DOI:
10.1039/D0NR06920E
(Paper)
Nanoscale, 2021,
13, 100-107
Extended gate-type organic transistor functionalized by molecularly imprinted polymer for taurine detection†
Received
27th September 2020
, Accepted 2nd November 2020
First published on 2nd November 2020
Abstract
Molecularly imprinted polymers (MIPs) are a fascinating technology for the sensitive and selective detection of target molecules. However, in most situations, the need for complicated and expensive analytical devices for reading the responses of MIPs greatly limits their applications. For exploring low-cost and easy-to-use applications of MIPs, herein we have developed a MIP-modified extended-gate type organic field-effect transistor (MIP-OFET). Taurine was selected as a demonstrative analyte due to its biological roles and utility as a nutrient. We explored the rational design of the novel MIP with the aid of density functional theory and wave function calculations and characterized the electrochemically synthesized MIP using differential pulse voltammetry and electrochemical impedance spectroscopy. The mechanism of taurine detection by the MIP-OFET can be explained by the changes in the surface potential of the MIP-functionalized extended-gate electrode accompanied with the capture of taurine. The detection limit of taurine in complete aqueous media was estimated to be 0.33 μM, which was lower or comparable to those calculated by high-performance liquid chromatography. Furthermore, taurine in a commercial drink without any extraction was also successfully detected using the fabricated MIP-OFET. This study would broaden the scope of the applications of MIP-OFETS as chemical sensors for on-site detection of various daily nutrients.
Introduction
Organic field-effect transistors (OFETs) are one of the most promising candidates for sensor devices owing to their light weight, compact size, low-cost processability, mechanical flexibility, and eco-friendly manufacturing process.1 The OFETs have the potential to meet the increasing demands for improving the quality of our daily lives through environmental and physical assessments. In this regard, we have developed extended gate-type OFETs functionalized with artificial receptors as chemical sensor devices.2 The OFETs allow the detection of cations,3 anions,4 nonions,5 and proteins6 under environmental or biological conditions without labeling the analytes. In comparison to biomaterial-attached chemical sensors,7 the OFETs functionalized with artificial receptors can be employed under broader conditions and situations due to the chemical and thermal stabilities of the receptors. Furthermore, the time of analyses of the OFETs could be shorter as compared to the conventional bioanalytical methods such as enzyme-linked immunosorbent assay (ELISA).6 Thus, artificial receptors can potentially accelerate the practical applications of the OFET-based chemical sensor devices. However, the functionalization by the artificial receptors occasionally results in the OFET responses to the analytes besides the target ones.2a
To enhance the selectivity of the OFET device, we have focused on molecularly imprinted polymers (MIPs)8 for the molecular recognition sites on the extended gate. The MIPs are formed by simple polymerization of monomers in the presence of the target analytes as templates. After the removal of the templates, the structures of the MIPs have the specific cavities for the targets. Depending on the types of the polymer materials, a wide range of analytes from small molecules to biomacromolecules can be captured.8e,9 In addition, the MIPs can be easily attached to various types of substrates including gold, carbon materials, silicon and nanoparticles.10 Thus, MIPs have been employed for various sensor devices such as quartz crystal microbalance chips,11 surface plasmon resonance,12 and electrochemical devices.8e,11a,13 In this context, an OFET functionalized with MIP was previously reported for gas sensing;13 however, chemical sensing of aqueous analytes has not been achieved by the MIP-functionalized OFET. This is because, in general, OFET devices are highly vulnerable to humidity, and thus it is difficult to employ the typical approaches for chemical sensing in aqueous media. Besides, it is crucial to investigate whether the sensors can be repeatedly used for analyses.
To overcome these challenges, we have integrated the extended gate-type OFETs and MIP (MIP-OFET) as a new class of chemical sensor devices (Fig. 1). The detection part (i.e., extended gate) is separated from the drive part (i.e., OFET).2 Thus, the MIP-OFET only needs the immersion of the extended gate into an analyte solution, which prevents device degradation due to water exposure. With this in mind, taurine (Tau) was selected as a representative aqueous analyte because of its important roles in the functioning of vital organs including the liver,14 retina,15 muscles,16 and brain.17 We first carried out density functional theory (DFT) calculations to gain access to the rational molecular design for the selective detection of Tau by the MIP-OFET. The MIP-OFET was successfully prepared and subjected to the functional study of chemical sensing in aqueous media. Most importantly, we successfully achieved the qualitative, quantitative, selective and repeatable detections of Tau. We demonstrate herein that the MIP-OFET is an effective strategy to overcome the potential drawback of the OFET toward chemical sensing, and would be promising candidates as platforms for the analyses of aqueous analytes.
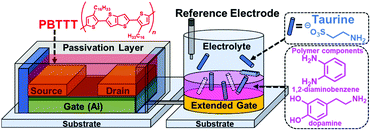 |
| Fig. 1 Extended gate-type OFET functionalized with MIP for taurine detection. | |
Experimental section
Density functional theory (DFT) calculation for the MIP material
The DFT calculation was carried out using Gaussian 16 Revision C.01.18 B3LYP-D3(BJ)/6-311+G* with the PCM solvent model (i.e., water was used as a solvent) was used for the optimization of the molecular complex. The electronic energy of the molecular complex was calculated using M06-2X-D3(0)/ma-def2-TZVPP with the PCM solvent model using water as a solvent. The M06-2X-D3(0)/ma-def2-TZVPP combined with a counterpoise (CP) method was used to calculate the energy of the Basis Set Superposition Error. The wave function was calculated using Multiwfn 3.7. The isosurface figure of the Independent Gradient Model (IGM)19 was calculated with Multiwfn 3.7 and visualized with Visual Molecular Dynamics (VMD) 1.9.4. See more details in the ESI† for the calculation.
Functionalization of the extended-gate electrode by MIP
The extended-gate electrode (thickness: 70 nm, sensing area: 15 mm2) made of gold (Au) was deposited on a polyethylene naphthalate film (125 μm in thickness) by thermal evaporation. The electrode was washed with Milli-Q water and methanol, dried with nitrogen gas flow, and cleaned by UV-O3 treatment for 10 minutes. Subsequently, a MIP thin film was prepared on the surface of the extended gate by cyclic voltammetry (CV) at a scan rate of 20 mV s−1 in a phosphate buffered solution (PBS) at pH 7.4 (20 mM) containing 3-hydroxytyramine hydrochloride (6 mM), 1,2-diaminobenzene (2 mM), and taurine (2 mM). After the polymerization, the template was removed from the electrode by repeatedly rinsing with HClaq. (50 mM) and deionized water to obtain the MIP-functionalized electrode. For comparison, the non-imprinted polymer (NIP) electrode was also prepared by the same procedure but without Tau.
Electrochemical characterization of functionalized electrodes
A three-electrode system was applied for differential pulse voltammetry (DPV) and electrochemical impedance spectroscopy (EIS). A bare Au electrode, the MIP-covered Au electrode, and a NIP-covered Au electrode were used as working electrodes. An Ag/AgCl electrode (with 3 M NaCl) and a Pt wire electrode were used as the reference electrode and the counter electrode, respectively. For the background measurements of DPV, a PBS (50 mM) of pH 7.4 containing K3FeCN6 (50 mM) and KCl (100 mM) was used as the background solution. The potential range was set to 0.35–0.16 V with a scanning rate of 4 mV s−1 and an amplitude of 50 mV. For the background measurements of EIS, a mixture of K3FeCN6 (10 mM) and KCl (100 mM) was used. The potential was set at 175 mV (vs. Ag/AgCl) and the frequency range was set as 3 MHz to 0.1 Hz with an amplitude potential of 5 mV.
Fabrication and characterization of the OFET
The OFET was operated at a low drive voltage (<|3| V) by employing aluminum oxide (AlOx) with tetradecylphosphonic acid (TDPA) as a gate dielectric layer.20 An easy fabrication was enabled using solution-processable poly{2,5-bis(3-hexadecylthiophene-2-yl)thieno[3,2-b]thiophene} (PBTTT) as the active layer.21 To obtain a stable operation, the active layer was passivated with a fluoropolymer (CYTOP™). More details of reagents, instruments, and the fabrication process of the OFET are summarized in the ESI.† The electrical characteristics of the fabricated OFET device were measured using a source meter, where sweep voltage was applied to the drain electrode. The source electrode was connected to the ground, and the gate voltage was applied through a combination of the extended-gate and Ag/AgCl reference electrodes immersed in PBS buffer (10 mM) at pH 7.4. To measure the transfer characteristics, a constant drain voltage (VDS = −1 V) was applied to the drain electrode, and the sweep voltage (VGS) was applied to the gate electrode from 0.5 V to −3 V. The measurement was repeated for three times. In the case of the output characteristics, VDS was swept from 0 V to −3 V, while VGS was set from 0 V to −3 V at −1 V steps.
Taurine detection by the extended gate-type OFET functionalized with MIP
Various concentrations of taurine (1–10 μM) were added into the PBS (10 mM) at pH 7.4 with KCl (50 mM). The transfer characteristics were measured 10 min after the addition of Tau. The threshold voltage (VTH) was calculated from the obtained transfer characteristics.1c For the selectivity test, the 10 μM analytes (L-alanine (Ala), L-arginine (Arg), L-aspartic acid (Asp), L-cysteine (Cys), L-glutamic acid (Glu), malonic acid (Mal) or Tau) each were added into the buffer solution. To investigate the repeatability of the OFET device, the MIP-functionalized extended-gate was rinsed thoroughly with HClaq. (50 mM) and deionized water after each measurement. Then, the transfer characteristics were measured using the same electrode. The reusability test was repeated for five times. For the real sample analysis, 0.05 mL of a commercially available drink Lipovitan (Taisho Pharmaceutical Co. Ltd,) containing Tau (79.9 mM) was mixed with 19.95 mL of the buffer solution and 0.20 mL of the diluted solution was further mixed with 19.80 mL of the PBS. The final mixture was used for measuring the transfer characteristics of the MIP-OFET.
Results and discussion
DFT and wave function calculation of intermolecular interactions between monomers and taurine
One critical factor for the successful synthesis of MIPs is the strong intermolecular interactions between the monomers and templates. To evaluate the strength of the interactions, the binding energy (BE) of the complex composed of monomers and templates can be calculated as BE = E(complex) − E(monomers) − E(templates). DFT calculations were carried out to calculate all the electronic properties. Before the experimental polymerization, the complexes among Tau, dopamine and 1,2-diaminobenzene in the molar ratios of 1
:
1
:
1 to 1
:
4
:
1 were optimized by B3LYP-D3(BJ)/6-311+G*
22 and calculated by M06-2X-D3(0)/ma-def2-TZVPP with the PCM solvent model.23 The M06-2X with Grimme DFT-D3(0) correlation exhibits excellent performance in comprehending the energy of the intermolecular noncovalent interactions (NCIs) between the organic molecules.24 The calculated BE values are listed in Table S1.† The energy values of complexes with the molar ratios (dopamine
:
1,2-diaminobenzene
:
taurine) 3
:
1
:
1 and 4
:
1
:
1 were very close and greatly higher than those with the molar ratios 1
:
1
:
1 and 2
:
1
:
1. To calculate more precise BE values for comparison, the Basis Set Superposition Error (BSSE) was taken into account. The BSSE was formed owing to the overlap of the basis function of single molecules in the complex, leading to the overestimation of the BE values. In addition, the CP method was adopted to decrease the effect of BSSE. After the CP correlation, the 3
:
1
:
1 molar ratio showed the lowest BE value (−48.1 kcal mol−1). The optimized structure is shown in Fig. S1.† To further visualize the NCIs sophisticatedly, the isosurface IGM figure was obtained by the wave function analysis of the optimized complex using Multiwfn25 and presented in VMD26 (Fig. 2). The green areas indicate weak NCIs, while the blue areas indicate strong NCIs. From the IGM isosurface figure, the hydrogen bonds between Tau and the monomers were precisely displayed. From these data calculated by the DFT and wave function analysis, we evaluated the NCIs of the complexes, and subsequently, selected dopamine
:
1,2-diaminobenzene
:
Tau ratio = 3
:
1
:
1 as ideal for the MIP synthesis.
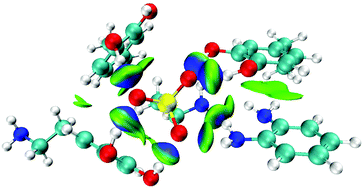 |
| Fig. 2 The isosurface IGM figure of the optimized complex with dopamine, 1,2-diaminobenzene, and Tau. The molar ratio equals to 3 : 1 : 1. The green and blue areas indicate the weak and strong NCIs, respectively. | |
Electrochemical polymerization of MIP/NIP modified electrodes and characterization of MIP modified electrodes
The electrochemical polymerization of MIP was operated by CV in a 3-electrode cell (Fig. 3). Above 0.1 V vs. Ag/AgCl, the monomers together with Tau polymerized on the surface of the Au electrode. As the cycles were repeated for electrochemical polymerization, the peak current gradually decreased due to the limited accessibility of the monomer to the electrode surface. After 10 cycles, the observed peak current was scarce because the electrode surface was almost completely covered with the MIP. The extraction of Tau by repeated washing (details in the Experimental section) afforded the MIP-functionalized electrode. The electrochemical polymerization of NIP was also operated under the same conditions but without Tau, which showed similar CV (Fig. S2†). The formation of the MIP and NIP was also supported by an electrochemical quartz crystal microbalance that measured the increase in the mass of the electrodes (see the ESI†).27
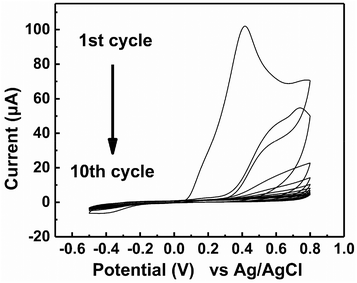 |
| Fig. 3 Cyclic voltammograms obtained during the formation of an MIP film on the surface of Au electrode in the 20 mM PBS (pH 7.4) containing 6 mM dopamine, 2 mM 1,2-diaminobenzene and 2 mM Tau. | |
Characterization of MIP modified electrodes
EIS and DPV measurements were carried out to characterize the functionalized electrodes and to investigate their potential sensing ability. Fig. 4 shows the changes in the Nyquist plots measured at different steps in a 10 mM K3Fe(CN)6 solution. In the Nyquist plot, the diameter of the semicircle corresponds to the charge-transfer resistance (i.e., the Rct value). The bare Au electrode exhibited the lowest resistance (Fig. 4, black line) and the small diameter implied almost no charge-transfer resistance on the bare electrode. The Rct value of the NIP electrode (Fig. 4, blue line) was obviously larger than those of other electrodes and was almost unchanged before and after the extraction treatment. Meanwhile, the Rct value of the MIP electrode after the extraction (Fig. 4, red line) was significantly decreased compared to that before the extraction (Fig. 4, green line), implying the formation of the imprinted cavities. In addition, after incubation in a Tau solution (100 μM), the Rct value of the MIP electrode increased again (Fig. 4, purple line), indicating the Tau was rebound to the imprinted cavities.8e Furthermore, DPV was also utilized to monitor the formation of the MIP electrode and to investigate its adsorption capacity (Fig. 5a). The DPV curve of the bare electrode in 10 mM K3Fe(CN)6 solution showed clearly-defined redox peak (Fig. 5a, blue solid line), which greatly decreased after the electrochemical polymerization for the MIP or NIP film on the electrode surface (Fig. 5a, red and black solid lines). This phenomenon implied that the polymer film exhibits low conductivity, which prevented the diffusion of the electroactive ion [Fe(CN)6]3− to the surface of the Au electrode. The redox peak of the MIP film obviously increased after the extraction of the Tau templates from the MIP film (Fig. 5a, red dashed line), indicating that the imprinted cavities were generated in the MIP film. These cavities could allow more [Fe(CN)6]3− to approach the Au electrode surface. On the other hand, the redox peak of the NIP was unaffected by the extraction treatment (Fig. 5a, dashed black line). After the incubation of the extracted MIP electrode with various concentrations of Tau, the redox peaks decreased step-by-step and showed a linear response (Fig. 5c). The lower and upper limits of detection for Tau were 100 μM and 1000 μM, respectively. The responses suggest that the cavities rebound with Tau molecules via noncovalent interactions, which prevented the [Fe(CN)6]3− to approach the surface of the Au electrode.28 Thus, the combination of DPV and EIS measurements proved that the electrochemical responses differ between the MIP and the NIP and that the responses of the MIP film are affected by Tau in the imprinted cavities.
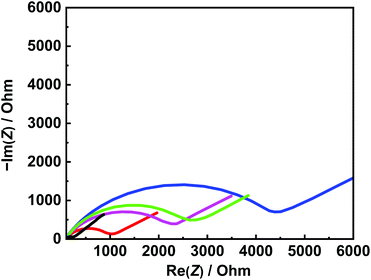 |
| Fig. 4 Nyquist plots for black line: the bare Au electrode, blue line: the NIP electrode, green line: the MIP electrode before removal of Tau, red line: the MIP electrode after removal of Tau, and purple line: the MIP electrode after incubation with 100 μM Tau. Applied potential: 175 mV vs. Ag/AgCl. Frequency range: 3 MHz to 0.1 Hz. Amplitude potential: 5 mV. | |
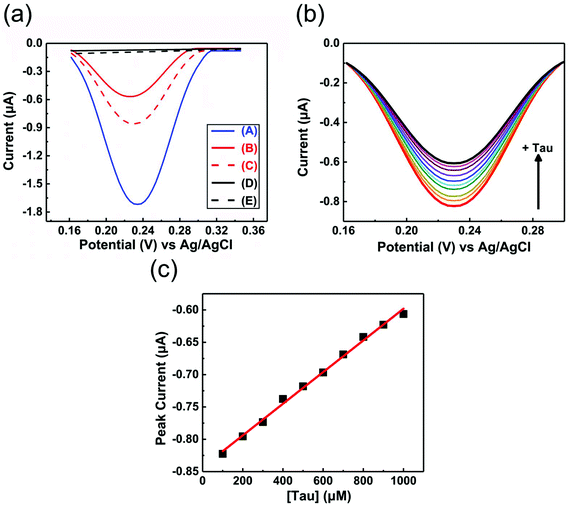 |
| Fig. 5 (a) DPV of A: the bare Au electrode, B: the MIP without Tau extraction, C: the MIP after Tau extraction, D: NIP without Tau extraction, and E: the NIP after Tau extraction. (b) DPV of the MIP after Tau extraction (B) in the presence of 100–1000 μM Tau in the background solution. (c) Plot of the peak current as a function of [Tau] (100–1000 μM). | |
To gain more evidence of the formation of MIP on the electrode, the surface morphologies of the electrodes were observed by atomic force microscopy (AFM) (Fig. 6). The smooth surface of the bare Au showed a low roughness with a root mean square deviation (Rq) of 2.7 nm (Fig. 6a). The Rq value (6.0 nm) of the NIP film was higher due to the protuberance probably formed by the copolymerization of dopamine and 1,2-diaminobenzene without Tau (Fig. 6b). The surface morphology of the MIP film (Rq = 3.8 nm) was smoother than that of the NIP film (Fig. 6c). More importantly, the roughness of MIP was obviously increased after the extraction of the MIP film (Rq = 4.6 nm) (Fig. 6d), indicating the formation of pore structures after the removal of Tau from the MIP film.29
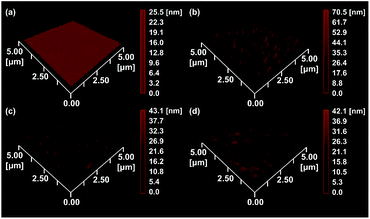 |
| Fig. 6 AFM images of (a) the bare Au electrode, (b) the NIP-covered Au electrode, (c) the MIP-covered Au electrode without Tau extraction, and (d) the MIP-covered Au electrode after Tau extraction. | |
Taurine detection by the OFET with the MIP-functionalized extended gate electrode
The basic characteristics of the fabricated OFET were initially measured, showing the stable operation at low drive voltages (<|3| V) (Fig. S4†). The successful characterization of the fabricated OFET and the electrochemical response of the MIP to Tau motivated us to detect Tau by the MIP-OFET. The transfer characteristics of MIP-OFET were measured by adding Tau in a PBS (10 mM) with KCl (50 mM) at pH 7.4. The negative shift of the transfer characteristics was observed by the addition of Tau in a concentration-dependent manner, suggesting the encapsulation of Tau in the MIP cavities. At physiological pH, Tau has an anionic form30 which would mainly affect the surface potential on the extended gate of the MIP-OFET. The potential shift would subsequently affect the channel conductance of MIP-OFET, which was closely related to the VTH value.31 Thus, the VTH value was shifted upon the addition of Tau as shown in the titration isotherm (Fig. 7). The limit of detection (LoD) was calculated as 0.33 μM based on the 3σ method which defined the LoD as three multiplied standard deviation (σ) of the converted concentrations from signals of the blank samples upon the regression line obtained from the linear range of the titration curve.32 Notably, the sensitivity was further enhanced compared to that of the DPV, where the quantitativity was not seen below 100 μM Tau due to the high background signal of the indicator K3FeCN6. In addition, the LoD value is lower than or comparable to those of high-performance liquid chromatography and other electrochemical sensors reported previously.33–35 To evaluate the selectivity of the MIP-OFET, several functional and structural analogs (i.e., Ala, Arg, Asp, Cys, Glu, and Mal) were also tested. As shown in Fig. 8, Tau resulted in the highest change in VTH value, indicating that the imprinted cavities of the MIP were selective for Tau.
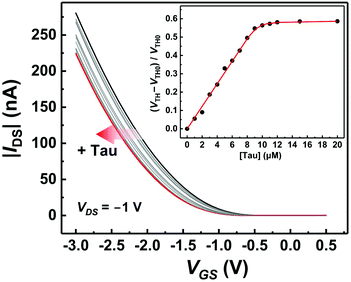 |
| Fig. 7 Transfer characteristics of the MIP-OFET upon the addition of Tau in a PBS (10 mM) with KCl (50 mM) at pH 7.4. Inset: Titration isotherm corresponding to the Tau-induced VTH shift. [Tau] = 0–20 μM. For each concentration, the measurement was performed for three times. The standard deviation of the titration profile was within 3%. | |
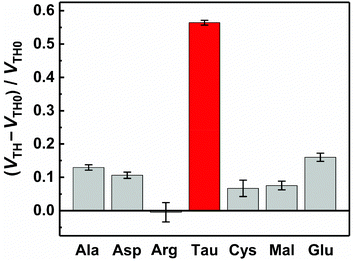 |
| Fig. 8 The VTH changes by the addition of Tau or its functional and structural analogues (Ala, Arg, Asp, Cys, Glu, and Mal) in a PBS (10 mM) with KCl (50 mM) at pH 7.4. For each analyte, the measurement was performed for three times. [Analyte] = 10 μM. | |
Furthermore, the reusability of the MIP-OFET was investigated (Fig. 9). The changes in the VTH value were recovered after rinsing the extended gate of the MIP-OFET with HClaq. (50 mM) and DI water, demonstrating that the MIP-OFET device can be reusable. Tau in the MIP would be protonated to its cationic form, followed by release from the MIP layer because of the weakened noncovalent interactions between Tau and MIP.36
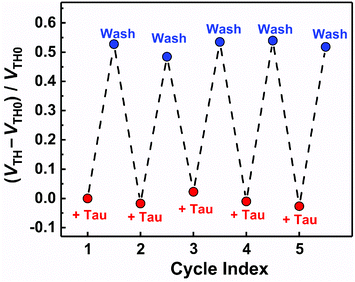 |
| Fig. 9 The reusability test for the MIP-OFET. [Tau] = 10 μM. After each Tau detection, the MIP was washed with HClaq. (50 mM) and DI water, and then Tau was added repeatedly. | |
Finally, Tau detection in the real-sample was attempted using the commercially available energy drink (Lipovitan). The Tau concentration in Lipovitan, which was estimated from the diluted sample solution detected by the MIP-OFET, was 77.0 mM. Compared to the declared Tau concentration in the same drink (79.9 mM), the error rate was only 3.64%. This result supported the feasibility of the MIP-OFET for the detection of nutrients in daily drinks.
Conclusions
In summary, we have successfully developed a selective and sensitive Tau sensor based on the novel MIP-OFET. The ideal molar ratio of the MIP for the Tau detection was determined by DFT and wave function calculations. The MIP was initially prepared by electrochemical polymerization with CV. The EIS and DPV measurements showed the Tau sensing ability of the MIP. The surface morphology of the extended gate reflected the detectability of the MIP. The fabricated MIP-OFET was successfully employed to detect Tau in a completely aqueous media. Most importantly, the MIP-OFET could be repeatedly used at least five times for Tau detection and quantification of Tau in daily drinks. Because MIPs can be prepared with various templates by rational molecular design, MIP-OFETs would greatly facilitate numerous applications of disposable but reusable chemical sensors in our daily lives.
Conflicts of interest
There are no conflicts to declare.
Acknowledgements
TM is grateful for the financial support from JSPS KAKENHI (Grant Numbers JP20H05207 and JP20K21204). We also acknowledge AGC for supplying CYTOP™.
References
- For reviews, see.
(a) H. Klauk, Chem. Soc. Rev., 2010, 39, 2643–2666 RSC;
(b) K. Fukuda and T. Someya, Adv. Mater., 2017, 29, 1602736–1602757 CrossRef;
(c) J. G. Mei, Y. Diao, A. L. Appleton, L. Fang and Z. N. Bao, J. Am. Chem. Soc., 2013, 135, 6724–6746 CrossRef CAS;
(d) M. Muccini, Nat. Mater., 2006, 5, 605–613 CrossRef CAS;
(e) A. R. Murphy and J. M. J. Frechet, Chem. Rev., 2007, 107, 1066–1096 CrossRef CAS;
(f) H. Sirringhaus, Adv. Mater., 2014, 26, 1319–1335 CrossRef CAS;
(g) Y. G. Wen, Y. Q. Liu, Y. L. Guo, G. Yu and W. P. Hu, Chem. Rev., 2011, 111, 3358–3406 CrossRef CAS;
(h) E. Macchia, R. A. Picca, K. Manoli, C. Di Franco, D. Blasi, L. Sarcina, N. Ditaranto, N. Cioffi, R. Osterbacka, G. Scamarcio, F. Torricelli and L. Torsi, Mater. Horiz., 2020, 7, 999–1013 RSC. For example, see.
(i) M. Kaltenbrunner, T. Sekitani, J. Reeder, T. Yokota, K. Kuribara, T. Tokuhara, M. Drack, R. Schwodiauer, I. Graz, S. Bauer-Gogonea, S. Bauer and T. Someya, Nature, 2013, 499, 458–463 CrossRef CAS.
-
(a) R. Kubota, Y. Sasaki, T. Minamiki and T. Minami, ACS Sens., 2019, 4, 2571–2587 CrossRef CAS;
(b) T. Minamiki, T. Minami, R. Kurita, O. Niwa, S. Wakida, K. Fukuda, D. Kumaki and S. Tokito, Appl. Phys. Lett., 2014, 104, 243703–243706 CrossRef.
- T. Minami, Y. Sasaki, T. Minamiki, P. Koutnik, P. Anzenbacher and S. Tokito, Chem. Commun., 2015, 51, 17666–17668 RSC.
- T. Minami, T. Minamiki and S. Tokito, Chem. Commun., 2015, 51, 9491–9494 RSC.
- T. Minami, T. Minamiki, Y. Hashima, D. Yokoyama, T. Sekine, K. Fukuda, D. Kumaki and S. Tokito, Chem. Commun., 2014, 50, 15613–15615 RSC.
- T. Minamiki, T. Minami, P. Koutnik, P. Anzenbacher and S. Tokitot, Anal. Chem., 2016, 88, 1092–1095 CrossRef CAS.
- For reviews, see.
(a) L. Torsi, M. Magliulo, K. Manoli and G. Palazzo, Chem. Soc. Rev., 2013, 42, 8612–8628 RSC;
(b) S. Yuvaraja, A. Nawaz, Q. Liu, D. Dubal, S. G. Surya, K. N. Salama and P. Sonar, Chem. Soc. Rev., 2020, 49, 3423–3460 RSC;
(c) H. Li, W. Shi, J. Song, H. J. Jang, J. Dailey, J. S. Yu and H. E. Katz, Chem. Rev., 2019, 119, 3–35 CrossRef CAS. For examples, see.
(d) W. G. Huang, K. Besar, R. LeCover, P. Dulloor, J. Sinha, J. F. M. Hardigree, C. Pick, J. Swavola, A. D. Everett, J. Frechette, M. Bevan and H. E. Katz, Chem. Sci., 2014, 5, 416–426 RSC;
(e) M. Xu, D. Obodo and V. K. Yadavalli, Biosens. Bioelectron., 2019, 124, 96–114 CrossRef.
- For reviews, see.
(a) Y. Fuchs, O. Soppera and K. Haupt, Anal. Chim. Acta, 2012, 717, 7–20 CrossRef CAS;
(b) K. Haupt and K. Mosbach, Chem. Rev., 2000, 100, 2495–2504 CrossRef CAS;
(c) T. Takeuchi and H. Sunayama, Chem. Commun., 2018, 54, 6243–6251 RSC;
(d) L. X. Chen, X. Y. Wang, W. H. Lu, X. Q. Wu and J. H. Li, Chem. Soc. Rev., 2016, 45, 2137–2211 RSC. For examples, see.
(e) L. J. Zhang, G. H. Wang, D. Wu, C. Xiong, L. Zheng, Y. S. Ding, H. B. Lu, G. B. Zhang and L. Z. Qiu, Biosens. Bioelectron., 2018, 100, 235–241 CrossRef CAS.
-
(a) B. Konig, M. Pelka, H. Zieg, T. Ritter, H. Bouas-Laurent, R. Bonneau and J. P. Desvergne, J. Am. Chem. Soc., 1999, 121, 1681–1687 CrossRef;
(b) X. Y. Wang, S. M. Yu, J. R. Wang, J. L. Yu, M. Arabi, L. W. Fu, B. W. Li, J. H. Li and L. X. Chen, Talanta, 2020, 211, 120727–120734 CrossRef CAS.
- For a review, see.
(a) M. Sobiech, P. Bujak, P. Luliński and A. Pron, Nanoscale, 2019, 11, 12030–12074 RSC. For examples, see.
(b) H. L. Liu, G. Z. Fang, H. D. Zhu, C. M. Li, C. C. Liu and S. Wang, Biosens. Bioelectron., 2013, 47, 127–132 CrossRef CAS;
(c) H. da Silva, J. G. Pacheco, J. Magalhaes, S. Viswanathan and C. Delerue-Matos, Biosens. Bioelectron., 2014, 52, 56–61 CrossRef CAS;
(d) H. Y. Wang, P. P. Cao, Z. Y. He, X. W. He, W. Y. Li, Y. H. Li and Y. K. Zhang, Nanoscale, 2019, 11, 17018–17030 RSC;
(e) A. Poma, A. Guerreiro, M. J. Whitcombe, E. V. Piletska, A. P. F. Turner and S. A. Piletsky, Adv. Funct. Mater., 2013, 23, 2821–2827 CrossRef CAS.
-
(a) C. J. Percival, S. Stanley, A. Braithwaite, M. I. Newton and G. McHale, Analyst, 2002, 127, 1024–1026 RSC;
(b) T. R. Ling, Y. Z. Syu, Y. C. Tasi, T. C. Chou and C. C. Liu, Biosens. Bioelectron., 2005, 21, 901–907 CrossRef CAS;
(c) D. M. Gao, Z. P. Zhang, M. H. Wu, C. G. Xie, G. J. Guan and D. P. Wang, J. Am. Chem. Soc., 2007, 129, 7859–7866 CrossRef CAS;
(d) T. Kobayashi, Y. Murawaki, P. S. Reddy, M. Abe and N. Fujii, Anal. Chim. Acta, 2001, 435, 141–149 CrossRef CAS.
- For a review, see.
(a) J. Wackerlig and P. A. Lieberzeit, Sens. Actuators, B, 2015, 207, 144–157 CrossRef CAS. For examples, see.
(b) L. Cenci, E. Andreetto, A. Vestri, M. Bovi, M. Barozzi, E. Iacob, M. Busato, A. Castagna, D. Girelli and A. M. Bossi, J. Nanobiotechnol., 2015, 13, 15 CrossRef;
(c) N. Cennamo, A. Dona, P. Pallavicini, G. D'Agostino, G. Dacarro, L. Zeni and M. Pesavento, Sens. Actuators, B, 2015, 208, 291–298 CrossRef CAS.
-
H. Zheng, presented in part at 18th International Conference on Solid-State Sensors, Actuators and Microsystems, New York, 2015.
- R. A. Nagel, A. Javaid, H. B. Meire, A. Wise, D. Westaby, J. Kavani, M. G. Lombard, R. Williams and M. E. Hodson, Lancet, 1989, 2, 1422–1425 CrossRef CAS.
- H. S. Geggel, M. E. Ament, J. R. Heckenlively, D. A. Martin and J. D. Kopple, N. Engl. J. Med., 1985, 312, 142–146 CrossRef CAS.
- J. R. Darsee and S. B. Heymsfield, N. Engl. J. Med., 1981, 304, 129–135 CrossRef CAS.
- For a review, see.
(a) J. Das, A. Roy and P. C. Sil, Food Funct., 2012, 3, 1251–1264 RSC. For example, see.
(b) D. G. Tu, Y. L. Chang, C. H. Chou, Y. L. Lin, C. C. Chiang, Y. Y. Chang and Y. C. Chen, Food Funct., 2018, 9, 124–133 RSC.
-
M. J. Frisch, G. W. Trucks, H. B. Schlegel, G. E. Scuseria, M. A. Robb, J. R. Cheeseman, G. Scalmani, V. Barone, G. A. Petersson, H. Nakatsuji, X. Li, M. Caricato, A. V. Marenich, J. Bloino, B. G. Janesko, R. Gomperts, B. Mennucci, H. P. Hratchian, J. V. Ortiz, A. F. Izmaylov, J. L. Sonnenberg, D. Williams-Young, F. Ding, F. Lipparini, F. Egidi, J. Goings, B. Peng, A. Petrone, T. Henderson, D. Ranasinghe, V. G. Zakrzewski, J. Gao, N. Rega, G. Zheng, W. Liang, M. Hada, M. Ehara, K. Toyota, R. Fukuda, J. Hasegawa, M. Ishida, T. Nakajima, Y. Honda, O. Kitao, H. Nakai, T. Vreven, K. Throssell, J. A. Montgomery Jr., J. E. Peralta, F. Ogliaro, M. J. Bearpark, J. J. Heyd, E. N. Brothers, K. N. Kudin, V. N. Staroverov, T. A. Keith, R. Kobayashi, J. Normand, K. Raghavachari, A. P. Rendell, J. C. Burant, S. S. Iyengar, J. Tomasi, M. Cossi, J. M. Millam, M. Klene, C. Adamo, R. Cammi, J. W. Ochterski, R. L. Martin, K. Morokuma, O. Farkas, J. B. Foresman and D. J. Fox, Gaussian 16 (Revision C.01), Gaussian, Inc., Wallingford CT, 2016 Search PubMed.
- C. Lefebvre, G. Rubez, H. Khartabil, J. C. Boisson, J. Contreras-Garcia and E. Henon, Phys. Chem. Chem. Phys., 2017, 19, 17928–17936 RSC.
- M. Halik, H. Klauk, U. Zschieschang, G. Schmid, C. Dehm, M. Schütz, S. Maisch, F. Effenberger, M. Brunnbauer and F. Stellacci, Nature, 2004, 431, 963–966 CrossRef CAS.
- I. McCulloch, M. Heeney, C. Bailey, K. Genevicius, I. Macdonald, M. Shkunov, D. Sparrowe, S. Tierney, R. Wagner, W. M. Zhang, M. L. Chabinyc, R. J. Kline, M. D. McGehee and M. F. Toney, Nat. Mater., 2006, 5, 328–333 CrossRef CAS.
-
(a) P. J. Stephens, F. J. Devlin, C. F. Chabalowski and M. J. Frisch, J. Phys. Chem., 1994, 98, 11623–11627 CrossRef CAS;
(b) R. Krishnan, J. S. Binkley, R. Seeger and J. A. Pople, J. Chem. Phys., 1980, 72, 650–654 CrossRef CAS;
(c) G. W. Spitznagel, T. Clark, P. V. Schleyer and W. J. Hehre, J. Comput. Chem., 1987, 8, 1109–1116 CrossRef CAS;
(d) S. Grimme, J. Antony, S. Ehrlich and H. Krieg, J. Chem. Phys., 2010, 132, 154104–154122 CrossRef.
-
(a) Y. Zhao and D. G. Truhlar, Theor. Chem. Acc., 2008, 120, 215–241 Search PubMed;
(b) F. Weigend and R. Ahlrichs, Phys. Chem. Chem. Phys., 2005, 7, 3297–3305 RSC;
(c) E. Papajak, J. J. Zheng, X. F. Xu, H. R. Leverentz and D. G. Truhlar, J. Chem. Theory Comput., 2011, 7, 3027–3034 CrossRef CAS;
(d) J. J. Zheng, X. F. Xu and D. G. Truhlar, Theor. Chem. Acc., 2011, 128, 295–305 Search PubMed;
(e) J. Tomasi, B. Mennucci and R. Cammi, Chem. Rev., 2005, 105, 2999–3093 CrossRef CAS.
- L. Goerigk, A. Hansen, C. Bauer, S. Ehrlich, A. Najibi and S. Grimme, Phys. Chem. Chem. Phys., 2017, 19, 32184–32215 RSC.
- T. Lu and F. W. Chen, J. Comput. Chem., 2012, 33, 580–592 CrossRef CAS.
- W. Humphrey, A. Dalke and K. Schulten, J. Mol. Graphics Modell., 1996, 14, 33–38 CrossRef CAS.
- S. Yagi, M. Fukuda, R. Makiura, T. Ichitsubo and E. Matsubara, J. Mater. Chem. A., 2014, 2, 8041–8047 RSC.
- S. Motia, B. Bouchikhi, E. Llobet and N. El Bari, Talanta, 2020, 216, 120953 CrossRef CAS.
- A. K. Singh and M. Singh, J. Mol. Recognit., 2017, 30, 9 CrossRef.
- I. H. Lambert and D. B. Hansen, Cell. Physiol. Biochem., 2011, 28, 1099–1110 CrossRef CAS.
- G. Horowitz, Adv. Mater., 1998, 10, 365–377 CrossRef CAS.
-
J. N. Miller and J. C. Miller, Statistics and Chemometrics for Analytical Chemistry, Pearson/Prentice Hall, Upper Saddle River, N.J., 2005 Search PubMed.
- I. Ferreira, M. V. Nunes, E. Mendes, F. Remião and M. A. Ferreira, J. Liq. Chromatogr. Relat. Technol., 1997, 20, 1269–1278 CrossRef CAS.
- J. Kupis-Rozmyslowicz, M. Wagner, J. Bobacka, A. Lewenstam and J. Migdalski, Electrochim. Acta, 2016, 188, 537–544 CrossRef CAS.
- M. Revenga-Parra, E. Martinez-Perinan, B. Moreno, F. Pariente and E. Lorenzo, Electrochim. Acta, 2017, 240, 506–513 CrossRef CAS.
- R. Ouyang, J. Lei, H. Ju and Y. Xue, Adv. Funct. Mater., 2007, 17, 3223–3230 CrossRef CAS.
Footnote |
† Electronic supplementary information (ESI) available: DFT calculation, cyclic voltammetry, electrochemical quartz crystal microbalance, differential pulse voltammetry, OFET fabrication, and transfer and output characteristics. See DOI: 10.1039/d0nr06920e |
|
This journal is © The Royal Society of Chemistry 2021 |