified silica nanoparticles at pH 5; (F) temperature dependent hydrodynamic diameters and derived count rates of SiO2-g-P(NIPAM-co-DMAEMA) with proposed schematic illustration of the aggregation behaviour of modified silica nanoparticles at pH; 7 (G) temperature dependent hydrodynamic diameters and derived count rates of SiO2-g-P(NIPAM-co-DMAEMA) with proposed schematic illustration of the aggregation behaviour of modified silica nanoparticles at pH 10. (A)–(D) adapted with permission from Song et al.84 (E)–(G) adapted with permission from Chen et al.85 | |
4.3. Temperature-, pH- and ionic strength-responsive nanoparticle aggregation
Multi-responsive NPs which are sensitive to all three stimuli (temperature, pH and ionic strength) yield even greater control over their dispersed/aggregated states. These systems combine the intrinsic temperature- and pH-responsive polymer behaviour with the ionic screening of charged surfactants or chain ends. Mohsen et al. synthesised multi-responsive P(NIPAM-co-AA), at 5 and 10 wt% AA monomer concentration, via surfactant-free emulsion polymerisation with a KPS initiator.4 A temperature-responsive de-swelling transition was observed for both microgels upon heating above the VPTT in water at pH 3. De-swelling was reduced at pH 6 due to electrostatic repulsion between anionic PAA units hindering a full collapse, which was more prominent in the 10 wt% PAA microgels. The de-swollen microgels remained colloidally dispersed above the VPTT at pH 3–6 due to electrostatic stabilisation from the sulfate chain ends. With the addition of electrolyte (0.1 M NaCl), both microgels reversibly aggregated when heated and cooled past the VPTT at pH 3. However, at pH 6 both microgels remained dispersed above the VPTT, even with the addition of ionic strength. Alongside the dependence upon all three stimuli to induce aggregation, Farooqi et al. reported the influence of ionic strength on the size of P(NIPAM-co-AA) microgel aggregates.91 DLS data obtained when heating P(NIPAM-co-AA) microgels with varying ionic strength at pH 9 showed no change in microgel size at any of the ionic strengths tested, while at pH 3 an increase in ionic strength from 0.05 M to 0.1 M NaCl solution resulted in a much larger aggregate formation. This can be attributed to the salting-out effect discussed in section 4.1.55 At pH 9, temperature-responsive de-swelling was inhibited by the ionised PAA, but ionic strength-responsive de-swelling could be seen with the microgels contracting as the increased ionic strength reduced the quality of the solvent environment.
Pinheiro et al. developed multi-stimuli-responsive core–shell microgels consisting of a poly(methyl methacrylate) (PMMA) core and a cross-linked P(NIPAM-co-AA) or P(NIPAM-co-MAA) shell (Fig. 4A).92 Core–shell microgels can be considered a hybrid between microgels and core–shell NPs, often possessing a denser core and a more loosely cross-linked polymer shell.93,94 Due to differences in reactivity ratios between NIPAM/AA and NIPAM/MAA, the two core–shell microgels incorporated the same bulk –COOH/–COO− content, but differed in its distribution.95–97 Thus, PMAA of P(NIPAM-co-MAA) resided mainly towards the PMMA core, whereas slower reacting PAA comonomer was distributed more towards the surface. This is represented graphically in Fig. 4A. The responsive behaviour of both core–shell microgels were measured primarily by DLS, Fig. 4B. The LCST values of these systems were obtained from Fig. 4B(i), which showed slight differences in the VPTT values observed: P(NIPAM-co-MAA) 30 °C; P(NIPAM-co-AA) 34 °C (at 0.005 wt%, pH 3.8). This is likely due to the surface PAA enhancing solvation of the cross-linked shell. Pinheiro et al. observed no aggregation above the VPTT at pH 5.5, for either P(NIPAM-co-AA) (Fig. 4B(ii)) or P(NIPAM-co-MAA) (Fig. 4B(iii)) despite high ionic strength (0.1 M NaNO3). This behaviour indicated that the degree of ionisation was too high at this point to be screened by ionic strength. The anionic charge of P(NIPAM-co-MAA) was found to be fully screened at lower ionic strength (0.015 M NaNO3, pH 5.5) compared to P(NIPAM-co-AA) coated particles (0.1 M NaNO3, pH 5). This was thought to be due to the higher charge density in P(NIPAM-co-MAA), compared with P(NIPAM-co-AA), whose charge is more difficult to screen as PAA is distributed towards the surface and occupies a larger area. It was found that at pH 5 microgel aggregation was induced for the PAA containing particles above the VPTT due to the ionic strength (0.1 M NaNO3) being sufficient to screen the anionic charge from ionised PAA, Fig. 4B(iv). This differed to the findings of Mohsen et al., who found high ionic strength was insufficient to screen ionised PAA at pH 6, this difference likely demonstrates the delicate interplay of interactions. The difference in salts used in the two studies (NaCl vs. NaNO3) may also influence the screening and salting-out abilities.56,57 However, no data is given for PMAA containing particles. Ultimately both of the core–shell microgels showed multi-responsive aggregation dependent on temperature, pH and ionic strength, however the distribution of charge does allow for slight variations in the aggregating conditions.
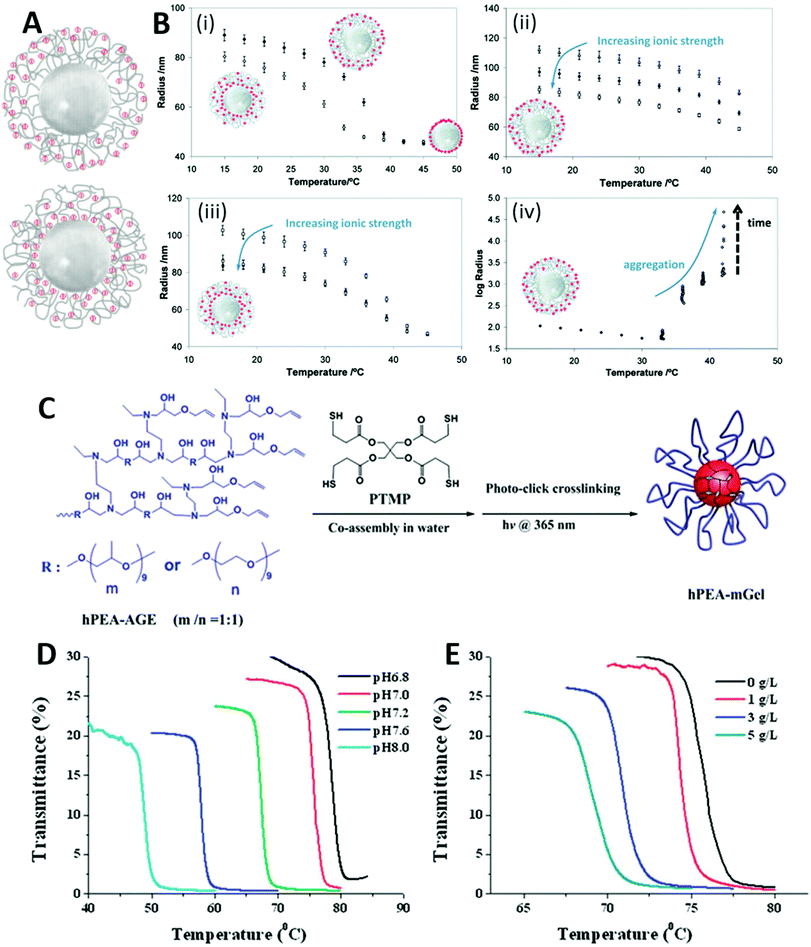 |
| Fig. 4 Examples of systems demonstrating temperature-, pH- and ionic strength-responsive nanoparticle aggregation behaviour. (A) Graphical representation of the charge distributions of P(NIPAM-co-AA) [top] and P(NIPAM-co-MAA) [bottom] coated PMMA particles. (B) DLS measurements showing the temperature-dependent hydrodynamic radius of P(NIPAM-co-AA/MAA) coated PMMA particles in various conditions, each graph contains schematic representations of the charge distributions of NPs: (i) P(NIPAM-co-AA) = ◆, P(NIPAM-co-MAA) = ◊, 0.005 wt%, pH 3.8, [NaNO3] = 0.003 M; (ii) P(NIPAM-co-AA), pH 5.5, [NaNO3] = 0.003 M (△), 0.015 M (◆), 0.1 M (□); (iii) P(NIPAM-co-MAA), pH 5, [NaNO3] = 0.003 M (□), 0.015 M (▲), 0.1 M (○); (iv) P(NIPAM-co-AA), pH 3.5, [NaNO3] = 0.1 M. Data was collected over a 20 minutes period after stabilisation at each temperature. (C) Scheme of the synthesis of PEA microgels. (D) Optical transmittance at 500 nm against temperature for PEA microgels in aqueous media. (E) Optical transmittance at 500 nm against temperature for PEA microgels in varying concentrations of NaCl at pH 7. (A) and (B) reproduced with permission from Pinheiro et al.92 (C)–(E) reproduced with permission from Li et al.98 | |
Li et al. synthesised hyperbranched poly(ether amine) (PEA) microgels by assembly of PEA and pentaerythritol tetra(3-mercaptopropionate) (PTMP) in water and the subsequent crosslinking of these reagents via a thiol–ene photo-click reaction – see Fig. 4C.98 The resulting 250 nm particles (PDI 0.15) were comprised of a poly(ethylene oxide) (PEO) shell and a PTMP cross-linked hydrophobic poly(propylene oxide) (PPO) core. The PEO shell was determined to be temperature responsive and became hydrophobic at ∼70 °C at pH 7.2. This was determined from UV-Vis spectroscopy data using transmittance to determine the cloud point, and therefore the aggregation temperature, of the microgels. Increasing the pH decreased the aggregation temperature of the microgels, Fig. 4D. This was attributed to the deprotonation of amino groups resulting in an overall more hydrophilic polymer. Similar results were also seen with increasing ionic strength, Fig. 4E. Unfortunately, the effect of increasing both of these variables was not studied. Yin's group also published results on the separation of dyes using silica particles with a PEA shell.99–102 These particles are again responsive to temperature, pH and ionic strength, and the aggregation of these particles is observed. The greater number of aggregating particle systems that are discovered and the more in-depth knowledge we generate of them, the more possible applications of these systems will be discovered.
5. Applications
Multi-stimuli-responsive NPs can be designed to aggregate under a diverse set of conditions, offering a wide variety of potential applications. The following sections will discussion examples of multi-stimuli-responsive aggregation of nanoparticles for applications in nanomedicine, pore-blocking, separation and extraction, and colloidal catalysts.
5.1. Nanomedicine
Temperature- and pH-responsive NPs have received particular interest for the diagnosis and treatment of cancerous tumours, as slight differences in the tumour microenvironment compared with healthy tissue allows for site-specific aggregation.103 This application is the focus of a recent review by Yu et al., which we direct readers to read for more information on this specific application.104 More broadly, stimuli-responsive microgels are commonly utilised as biomaterials due to their typically good biocompatibility and permeability, indicating suitability for tissue engineering and drug delivery applications.105–108 The stimuli-responsive NP aggregation behaviour can be used to produce a (semi-) solid material that can be injected into the body in a minimally invasion manner. Our group recently utilised dual stimuli-responsive PNIPAM microgels with solid drug NPs to produce an in situ forming implant (ISFI).3 ISFIs are a low viscosity, injectable solution which transforms into a (semi-) solid depot in the body for sustained drug release109 – see Fig. 2C. Once injected into a tissue environment, the PNIPAM microgels aggregated in response to physiological conditions, entrapping solid drug NPs in the process and producing a drug depot. This system benefitted from the dual temperature- and ionic strength-responsivity of the microgels, as this avoided premature aggregation in the needle from the elevated temperatures during injection. Sustained release of a HIV drug (lopinavir) from the ISFI was observed over a 120-day period in vitro. Subsequent studies revealed drug release rate could be fine-tuned through the adjustment of microgel size and solid drug NP loading, enhancing the suitability for real world applicability.1 Kjøniksen et al. reported a similar system using PNIPAM-based microgels for the sustained release of naltrexone.110 They demonstrated naltrexone permeated microgels at low temperatures and became entrapped upon de-swelling and aggregation in physiological conditions. Sustained release could be prolonged by the copolymerisation of 1 mol% PAA, which increased affinity to the positively charged naltrexone without compromising aggregation. Although these findings are conceptually promising, considerations of biocompatibility and biodegradability of polymer materials persist as the main obstacle for fulfilling drug delivery applications.
5.2. Pore blocking
The aggregation of temperature-responsive PNIPAM-based microgels may also be exploited for pore blocking applications, such as for enhancing oil recovery from petroleum reservoirs. The oil recovery process involves injecting pumping material (often seawater) into oil-bearing rock, thus displacing the oil and forcing it out of the reservoir. The pumping material follows the path of least resistance through the most permeable layers of rock, often resulting in <50% yield.111,112 However, the process efficiency may be improved through the injection of dual-stimuli-responsive microgels which aggregate in response to the high temperatures of oil-bearing rock and the ionic strength of seawater.8,9 Aggregated NPs can block the highly permeable channels and redirect the pumping material to mobilise oil from less permeable rock. Mohsen et al. demonstrated the ability of PNIPAM and multi-responsive P(NIPAM-co-AA) microgels ability to block a 5 μm porous membrane by measuring flow rate against time (Fig. 5A).4 A decrease in flow rate by up to 96% was observed after 180 minutes, indicating the membrane was almost fully blocked by aggregated NPs. The authors suggested this pore blocking may be suitable for the treatment of dentin hypersensitivity, where ∼3 μm tubules in the teeth expose the nerve to the external environment.113
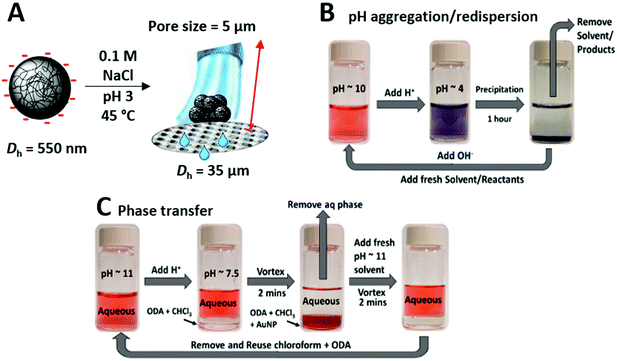 |
| Fig. 5 Examples of some of applications for which colloidal systems with multi-stimuli-responsive aggregation have been used. (A) Illustration of P(NIPAM-co-AA) particle aggregation for pore blocking. (B) Using responsive changes in the colloidal properties to enable catalyst separation. The catalyst NPs could be separated by either a pH triggered aggregation and redispersion, or (C) a pH-triggered phase transfer method using chloroform and phase transfer agent octadecylamine. (A) reproduced with permission from Mohsen et al.4 (B) and (C) reproduced with permission from Chakraborty et al.5,7 | |
5.2.1. Separation and extraction.
Favourable interactions between polymer-based NPs and certain compounds or proteins can result in their adsorption and encapsulation upon stimuli-responsive aggregation, which may prove useful for selective chemical separations or bioseparation applications.99 Di et al.100 demonstrated the capability of multi-stimuli-responsive core–shell NPs, consisting of a novel poly(ether amine) (PEA) grafted to a poly(dimethylsiloxane) (PDMS) core, for the selective separation of water-soluble dyes. Dyes could be separated from water upon aggregation of the NPs, producing coloured precipitates or transferring to an organic solvent phase, and leaving the aqueous phase almost colourless. Superparamagnetic NPs, such as iron oxide core–shell NPs, have received particular interest for separation applications, as the aggregate can be easily separated by magnetic extraction.114,115 For example, Paulus et al. integrated ion-exchange monomers into the PNIPAM polymer brush layer of a superparamagnetic core–shell NP, to act as binding sites for protein adsorption and extraction.116 This resulted in high protein adsorption (57–132 mg protein per g NPs) which remained unaffected upon heating to 40 °C, and close to 100% desorption could be achieved by elution with NaCl.
5.2.2. Colloidal catalysis.
Reversible stimuli-responsive NP aggregation has found interest in colloidal catalysis, as this may facilitate the recovery of the catalyst from the products/solvent and enhance reusability.5,117,118 Chakraborty and Kitchens recently prepared AuNPs with a grafted PAA shell to aid the recovery and reusability for multiple catalytic cycles of 4-nitrophenol reduction.5 The catalyst particles could be effectively recovered by two approaches: responsive aggregation and redispersion in fresh solvent/reagent mixture, or responsive phase transfer to an organic solvent, see Fig. 5B and C. In the responsive aggregation and redispersion approach, the use of a pH switch was used to trigger aggregation under acidic conditions which allowed the catalyst to then be easily separated from the solvent and products before being redispersed in fresh reactants. The phase transfer approach utilised the addition of acid to trigger a pH responsive transfer of the colloidal catalyst from the aqueous phase into an organic phase. This allowed easy separation of the products from the catalyst, the addition of base then resulted in the catalyst transferring back into the aqueous phase ready for reuse.
The activity of colloidal catalysts can also be modulated through responsive aggregation behaviour. Self-inhibition of catalytic activity can be achieved through aggregation in response to the evolving reaction conditions. For example, the stimuli-responsive collapse of the brush layer and subsequent aggregation of catalytic core–shell NPs can hinder activity by preventing reagent diffusion to the NP surface.119 Another approach involves embedding catalytic NPs within stimuli-responsive microgel matrices.120,121 The cross-linked polymer network supports the embedded NPs and assists their colloidal stability, whilst stimuli-responsive swelling and de-swelling behaviour regulates catalytic activity by hindering or facilitating the access of reagents to the surface of the embedded catalytic NPs. This regulation of reaction rate is particularly useful for avoiding overheating in the catalysis of exothermic reactions.119
6. Conclusions
To summarise, this review has provided an overview of the recent developments of stimuli-responsive core–shell NPs and microgels, which can form aggregates in response to the synergistic interplay between temperature, pH and ionic strength stimuli. The delicate balance of hydrophilic/hydrophobic interactions of the polymer-based NPs is key to achieving this responsive aggregation, as the balance can be shifted through changes in the external stimuli, leading to a loss of colloidal stability. As a result of multi-stimuli-responsivity, a complex and varied range of aggregation behaviours can be ascertained under potentially tightly defined conditions. Such spatial and temporal control of the aggregation behaviour offers the potential for such systems to fulfil a wide range of applications. These multi-responsive NPs are particularly interesting in the field of biomaterials, with temperature, pH and ionic strength stimuli all contributing to the physiological environment. The specificity of multi-responsive aggregating colloidal systems may also be further improved for biomedical application by designing in responsive elements that respond to biomarkers for disease. For example, enzymes have been previously shown to provide a single stimulus that triggered a change in the colloidal stability of NPs.122–124 In the future, the inclusion of such responsive elements into multi-responsive NP systems might offer the potential to trigger nanoparticle aggregation in response very tightly defined conditions, such as the presence of a certain enzyme while also in the appropriate pH or temperature range.
Although impressive progress has been made in this field over a short period of time, there are still shortcomings which may limit advances. PNIPAM heavily dominates the field of stimuli-responsive materials due to the LCST being within close proximity of body temperature. However, such PNIPAM based-systems are rarely degradable within the body which may limit their clinical suitability for some applications. This can be achieved either through the addition of degradable units within the polymer backbone or, for macro- or nanogels, the inclusion of a degradable cross-linker.125–131 Future research is required to develop PNIPAM polymers that are truly biodegradable, with predictable or tailorable degraded polymer molecular weights for excretion. Additionally, although PNIPAM-based NPs remain desirable for biomedical applications, the relatively low LCST may lack suitability for chemical processing applications such as selective separations and reusable/responsive catalysis. It could be suggested that a stronger emphasis should be placed on the development of a wider understanding of stimuli-responsive polymers, which in turn would facilitate the development of a broader range of responsive NPs, and progress their usefulness for a variety of applications. The aggregation of colloidal systems is often seen as a negative occurrence and as such is often overlooked or ignored in studies. Therefore, more detailed disclosure of the conditions under which responsive aggregation occurred would be a great aid to this research area. However, as shown in this review triggered aggregation is a useful behaviour that could be exploited in existing applications but also many future applications exist. One possible application is using in situ aggregation to cause targeted tissue embolization.
Conflicts of interest
There are no conflicts to declare.
Acknowledgements
We gratefully acknowledge financial support from the EPSRC (EP/R024839/1 and EP/S012265/1).
References
- A. R. Town, J. Taylor, K. Dawson, E. Niezabitowska, N. M. Elbaz, A. Corker, E. Garcia-Tuñón and T. O. McDonald, Tuning HIV drug release from a nanogel-based in situ forming implant by changing nanogel size, J. Mater. Chem. B, 2019, 7(3), 373–383 RSC.
- Y. Gao, A. Ahiabu and M. J. Serpe, Controlled Drug Release from the Aggregation–Disaggregation Behavior of pH-Responsive Microgels, ACS Appl. Mater. Interfaces, 2014, 6(16), 13749–13756 CrossRef CAS.
- A. R. Town, M. Giardiello, R. Gurjar, M. Siccardi, M. E. Briggs, R. Akhtar and T. O. McDonald, Dual-stimuli responsive injectable microgel/solid drug nanoparticle nanocomposites for release of poorly soluble drugs, Nanoscale, 2017, 9(19), 6302–6314 RSC.
- R. Mohsen, G. J. Vine, N. Majcen, B. D. Alexander and M. J. Snowden, Characterization of thermo and pH responsive NIPAM based microgels and their membrane blocking potential, Colloids Surf., A, 2013, 428, 53–59 CrossRef CAS.
- S. Chakraborty and C. L. Kitchens, Modifying Ligand Chemistry To Enhance Reusability of pH-Responsive Colloidal Gold Nanoparticle Catalyst, J. Phys. Chem. C, 2019, 123(43), 26450–26459 CrossRef CAS.
- T. Shu, Q. Shen, X. Zhang and M. J. Serpe, Stimuli-responsive polymer/nanomaterial hybrids for sensing applications, Analyst, 2020, 145(17), 5713–5724 RSC.
- J. Hu and S. Liu, Responsive Polymers for Detection and Sensing Applications: Current Status and Future Developments, Macromolecules, 2010, 43(20), 8315–8330 CrossRef CAS.
- R. J. Hall, V. T. Pinkrah, B. Z. Chowdhry and M. J. Snowden, Heteroaggregation studies of mixed cationic co-polymer/anionic homopolymer microgel dispersions, Colloids Surf., A, 2004, 233(1), 25–38 CrossRef CAS.
-
M. J. Snowden, B. Vincent and J. C. Morgan, Conformance control in underground reservoirs, GB2262117, 1993 Search PubMed.
- H. G. Schild, Poly(N-isopropylacrylamide): experiment, theory and application, Prog. Polym. Sci., 1992, 17(2), 163–249 CrossRef CAS.
- X. Fu, L. Hosta-Rigau, R. Chandrawati and J. W. Cui, Multi-Stimuli-Responsive Polymer Particles, Films, and Hydrogels for Drug Delivery, Chem, 2018, 4(9), 2084–2107 CAS.
- X. N. An, A. J. Zhu, H. H. Luo, H. T. Ke, H. B. Chen and Y. L. Zhao, Rational Design of Multi-Stimuli-Responsive Nanoparticles for Precise Cancer Therapy, ACS Nano, 2016, 10(6), 5947–5958 CrossRef CAS PubMed.
- M. L. Wei, Y. F. Gao, X. Li and M. J. Serpe, Stimuli-responsive polymers and their applications, Polym. Chem., 2017, 8(1), 127–143 RSC.
- R. G. Chaudhuri and S. Paria, Core/Shell Nanoparticles: Classes, Properties, Synthesis Mechanisms, Characterization, and Applications, Chem. Rev., 2012, 112(4), 2373–2433 CrossRef PubMed.
- B. Zdyrko and I. Luzinov, Polymer Brushes by the “Grafting to” Method, Macromol. Rapid Commun., 2011, 32(12), 859–869 CrossRef CAS PubMed.
- R. C. Advincula, Surface Initiated Polymerization from Nanoparticle Surfaces, J. Disper. Sci. Technol., 2003, 24(3/4), 343–361 CrossRef CAS.
- B. R. Saunders, N. Laajam, E. Daly, S. Teow, X. Hu and R. Stepto, Microgels: From responsive polymer colloids to biomaterials, Adv. Colloid Interface Sci., 2009, 147–148, 251–262 CrossRef CAS PubMed.
- R. Pelton, Temperature-sensitive aqueous microgels, Adv. Colloid Interface Sci., 2000, 85(1), 1–33 CrossRef CAS PubMed.
- P. Zarrintaj, M. Jouyandeh, M. R. Ganjali, B. S. Hadavand, M. Mozafari, S. S. Sheiko, M. Vatankhah-Varnoosfaderani, T. J. Gutiérrez and M. R. Saeb, Thermo-sensitive polymers in medicine: A review, Eur. Polym. J., 2019, 117, 402–423 CrossRef CAS.
- I. Dimitrov, B. Trzebicka, A. H. E. Müller, A. Dworak and C. B. Tsvetanov, Thermosensitive water-soluble copolymers with doubly responsive reversibly interacting entities, Prog. Polym. Sci., 2007, 32(11), 1275–1343 CrossRef CAS.
- S. Strandman and X. X. Zhu, Thermo-responsive block copolymers with multiple phase transition temperatures in aqueous solutions, Prog. Polym. Sci., 2015, 42, 154–176 CrossRef CAS.
- Y. Kotsuchibashi, Recent advances in multi-temperature-responsive polymeric materials, Polym. J., 2020, 52(7), 681–689 CrossRef CAS.
- G. Kocak, C. Tuncer and V. Bütün, pH-Responsive polymers, Polym. Chem., 2017, 8(1), 144–176 RSC.
- S. Dai, P. Ravi and K. C. Tam, pH-Responsive polymers: synthesis, properties and applications, Soft Matter, 2008, 4(3), 435–449 RSC.
- T. Xiang, T. Lu, W.-F. Zhao and C.-S. Zhao, Ionic-Strength Responsive Zwitterionic Copolymer Hydrogels with Tunable Swelling and Adsorption Behaviors, Langmuir, 2019, 35(5), 1146–1155 CrossRef CAS PubMed.
- O. Bertrand and J.-F. Gohy, Photo-responsive polymers: synthesis and applications, Polym. Chem., 2017, 8(1), 52–73 RSC.
- V. Marturano, P. Cerruti, M. Giamberini, B. Tylkowski and V. Ambrogi, Light-Responsive Polymer Micro- and Nano-Capsules, Polymers, 2017, 9(1), 19 Search PubMed.
- R. V. Ulijn, Enzyme-responsive materials: a new class of smart biomaterials, J. Mater. Chem., 2006, 16(23), 2217–2225 RSC.
-
M. Zelzer and R. V. Ulijn, Enzyme-responsive polymers: properties, synthesis and applications, in Smart Polymers and Their Applications, ed. M. R. Aguilar and J. SanRoman, Woodhead Publ Ltd, Cambridge, 2014, pp. 166–203 Search PubMed.
- J. Zhao, V. E. Lee, R. Liu and R. D. Priestley, Responsive Polymers as Smart Nanomaterials Enable Diverse Applications, Annu. Rev. Chem. Biomol. Eng., 2019, 10(1), 361–382 CrossRef CAS PubMed.
- X. Y. Zhang, L. Han, M. Y. Liu, K. Wang, L. Tao, Q. Wan and Y. Wei, Recent progress and advances in redox-responsive polymers as controlled delivery nanoplatforms, Mater. Chem. Front., 2017, 1(5), 807–822 RSC.
- M. Huo, J. Yuan, L. Tao and Y. Wei, Redox-responsive polymers for drug delivery: from molecular design to applications, Polym. Chem., 2014, 5(5), 1519–1528 RSC.
- E. A. Clark and J. E. G. Lipson, LCST and UCST behavior in polymer solutions and blends, Polymer, 2012, 53(2), 536–545 CrossRef CAS.
- Y. Kotsuchibashi, M. Ebara, T. Aoyagi and R. Narain, Recent Advances in Dual Temperature Responsive Block Copolymers and Their Potential as Biomedical Applications, Polymers, 2016, 8(11), 380 CrossRef PubMed.
- Y. Maeda, T. Nakamura and I. Ikeda, Hydration and Phase Behavior of Poly(N-vinylcaprolactam) and Poly(N-vinylpyrrolidone) in Water, Macromolecules, 2002, 35(1), 217–222 CrossRef CAS.
- Y. Maeda, IR Spectroscopic Study on the Hydration and the Phase Transition of Poly(vinyl methyl ether) in Water, Langmuir, 2001, 17(5), 1737–1742 CrossRef CAS.
- R. Hoogenboom, H. M. L. Thijs, M. J. H. C. Jochems, B. M. van Lankvelt, M. W. M. Fijten and U. S. Schubert, Tuning the LCST of poly(2-oxazoline)s by varying composition and molecular weight: alternatives to poly(N-isopropylacrylamide)?, Chem. Commun., 2008,(44), 5758–5760 RSC.
- J.-F. Lutz, Polymerization of oligo(ethylene glycol) (meth)acrylates: Toward new generations of smart biocompatible materials, J. Polym. Sci., Part A: Polym. Chem., 2008, 46(11), 3459–3470 CrossRef CAS.
- M. Constantin, M. Cristea, P. Ascenzi and G. Fundueanu, Lower critical
solution temperature versus volume phase transition temperature in thermoresponsive drug delivery systems, eXPRESS Polym. Lett., 2011, 5(10), 839–848 CrossRef CAS.
- K. Na, K. H. Lee and Y. H. Bae, pH-sensitivity and pH-dependent interior structural change of self-assembled hydrogel nanoparticles of pullulan acetate/oligo-sulfonamide conjugate, J. Controlled Release, 2004, 97(3), 513–525 CrossRef CAS.
- B. V. Derjaguin and L. D. Landau, Theory of the stability of strongly charged lyophobic sols and of the adhesion of strongly charged particles in solutions of electrolytes, Acta Physicochim. URSS, 1941, 14, 633 Search PubMed.
-
E. J. W. Verwey and J. T. G. Overbeek, Theory of the stability of lyophobic colloids, Elsevier Publishing Company, Inc., Amsterdam, 1948 Search PubMed.
- Y. Liang, N. Hilal, P. Langston and V. Starov, Interaction forces between colloidal particles in liquid: Theory and experiment, Adv. Colloid Interface Sci., 2007, 134–135, 151–166 CrossRef CAS PubMed.
- D. H. Napper and A. Netschey, Studies of the steric stabilization of colloidal particles, J. Colloid Interface Sci., 1971, 37(3), 528–535 CrossRef CAS.
- J. Brijitta and P. Schurtenberger, Responsive hydrogel colloids: Structure, interactions, phase behavior, and equilibrium and nonequilibrium transitions of microgel dispersions, Curr. Opin. Colloid Interface Sci., 2019, 40, 87–103 CrossRef CAS.
- A. Guillermo, J. P. Cohen Addad, J. P. Bazile, D. Duracher, A. Elaissari and C. Pichot, NMR investigations into heterogeneous structures of thermosensitive microgel particles, J. Polym. Sci., Part B: Polym. Phys., 2000, 38(6), 889–898 CrossRef CAS.
- S. Nöjd, P. Holmqvist, N. Boon, M. Obiols-Rabasa, P. S. Mohanty, R. Schweins and P. Schurtenberger, Deswelling behaviour of ionic microgel particles from low to ultra-high densities, Soft Matter, 2018, 14(20), 4150–4159 RSC.
- D. M. Heyes and A. C. Brańka, Interactions between microgel particles, Soft Matter, 2009, 5(14), 2681–2685 RSC.
- W. R. Schowalter, Stability and Coagulation of Colloids in Shear Fields, Annu. Rev. Fluid Mech., 1984, 16(1), 245–261 CrossRef.
- N. Bizmark and M. A. Ioannidis, Effects of Ionic Strength on the Colloidal Stability and Interfacial Assembly of Hydrophobic Ethyl Cellulose Nanoparticles, Langmuir, 2015, 31(34), 9282–9289 CrossRef CAS PubMed.
- K. A. Huynh and K. L. Chen, Aggregation Kinetics of Citrate and Polyvinylpyrrolidone Coated Silver Nanoparticles in Monovalent and Divalent Electrolyte Solutions, Environ. Sci. Technol., 2011, 45(13), 5564–5571 CrossRef CAS PubMed.
- X. Li, J. J. Lenhart and H. W. Walker, Aggregation Kinetics and Dissolution of Coated Silver Nanoparticles, Langmuir, 2012, 28(2), 1095–1104 CrossRef CAS PubMed.
- E. P. K. Currie, W. Norde and M. A. Cohen Stuart, Tethered polymer chains: surface chemistry and their impact on colloidal and surface properties, Adv. Colloid Interface Sci., 2003, 100–102, 205–265 CrossRef CAS PubMed.
- A. Lee, H. Y. Tsai and M. Z. Yates, Steric Stabilization of Thermally Responsive N-Isopropylacrylamide Particles by Poly(vinyl alcohol), Langmuir, 2010, 26(23), 18055–18060 CrossRef CAS PubMed.
- H. Du, R. Wickramasinghe and X. Qian, Effects of Salt on the Lower Critical Solution Temperature of Poly (N-Isopropylacrylamide), J. Phys. Chem. B, 2010, 114(49), 16594–16604 CrossRef CAS PubMed.
- Y. Zhang, S. Furyk, D. E. Bergbreiter and P. S. Cremer, Specific Ion Effects on the Water Solubility of Macromolecules: PNIPAM and the Hofmeister Series, J. Am. Chem. Soc., 2005, 127(41), 14505–14510 CrossRef CAS PubMed.
- J. Heyda and J. Dzubiella, Thermodynamic Description of Hofmeister Effects on the LCST of Thermosensitive Polymers, J. Phys. Chem. B, 2014, 118(37), 10979–10988 CrossRef CAS PubMed.
- D. Zámbó, G. Z. Radnóczi and A. Deák, Preparation of Compact Nanoparticle Clusters from Polyethylene Glycol-Coated Gold Nanoparticles by Fine-Tuning Colloidal Interactions, Langmuir, 2015, 31(9), 2662–2668 CrossRef PubMed.
- S. Saeki, N. Kuwahara, M. Nakata and M. Kaneko, Upper and lower critical solution temperatures in poly (ethylene glycol) solutions, Polymer, 1976, 17(8), 685–689 CrossRef CAS.
- M. Rasmusson and B. Vincent, Flocculation of microgel particles, React. Funct. Polym., 2004, 58(3), 203–211 CrossRef CAS.
- M. Rasmusson, A. Routh and B. Vincent, Flocculation of Microgel Particles with Sodium Chloride and Sodium Polystyrene Sulfonate as a Function of Temperature, Langmuir, 2004, 20(9), 3536–3542 CrossRef CAS PubMed.
- A. Town, E. Niezabitowska, J. Kavanagh, M. Barrow, V. R. Kearns, E. García-Tuñón and T. O. McDonald, Understanding the Phase and Morphological Behavior of Dispersions of Synergistic Dual-Stimuli-Responsive Poly(N-isopropylacrylamide) Nanogels, J. Phys. Chem. B, 2019, 123(29), 6303–6313 CrossRef CAS PubMed.
- M. Rasmusson and B. Vincent, Flocculation of microgel particles, React. Funct. Polym., 2004, 58(3), 203–211 CrossRef CAS.
- Z. Zhang, S. Maji, A. B. d. F. Antunes, D. Rycke, R. Zhang, Q. Hoogenboom, R. De Geest and B. G, Salt Plays a Pivotal Role in the Temperature-Responsive Aggregation and Layer-by-Layer Assembly of Polymer-Decorated Gold Nanoparticles, Chem. Mater., 2013, 25(21), 4297–4303 CrossRef CAS.
- S.-i. Yusa, K. Fukuda, T. Yamamoto, Y. Iwasaki, A. Watanabe, K. Akiyoshi and Y. Morishima, Salt Effect on the Heat-Induced Association Behavior of Gold Nanoparticles Coated with Poly(N-isopropylacrylamide) Prepared via Reversible Addition−Fragmentation Chain Transfer (RAFT) Radical Polymerization, Langmuir, 2007, 23(26), 12842–12848 CrossRef CAS PubMed.
- N. D. Samsuri, W. M. Mukhtar, A. R. Abdul Rashid, K. Ahmad Dasuki and A. A. R. H. Awangku Yussuf, Synthesis methods of gold nanoparticles for Localized Surface Plasmon Resonance (LSPR) sensor applications, EPJ Web Conf., 2017, 162, 01002 CrossRef.
- S. Liu, J. V. M. Weaver, Y. Tang, N. C. Billingham, S. P. Armes and K. Tribe, Synthesis of Shell Cross-Linked Micelles with pH-Responsive Cores Using ABC Triblock Copolymers, Macromolecules, 2002, 35(16), 6121–6131 CrossRef CAS.
- M. Zhang, L. Liu, H. Zhao, Y. Yang, G. Fu and B. He, Double-responsive polymer brushes on the surface of colloid particles, J. Colloid Interface Sci., 2006, 301(1), 85–91 CrossRef CAS PubMed.
- G. Liu, D. Wu, C. Ma, G. Zhang, H. Wang and S. Yang, Insight into the Origin of the Thermosensitivity of Poly[2-(dimethylamino)ethyl methacrylate], ChemPhysChem, 2007, 8(15), 2254–2259 CrossRef CAS PubMed.
- X. Han, X. Zhang, H. Zhu, Q. Yin, H. Liu and Y. Hu, Effect of Composition of PDMAEMA-b-PAA Block Copolymers on Their pH- and Temperature-Responsive Behaviors, Langmuir, 2013, 29(4), 1024–1034 CrossRef CAS PubMed.
- L. Hu, L.-Y. Chu, M. Yang, H.-D. Wang and C. H. Niu, Preparation and characterization of novel cationic pH-responsive poly(N,N′-dimethylamino ethyl methacrylate) microgels, J. Colloid Interface Sci., 2007, 311(1), 110–117 CrossRef CAS PubMed.
- Z. Dong, H. Wei, J. Mao, D. Wang, M. Yang, S. Bo and X. Ji, Synthesis and responsive behavior of poly(N,N-dimethylaminoethyl methacrylate) brushes grafted on silica nanoparticles and their quaternized derivatives, Polymer, 2012, 53(10), 2074–2084 CrossRef CAS.
- V. Bütün, S. P. Armes and N. C. Billingham, Synthesis and aqueous solution properties of near-monodisperse tertiary amine methacrylate homopolymers and diblock copolymers, Polymer, 2001, 42(14), 5993–6008 CrossRef.
- M. Mohammadi, M. Salami-Kalajahi, H. Roghani-Mamaqani and M. Golshan, Synthesis and investigation of dual pH- and temperature-responsive behaviour of poly[2-(dimethylamino)ethyl methacrylate]-grafted gold nanoparticles, Appl. Organomet. Chem., 2017, 31(9), e3702 CrossRef.
- A. A. Beltran-Osuna, J. L. Gomez-Ribelles and J. E. Perilla, Temperature and pH responsive behavior of antifouling zwitterionic mesoporous silica nanoparticles, J. Appl. Phys., 2020, 127(13), 11 CrossRef.
- E. C. Johnson, J. D. Willott, W. M. de Vos, E. J. Wanless and G. B. Webber, Interplay of Composition, pH, and Temperature on the Conformation of Multi-stimulus-responsive Copolymer Brushes: Comparison of Experiment and Theory, Langmuir, 2020, 36(21), 5765–5777 CrossRef CAS PubMed.
- R. Begum, Z. H. Farooqi and S. R. Khan, Poly(N-isopropylacrylamide-acrylic acid) copolymer microgels for various applications: A review, Int. J. Polym. Mater. Polym. Biomater., 2016, 65(16), 841–852 CrossRef CAS.
- V. Nigro, R. Angelini, B. Rosi, M. Bertoldo, E. Buratti, S. Casciardi, S. Sennato and B. Ruzicka, Study of network composition in interpenetrating polymer networks of poly(N isopropylacrylamide) microgels: The role of poly(acrylic acid), J. Colloid Interface Sci., 2019, 545, 210–219 CrossRef CAS PubMed.
- V. Nigro, R. Angelini, M. Bertoldo, V. Castelvetro, G. Ruocco and B. Ruzicka, Dynamic light scattering study of temperature and pH sensitive colloidal microgels, J. Non-Cryst. Solids, 2015, 407, 361–366 CrossRef CAS.
- P. Tian, Q. Wu and K. Lian, Preparation of temperature- and pH-sensitive, stimuli-responsive poly(N-isopropylacrylamide-co-methacrylic acid) nanoparticles, J. Appl. Polym. Sci., 2008, 108(4), 2226–2232 CrossRef CAS.
- J. B. Li, W. L. Wu, C. Han, S. J. Zhang, H. Y. Zhou and J. W. Guo, Aggregation behavior of pH- and thermo-responsive block copolymer protected gold nanoparticles, Colloid Polym. Sci., 2014, 292(7), 1657–1664 CrossRef CAS.
- H. Naeem, Z. H. Farooqi, L. A. Shah and M. Siddiq, Synthesis and characterization of p(NIPAM-AA-AAm) microgels for tuning of optical Properties of silver nanoparticles, J. Polym. Res., 2012, 19(9), 10 Search PubMed.
- N. Al-Manasir, K. Zhu, A.-L. Kjøniksen, K. D. Knudsen, G. Karlsson and B. Nyström, Effects of Temperature and pH on the Contraction and Aggregation of Microgels in Aqueous Suspensions, J. Phys. Chem. B, 2009, 113(32), 11115–11123 CrossRef CAS.
- L. Song, H. Sun, X. Chen, X. Han and H. Liu, From multi-responsive tri- and diblock copolymers to diblock-copolymer-decorated gold nanoparticles: the effect of architecture on micellization behaviors in aqueous solutions, Soft Matter, 2015, 11(24), 4830–4839 RSC.
- X. Chen, H. Sun, J. Xu, X. Han, H. Liu and Y. Hu, pH-modulated double LCST behaviors with diverse aggregation processes of random-copolymer grafted silica nanoparticles in aqueous solution, RSC Adv., 2015, 5(105), 86584–86592 RSC.
- S. F. Medeiros, P. F. M. Oliveira, T. M. Silva, B. R. Lara, A. Elaissari and A. M. Santos, Biocompatible and multi-responsive poly(N-vinylcaprolactam)-based microgels: The role of acidic comonomers in the colloidal properties and phase transition as a function of temperature and pH, Eur. Polym. J., 2015, 73, 191–201 CrossRef CAS.
- Y. Shi, V. Selin, Y. Wang and S. A. Sukhishvili, Multiresponsive Block Copolymer-Modified “Hairy” Gold Nanoparticles for Remote Control of Interfaces, Part. Part. Syst. Charact., 2013, 30(11), 950–957 CrossRef CAS.
- K. Chen, L. Cao, Y. Zhang, K. Li, X. Qin and X. Guo, Conformation Study of Dual Stimuli-Responsive Core-Shell Diblock Polymer Brushes, Polymers, 2018, 10(10), 1084 CrossRef PubMed.
- H. Y. Cao, F. H. Guo, Z. Y. Chen and X. Z. Kong, Preparation of Thermoresponsive Polymer Nanogels of Oligo(Ethylene Glycol) Diacrylate-Methacrylic Acid and Their Property Characterization, Nanoscale Res. Lett., 2018, 13, 10 CrossRef.
- J. Shan, J. Chen, M. Nuopponen and H. Tenhu, Two Phase Transitions of Poly(N-isopropylacrylamide) Brushes Bound to Gold Nanoparticles, Langmuir, 2004, 20(11), 4671–4676 CrossRef CAS PubMed.
- Z. H. Farooqi, H. U. Khan, S. M. Shah and M. Siddiq, Stability of poly(N-isopropylacrylamide-co-acrylic acid) polymer microgels under various conditions of temperature, pH and salt concentration, Arabian J. Chem., 2017, 10(3), 329–335 CrossRef CAS.
- J. P. Pinheiro, L. Moura, R. Fokkink and J. P. S. Farinha, Preparation and Characterization of Low Dispersity Anionic Multiresponsive Core–Shell Polymer Nanoparticles, Langmuir, 2012, 28(13), 5802–5809 CrossRef CAS PubMed.
- M. Ballauff and Y. Lu, “Smart” nanoparticles: Preparation, characterization and applications, Polymer, 2007, 48(7), 1815–1823 CrossRef CAS.
- J. Oberdisse and T. Hellweg, Recent advances in stimuli-responsive core-shell microgel particles: synthesis, characterisation, and applications, Colloid Polym. Sci., 2020, 298(7), 921–935 CrossRef CAS.
- T. Hoare and R. Pelton, Titrametric Characterization of pH-Induced Phase Transitions in Functionalized Microgels, Langmuir, 2006, 22(17), 7342–7350 CrossRef CAS PubMed.
- T. Hoare and R. Pelton, Dimensionless plot analysis: A new way to analyze functionalized microgels, J. Colloid Interface Sci., 2006, 303(1), 109–116 CrossRef CAS PubMed.
- J. R. S. Martin, I. Bihannic, C. Santos, J. P. S. Farinha, B. Demé, F. A. M. Leermakers, J. P. Pinheiro, E. Rotureau and J. F. L. Duval, Structure of Multiresponsive Brush-Decorated Nanoparticles: A Combined Electrokinetic, DLS, and SANS Study, Langmuir, 2015, 31(16), 4779–4790 CrossRef CAS PubMed.
- B. Li, X. S. Jiang and J. Yin, Multi-responsive microgel of hyperbranched poly(ether amine) (hPEA-mGel) for the selective adsorption and separation of hydrophilic fluorescein dyes, J. Mater. Chem., 2012, 22(34), 17976–17983 RSC.
- R. Wang, B. Yu, X. Jiang and J. Yin, Understanding the Host–Guest Interaction Between Responsive Core-Crosslinked Hybrid Nanoparticles of Hyperbranched Poly(ether amine) and Dyes: The Selective Adsorption and Smart Separation of Dyes in Water, Adv. Funct. Mater., 2012, 22(12), 2606–2616 CrossRef CAS.
- C. Di, X. Jiang, R. Wang and J. Yin, Multi-responsive polymer nanoparticles from the amphiphilic poly(dimethylsiloxane) (PDMS)-containing poly(ether amine) (PDMS-gPEA) and its potential application for smart separation, J. Mater. Chem., 2011, 21(12), 4416–4423 RSC.
- X. S. Jiang, C. F. Di, B. Yu and J. Yin, Amphiphilic Zwitterionic Poly(dimethylsiloxane) (PDMS)-Contained Poly(Ether amine) (Z-SiPEA) as the Responsive Polymeric Dispersant, ACS Appl. Mater. Interfaces, 2011, 3(5), 1749–1756 CrossRef CAS PubMed.
- S. J. Deng, R. Wang, H. J. Xu, X. S. Jiang and J. Yin, Hybrid hydrogels of hyperbranched poly(ether amine)s (hPEAs) for selective adsorption of guest molecules and separation of dyes, J. Mater. Chem., 2012, 22(19), 10055–10061 RSC.
- L. Tian, L. Lu, Y. Qiao, S. Ravi, F. Salatan and M. P. Melancon, Stimuli-Responsive Gold Nanoparticles for Cancer Diagnosis and Therapy, J. Funct. Biomater., 2016, 7(3), 19 CrossRef.
- W. Yu, M. Shevtsov, X. Chen and H. Gao, Advances in aggregatable nanoparticles for tumor-targeted drug delivery, Chin. Chem. Lett., 2020, 31(6), 1366–1374 CrossRef CAS.
- S. Hajebi, N. Rabiee, M. Bagherzadeh, S. Ahmadi, M. Rabiee, H. Roghani-Mamaqani, M. Tahriri, L. Tayebi and M. R. Hamblin, Stimulus-responsive polymeric nanogels as smart drug delivery systems, Acta Biomater., 2019, 92, 1–18 CrossRef CAS PubMed.
- X. Jin, Q. Wang, J. Sun, H. Panezail, X. Wu and S. Bai, Dual temperature- and pH-responsive ibuprofen delivery from poly(N-isopropylacrylamide-co-acrylic acid) nanoparticles and their fractal features, Polym. Bull., 2017, 74(9), 3619–3638 CrossRef CAS.
- K. H. Bae, L.-S. Wang and M. Kurisawa, Injectable biodegradable hydrogels: progress and challenges, J. Mater. Chem. B, 2013, 1(40), 5371–5388 RSC.
- M. S. Riederer, B. D. Requist, K. A. Payne, J. D. Way and M. D. Krebs, Injectable and microporous scaffold of densely-packed, growth factor-encapsulating chitosan microgels, Carbohydr. Polym., 2016, 152, 792–801 CrossRef CAS PubMed.
- C. B. Packhaeuser, J. Schnieders, C. G. Oster and T. Kissel, In situ forming parenteral drug delivery systems: an overview, Eur. J. Pharm. Biopharm., 2004, 58(2), 445–455 CrossRef CAS PubMed.
- A. L. Kjøniksen, M. T. Calejo, K. Zhu, A. M. S. Cardoso, M. C. P. de Lima, A. S. Jurado, B. Nyström and S. A. Sande, Sustained Release of Naltrexone from Poly(N–Isopropylacrylamide) Microgels, J. Pharm. Sci., 2014, 103(1), 227–234 CrossRef PubMed.
- R. Mohsen, G. J. Vine, N. Majcen, B. D. Alexander and M. J. Snowden, Characterization of thermo and pH responsive NIPAM based microgels and their membrane blocking potential, Colloids Surf., A, 2013, 428, 53–59 CrossRef CAS.
- M. S. Kamal, A. A. Adewunmi, A. S. Sultan, M. F. Al-Hamad and U. Mehmood, Recent Advances in Nanoparticles Enhanced Oil Recovery: Rheology, Interfacial Tension, Oil Recovery, and Wettability Alteration, J. Nanomater., 2017, 2017, 2473175 Search PubMed.
- M. B. Lopes, M. A. Sinhoreti, A. Gonini Júnior, S. Consani and J. F. McCabe, Comparative study of tubular diameter and quantity for human and bovine dentin at different depths, Braz. Dent. J., 2009, 20(4), 279–283 CrossRef PubMed.
- S. Kurzhals, R. Zirbs and E. Reimhult, Synthesis and Magneto-Thermal Actuation of Iron Oxide Core–PNIPAM Shell Nanoparticles, ACS Appl. Mater. Interfaces, 2015, 7(34), 19342–19352 CrossRef CAS PubMed.
- M. A. Nash, J. N. Waitumbi, A. S. Hoffman, P. Yager and P. S. Stayton, Multiplexed Enrichment and Detection of Malarial Biomarkers Using a Stimuli-Responsive Iron Oxide and Gold Nanoparticle Reagent System, ACS Nano, 2012, 6(8), 6776–6785 CrossRef CAS PubMed.
- A. S. Paulus, R. Heinzler, H. W. Ooi and M. Franzreb, Temperature-Switchable Agglomeration of Magnetic Particles Designed for Continuous Separation Processes in Biotechnology, ACS Appl. Mater. Interfaces, 2015, 7(26), 14279–14287 CrossRef CAS PubMed.
- S. Li, Y. Wu, J. Wang, Q. Zhang, Y. Kou and S. Zhang, Double-responsive polyampholyte as a nanoparticle stabilizer: application to reversible dispersion of gold nanoparticles, J. Mater. Chem., 2010, 20(21), 4379–4384 RSC.
- J. L. Zhang, M. X. Zhang, K. J. Tang, F. Verpoort and T. L. Sun, Polymer-Based Stimuli-Responsive Recyclable Catalytic Systems for Organic Synthesis, Small, 2014, 10(1), 32–46 CrossRef CAS PubMed.
- D. Li, N. Liu, Y. Gao, W. Lin and C. Li, Thermosensitive polymer stabilized core-shell AuNR@Ag nanostructures as “smart” recyclable catalyst, J. Nanopart. Res., 2017, 19(11), 377 CrossRef.
- S. R. Khan, Z. H. Farooqi, M. Ajmal, M. Siddiq and A. Khan, Synthesis, Characterization, and Silver Nanoparticles Fabrication in N-isopropylacrylamide-Based Polymer Microgels for Rapid Degradation of p-Nitrophenol, J. Dispersion Sci. Technol., 2013, 34(10), 1324–1333 CrossRef CAS.
- Y. Tang, T. Wu, B. Hu, Q. Yang, L. Liu, B. Yu, Y. Ding and S. Ye, Synthesis of thermo- and pH-responsive Ag nanoparticle-embedded hybrid microgels and their catalytic activity in methylene blue reduction, Mater. Chem. Phys., 2015, 149–150, 460–466 CrossRef CAS.
- M. Zelzer, S. J. Todd, A. R. Hirst, T. O. McDonald and R. V. Ulijn, Enzyme responsive materials: design strategies and future developments, Biomater. Sci., 2013, 1(1), 11–39 RSC.
- A. Laromaine, L. L. Koh, M. Murugesan, R. V. Ulijn and M. M. Stevens, Protease-triggered dispersion of nanoparticle assemblies, J. Am. Chem. Soc., 2007, 129(14), 4156–4157 CrossRef CAS PubMed.
- R. Chapman, Y. Y. Lin, M. Burnapp, A. Bentham, D. Hillier, A. Zabron, S. Khan, M. Tyreman and M. M. Stevens, Multivalent Nanoparticle Networks Enable Point-of-Care Detection of Human Phospholipase-A2 in Serum, ACS Nano, 2015, 9(3), 2565–2573 CrossRef CAS PubMed.
- J. C. Gaulding, M. H. Smith, J. S. Hyatt, A. Fernandez-Nieves and L. A. Lyon, Reversible Inter- and Intra-Microgel Cross-Linking Using Disulfides, Macromolecules, 2012, 45(1), 39–45 CrossRef CAS PubMed.
- B. Yu, N. Song, H. Hu, G. H. Chen, Y. Q. Shen and H. L. Cong, A degradable triple temperature-, pH-, and redox-responsive drug system for cancer chemotherapy, J. Biomed. Mater. Res., Part A, 2018, 106(12), 3203–3210 CrossRef CAS PubMed.
- M. Mackiewicz, J. Romanski, E. Drozd, B. Gruber-Bzura, P. Fiedor, Z. Stojek and M. Karbarz, Nanohydrogel with N,N ‘-bis(acryloyl)cystine crosslinker loading for high drug, Int. J. Pharm., 2017, 523(1), 336–342 CrossRef CAS PubMed.
- X. J. Zhang, S. Malhotra, M. Molina and R. Haag, Micro- and nanogels with labile crosslinks - from synthesis to biomedical applications, Chem. Soc. Rev., 2015, 44(7), 1948–1973 RSC.
- D. J. Siegwart, S. A. Bencherif, A. Srinivasan, J. O. Hollinger and K. Matyjaszewski, Synthesis, characterization, and in vitro cell culture viability of degradable poly(N-isopropylacrylamide-co-5,6-benzo-2-methylene-1,3-dioxepane)-based polymers and crosslinked gels, J. Biomed. Mater. Res., Part A, 2008, 87A(2), 345–358 CrossRef CAS PubMed.
- A. Galperin, T. J. Long and B. D. Ratner, Degradable, Thermo-Sensitive Poly(N-isopropyl acrylamide)-Based Scaffolds with Controlled Porosity for Tissue Engineering Applications, Biomacromolecules, 2010, 11(10), 2583–2592 CrossRef CAS PubMed.
- S. Y. Lu, M. Z. Liu and B. L. Ni, Degradable, injectable poly(N-isopropylacrylamide)-based hydrogels with low gelation concentrations for protein delivery application, Chem. Eng. J., 2011, 173(1), 241–250 CrossRef.
|
This journal is © The Royal Society of Chemistry 2021 |