DOI:
10.1039/D0NJ04530F
(Paper)
New J. Chem., 2021,
45, 471-484
Synthesis, spectroscopic characterization and DNA/HSA binding studies of (phenyl/naphthyl)ethenyl-substituted 1,3,4-oxadiazolyl-1,2,4-oxadiazoles†
Received
10th September 2020
, Accepted 24th November 2020
First published on 24th November 2020
Abstract
Two new series of conjugated arylethenyl-1,3,4-oxadiazolyl-1,2,4-oxadiazoles were obtained and spectroscopically characterized in terms of UV-Vis absorption, fluorescence and interaction with CT-DNA and Human Serum Albumin (HSA) biomolecules. Phenyl- and 1-naphthyl-bearing examples were analysed, and the spectroscopic properties of its substitution series were compared, showing extensive conjugation in all compounds and absorption differences due to both the aryl–ethenyl subunit and substituted phenyl/phenylene at the 1,2,4-oxadiazole side. Strong binding interactions of the obtained compounds with CT-DNA and moderate HSA-association capability were observed spectroscopically, and further docking studies were performed.
Introduction
Heterocyclic compounds, in particular azoles, have long been the object of much attention in chemistry.1 Among nitrogen-bearing heterocycles, oxadiazoles represent a significant portion of target molecules in organic synthesis.2 In addition to the methods themselves, focus is placed on their pharmacological activities and also on their physical–chemical properties.3 1,2,4- And 1,3,4-oxadiazoles are the most described oxadiazole regioisomers, and these have been incorporated in a great number of functional molecules, including antitumor,4 antiviral5 and antidepressant drugs,6 as well as in polymeric species.7 Moreover, due to their highly conjugated structure, aryl-1,3,4-oxadiazoles and their vinylogous counterparts have often been used in the synthesis of fluorescent compounds.8
Molecules containing the di-heterocyclic moiety 1,3,4-oxadiazolyl-1,2,4-oxadiazole have been studied recently by our group, having electron-withdrawing properties associated to the oxadiazoles and displaying significant binding affinity towards CT-DNA.9 However, the fluorescence spectroscopic properties of molecules based on this di-heterocyclic scaffold remain uncharacterized.
Since there is interest in DNA-binding molecules to be employed as drugs and/or biochemical probes, fluorescent 1,3,4-oxadiazolyl-1,2,4-oxadiazole derivatives may deserve attention for the development of such compounds.10 Considering other results obtained previously for ferrocene-substituted chemical species,9 it would be plausible to study any observable fluorescence after the replacement of ferrocene by other aryl groups, making fluorescence spectroscopic characterization of the respective DNA and/or protein-compound adducts possible.
In this work, a library of new fluorescent (phenyl/naphthyl)-ethenyl-1,3,4-oxadiazolyl-1,2,4-oxadiazoles (7aa–be, Fig. 1) were prepared and fully characterized using analytical techniques. The photophysical properties of these derivatives were evaluated via UV-Vis and steady-state fluorescence emission spectroscopy. Moreover, DNA and HSA binding assays were conducted through spectroscopic and theoretical analyses (molecular docking).
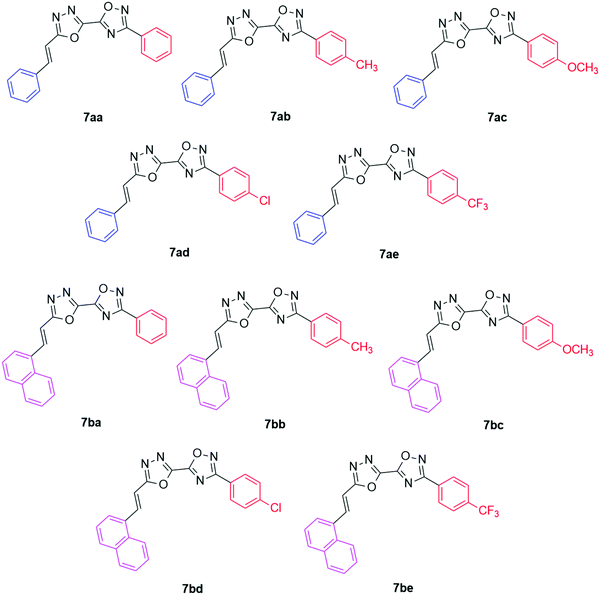 |
| Fig. 1 Structural representation of the 1,3,4-oxadiazolyl-1,2,4-oxadiazoles 7aa–be. | |
Experimental
General analysis
High-resolution mass spectra (HRMS) were obtained for compounds 7aa–be on a XEVO G2-Q-TOF (Waters) mass spectrometer, operating in the ESI(+) mode, at the Chemistry Department, Federal University of Santa Maria.
Thin layer chromatography (TLC) was performed using Merck Silica Gel GF254 (0.25 mm). For visualization, TLC plates were either placed under ultraviolet light or stained with iodine vapor or acidic vanillin solution, followed by heating.
Proton nuclear magnetic resonance spectra (1H NMR) were obtained at 400 MHz using a Bruker Avance III HD NMR spectrometer. The spectra were recorded in either CDCl3 or DMSO-d6 solution. Chemical shifts are reported in ppm, referenced to the solvent peak of tetramethyl silane (TMS) as the external reference. Data are reported as follows: chemical shift (δ) expressed in ppm, multiplicity (br = broad, s = singlet, d = doublet, dd = doublet of doublets, ddd = doublet of doublet of doublets, dt = doublet of triplets, t = triplet, m = multiplet, q = quartet), coupling constant (J) in Hertz and integrated intensity. Carbon-13 nuclear magnetic resonance (13C NMR) spectra were obtained at 100 MHz using an AVANCE III HD NMR spectrometer. Chemical shifts (δ) are reported in ppm, referenced to the solvent peak of CDCl3 or DMSO-d6. Deuterated solvents were acquired from Cambridge Isotope Laboratories®, having TMS added on opening.
X-ray crystallography
Crystallographic data were collected on a Bruker D8 Venture Photon 100 diffractometer equipped with an Incoatec IμS high brilliance Mo-Kα X-ray tube with two-dimensional Montel micro-focusing optics. The structure was solved by direct methods using SHELXS.11 Subsequent Fourier-difference map analyses yielded the positions of the non-hydrogen atoms. Refinements were carried out using the SHELXL package.11 All refinements were made by full-matrix least-squares on F2 with anisotropic displacement parameters for all non-hydrogen atoms. Hydrogen atoms were included in the refinement in calculated positions but the atoms (of hydrogens) that are involved in special bonds were located in the Fourier map. Drawings were done using ORTEP for Windows.12 Crystal data and more details of the data collection and refinements of the ligand are provided in the ESI† (Fig. S31).
Photophysical analysis
Electronic UV-Vis absorption spectra were recorded using a Shimadzu UV-2600 spectrophotometer (data interval, 1.0 nm) using chloroform and DMSO as solvents. Steady-state fluorescence spectra of samples in CHCl3 and DMSO solutions were measured using a Varian Cary 50 fluorescence spectrophotometer (emission; slit 2.5 mm) and were corrected according to the manufacturer's instructions. Fluorescence quantum yields (Φf) of compounds 7aa–ae/7ba–be in the solutions were determined by comparing the corrected fluorescence spectra with that of 9,10-diphenylanthracene (DPA) in chloroform (Φf = 0.65, λex = 366 nm) as the fluorescence yield standard.13 Collecting the samples and the standard fluorescence spectra under the same experimental condition, the fluorescence quantum yield was calculated using eqn (1): | 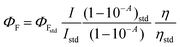 | (1) |
where ΦF, I, A and η are the fluorescence quantum yield, the integral area of fluorescence emission, the absorbance at λexc and the refractive index of selected solvents, respectively. The subscript “std” refers to the standard molecule – in this case, 9,10-diphenylanthracene (DPA) (ΦF = 0.65 in chloroform solution).
CT-DNA binding assays
The interactions between calf-thymus DNA (CT-DNA) and compounds 7aa–ae/7ba–be were studied via UV-Vis absorption measurements at room temperature in PBS buffer at pH 7.2 using DMSO stock solution of derivatives (10−4 M range) from 300 to 700 nm. The DNA pair base concentrations of low molecular weight DNA from CT-DNA were determined by absorption spectroscopy using a molar extinction coefficient of 6600 M−1 cm−1 (per base pair) at λmax = 260 nm. Heterocyclic compound solutions in DMSO with PBS buffer were titrated with increasing concentrations of CT-DNA (ranging from 0 to 100 μM). The intrinsic binding constants (Kb) of derivatives were calculated according to the decay of the absorption bands of compounds using eqn (2) through a plot of [DNA]/(εa − εf) versus [DNA]: | 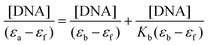 | (2) |
where [DNA] is the concentration of CT-DNA in the base pairs, εa is the extinction coefficient (Aobs/[compound]), and εb and εf are the extinction coefficients of free and fully bound forms, respectively. In plots of [DNA]/(εa − εf) versus [DNA], Kb is given by the ratio of the slope to the intercept.
Also, the standard Gibbs free-energy (ΔG°) of the CT-DNA:derivative adduct was calculated from the values of Kb using eqn (3):
| ΔG° = RT ln Kb | (3) |
where
R and
T are the gas constant (1.987 kcal K
−1 mol
−1) and temperature (298 K), respectively.
Competitive binding studies were conducted via steady-state fluorescence emission analysis using the same fluorimeter described in the photophysical analysis. Derivatives 7ba–be were dissolved in DMSO (stock solution in the 10−5 M range) and competitive studies were performed through the gradual addition of the compounds to a quartz cuvette (1.0 cm path length) containing ethidium bromide (EB, 2.0 × 10−7 M) and DNA (2.0 × 10−5 M) in PBS (pH 7.2). The concentrations of derivatives ranged from 0 to 100 μM. Compounds were excited at λexc = 510 nm and steady-state fluorescence emission spectra were recorded in the 550–800 nm range after 5 min of incubation. Stern–Volmer quenching constant (KSV) values were calculated according to the decay of the emission bands of EB–DNA using eqn (4) through a plot of F0/F versus [DNA]:
| 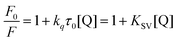 | (4) |
where
F and
F0 are the fluorescence intensities in the presence and absence of a quencher, respectively;
KSV,
kq,
τ0 and [Q] denote the Stern–Volmer quenching constant, bimolecular quenching rate constant, fluorescence lifetime of EB-DNA adducts (23 ns)
14 and the concentration of the quencher, respectively. According to
eqn (4), the
KSV values were calculated from the slope and
kq is equal to
KSV/
τ0.
In order to quantify the displacement ability of the derivatives under study, a minimum ligand concentration was used, which decreases in about 50% of the EB fluorescence emission (in this case, assumed to be 50% displacement of EB).15 The values of the apparent binding constant with CT-DNA (Kapp) were calculated using eqn (5):
| KEB[EB] = Kapp[compound] | (5) |
where
KEB (4.95 × 10
5 M
−1) is the DNA-binding constant of EB, [EB] is the EB concentration (1.40 × 10
−6 M), and [compound] is the concentration of the derivatives used to obtain a 50% reduction in the fluorescence emission intensity of the EB dye.
Steady-state fluorescence emission for HSA-binding assays
The experimental binding ability between human serum albumin (HSA) and compounds 7aa–ae/7ba–be was evaluated through steady-state fluorescence emission measurements at room temperature in PBS buffer (pH 7.2) using DMSO stock solution of heterocyclic derivatives (10−5 M range). The excitation and emission fluorescence wavelengths of 290 and 300–500 nm, respectively, were used. In order to obtain quantitative values for albumin fluorescence quenching data (KSV and kq values), the same Stern–Volmer approximation (eqn (4)) described in the CT-DNA binding assays was used; however, for HSA a fluorescence lifetime of τ0 = 5.67 ns was used.16
Generally, double logarithmic approximation (eqn (6)) is used to estimate the Stern–Volmer binding constant (Ka) and the number of binding sites (n) for the compound–HSA adduct:
| 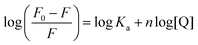 | (6) |
where
F0 and
F represent fluorescence intensities in the absence and presence of derivatives, respectively, while [Q] is the quencher concentration. According to
eqn (6), the
Ka values were calculated from the intercept and
n by the slope.
The standard Gibbs free-energy (ΔG°) for the compound-HSA adduct was calculated from the Ka values using eqn (3) previously described in the CT-DNA binding assays.
Molecular docking procedure
The chemical structure for the naphthylethenyl-substituted 1,3,4-oxadiazolyl-1,2,4-oxadiazoles 7aa–ae and 7ba–be was built and energy-minimized using Density Functional Theory (DFT) calculations with the B3LYP potential and basis set 6-31G*, available in the Spartan’18 software (Wavefunction Inc., Irvine, CA, USA).17 The crystallographic structures of HSA and DNA were obtained from the Protein Data Bank (PDB) with access codes 1N5U18 and 1BNA,19 respectively. The molecular docking studies were performed using the GOLD 5.6 software (CCDC, Cambridge Crystallographic Data Centre, CB2 1EZ, UK).20 The hydrogen atoms were added to the structure of biomacromolecules according to the data inferred by the GOLD 5.6 software on the ionization and tautomeric states. For the albumin, molecular docking calculations were performed with a 10 Å radius around the three main binding pockets: sites I, II, and III. On the other hand, for the DNA structure, a 5.0 Å radius around the major and minor grooves of the double helix was defined for the molecular docking calculations. The number of genetic operations (crossover, migration, mutation) in each docking run used in the search procedure was set to 100
000. The scoring function used was ‘ChemPLP’, which is the default function of the GOLD 5.6 software. The figures were generated using PyMOL Molecular Graphics System 1.0 level software (Delano Scientific LLC software San Carlos, CA, USA).21
Synthetic procedures
All reactants and solvents, unless noted, were commercially available (Sigma-Aldrich®, Alfa-Aesar®, Merck®, Vetec® and Synth®). Solvents were purified in accordance to standard procedures, namely by means of distillation and drying over molecular sieves, as described elsewhere. Intermediates and products were prepared as described below.
(E)-3-Arylprop-2-enoic acids (2a–b)22.
To a round-bottom two-necked balloon, under magnetic stirring, aldehyde 1a–b (10 mmol) and malonic acid (10 mmol) were dissolved in pyridine (10 mL), and 5 drops of piperidine was added to the mixture. The system was stirred at reflux temperature for 15 h. Then, the mixture was poured on water–ice (50 mL) and acidified with 6 M aqueous HCl until pH 2. The suspension was extracted three times with ethyl acetate (50 ml each time). The combined organic phase was dried over anhydrous magnesium sulfate and then filtered. The solvent was removed by rotary evaporation under reduced pressure, and the product was recrystallized from dichloromethane/hexane.
(E)-3-Phenylprop-2-enoic acid (cinnamic acid) (2a).
Yield = 0.977 g, 66% (white solid); mp = 126.7–130.8 °C. 1H NMR (CDCl3, 400 MHz), δ (ppm): 7.67–7.65 (m, 2 H), 7.60 (d, J = 16.0 Hz, 1 H), 7.42–7.39 (m, 3 H), 6.52 (d, J = 16.0 Hz, 1 H). 13C NMR (CDCl3, 100 MHz), δ (ppm): 167.5, 143.9, 134.2, 130.1, 128.9, 128.1, 119.2.
(E)-3-(Naphthalen-1-yl)prop-2-enoic acid (2b).
Yield = 1.188 g, 60% (yellow solid); mp = 152.7–156.8 °C. 1H NMR (CDCl3, 400 MHz), δ (ppm): 8.40 (d, J = 15.8 Hz, 1 H), 8.18 (d, J = 8.4 Hz, 1 H), 8.01–7.92 (m, 3 H), 7.63–7.52 (m, 3 H), 6.61 (d, J = 15.7 Hz, 1 H). 13C NMR (CDCl3, 100 MHz), δ (ppm): 167.6, 140.3, 133.4, 131.1, 130.9, 130.5, 128.8, 127.3, 126.4, 125.8, 125.3, 123.1, 122.0.
Arylamidoximes (4a–e)23
To a round-bottom two-necked balloon, under magnetic stirring, aryl nitrile 3a–e (15 mmol) was dissolved in ethanol (25 mL) and to the solution hydroxylamine hydrochloride (2.085 g, 30 mmol) and aqueous NaOH (2 equiv, 10 mL) were added. The mixture was stirred at room temperature for 15 to 24 h, and the reaction was monitored by TLC. Then, the solvent was removed by rotary evaporation and water was added to the crude mixture (20 mL). The mixture was extracted with ethyl acetate (3 × 20 mL) and the organic layer was dried over anhydrous MgSO4 and filtered. The solvent was removed by rotary evaporation and the product was recrystallized from chloroform–hexane.
(Z)-N′-Hydroxybenzimidamide (4a)
Yield = 2.000 g, 98% (white solid). 1H NMR (DMSO-d6, 400 MHz), δ (ppm): 9.52 (br, 1 H), 7.68 (ddd, J1 = 5.5 Hz; J2 = 3.0 Hz; J3 = 1.5 Hz, 2 H), 7.39–7.35 (m, 3 H), 5.69 (br, 2 H). 13C NMR (DMSO-d6, 100 MHz) δ (ppm): 150.8, 133.3, 128.7, 127.9, 125.3.
(Z)-N′-Hydroxy-4-methylbenzimidamide (4b)
Yield = 1.395 g, 62% (white solid). 1H NMR (DMSO-d6, 400 MHz), δ (ppm): 9.46 (br, 1 H), 7.56 (d, J = 8.0 Hz, 2 H), 7,17 (d, J = 7.8 Hz, 2 H), 5.64 (br, 2 H), 2.30 (s, 3 H). 13C NMR (DMSO-d6, 100 MHz) δ (ppm): 150.9, 138.3, 130.5, 128.6, 125.3, 20.7.
(Z)-N′-Hydroxy-4-methoxybenzimidamide (4c)
Yield = 2.116 g, 85% (grey-white solid). 1H NMR (DMSO-d6, 400 MHz), δ (ppm): 9,41 (br, 1 H), 7,61 (d, J = 8.9 Hz, 2 H), 6.92 (d, J = 8.9 Hz, 2 H), 5.66 (br, 2 H), 3.77 (s, 3 H). 13C NMR (DMSO-d6, 100 MHz) δ (ppm): 159.8, 150.7, 126.7, 125.7, 113.4, 55.1.
(Z)-N′-Hydroxy-4-chlorobenzimidamide (4d)
Yield = 2.532 g, >95% (white solid). 1H NMR (DMSO-d6, 400 MHz), δ (ppm): 9.68 (s, 1 H), 7.69 (d, J = 7.7 Hz, 2 H), 7.42 (d, J = 8.9 Hz, 2 H), 5.80 (br, 2H). 13C NMR (DMSO-d6, 100 MHz) δ (ppm): 150.0, 133.5, 132.2, 128.1, 127.1.
(Z)-N′-Hydroxy-4-trifluoromethylbenzimidamide (4e)
Yield = 3.029 g, >95% (white solid). 1H NMR (DMSO-d6, 400 MHz), δ (ppm): 9.88 (s, 1 H), 7.90 (d, J = 8.0 Hz, 2 H), 7.72 (d, J = 8.2 Hz, 2 H), 5.92 (br, 2 H). 13C NMR (DMSO-d6, 100 MHz) δ (ppm): 149.9, 137.3, 129.1 (q, J = 31.7 Hz); 126.1, 124.9 (q, J = 3.8 Hz); 124.2 (q, J = 272.2 Hz).
Ethyl 3-aryl-1,2,4-oxadiazole-5-carboxylates (5a–e)24
To a round-bottom two-necked balloon mounted with a reflux condenser, under magnetic stirring, amido-oxime 4a–e (10 mmol) was dissolved in tetrahydrofuran (20 mL). Thereafter, N,N′-diisopropylethylamine (2.6 mL, 15 mmol) was added and the system was cooled to 0–5 °C. Then, ethyl oxalyl chloride (1.3 mL, 12 mmol) was added dropwise under stirring; the reaction mixture was warmed to room temperature and kept under reflux for 2 h. After completion, the reaction mixture was cooled to room temperature, then aqueous 2 N HCl solution (10 mL) and water (100 mL) were added and the product was extracted with ethyl acetate (3 × 25 mL). The organic layer was washed with aqueous NaHCO3 and then with water. The organic layer was dried over anhydrous MgSO4 and filtered, and the solvent was removed by rotary evaporation. The crude product was purified through flash column chromatography on silica gel using hexane
:
ethyl acetate (85
:
15 v/v) and dried under reduced pressure.
Ethyl 3-phenyl-1,2,4-oxadiazole-5-carboxylate (5a)
Yield = 1.809 g, 83% (white solid). 1H NMR (CDCl3, 400 MHz), δ (ppm): 8.15 (d, 2 H, J = 6.6 Hz), 7.56–7.47 (m, 3 H), 4.57 (q, J = 7.1 Hz, 2 H), 1.49 (t, J = 7.1 Hz, 3 H). 13C NMR (CDCl3, 100 MHz) δ (ppm): 169.5, 166,7, 154.3, 131.8, 129.0, 127.8, 125.8, 63.9, 14.1.
Ethyl 3-(4-methylphenyl)-1,2,4-oxadiazole-5-carboxylate (5b)
Yield = 1.694 g, 73% (white solid). 1H NMR (CDCl3, 400 MHz), δ (ppm): 8.03 (d, J = 8.2 Hz, 2 H), 7.29 (d, J = 8.0 Hz, 2 H), 4.56 (q, J = 7.2 Hz, 2 H), 2.41 (s, 3 H), 1.48 (t, J = 7.2 Hz, 3 H). 13C NMR (CDCl3, 100 MHz) δ (ppm): 169.5, 166.5, 154.2, 142.3, 129.7, 127.6, 122.9, 63.8, 21.5, 14.0.
Ethyl 3-(4-methoxyphenyl)-1,2,4-oxadiazole-5-carboxylate (5c)
Yield = 1.835 g, 74% (white solid). 1H NMR (CDCl3, 400 MHz), δ (ppm): 8.07 (d, J = 9.0 Hz, 2 H), 6.99 (d, J = 9.0 Hz, 2H), 4.56 (q, J = 7.1 Hz, 2 H), 3.87 (s, 3 H), 1.48 (t, J = 7.1 Hz, 3 H). 13C NMR (CDCl3, 100 MHz) δ (ppm): 169.2, 166.4, 162.5, 154.3, 129.4, 118.1, 114.4, 63.8, 55.4, 14.0.
Ethyl 3-(4-chlorophenyl)-1,2,4-oxadiazole-5-carboxylate (5d)
Yield = 2.146 g, 85% (white solid). 1H NMR (CDCl3, 400 MHz), δ (ppm): 8.08 (d, J = 8.7, 2 H), 7.48 (d, J = 8.7 Hz, 2 H), 4.57 (q, J = 7.2 Hz, 2 H), 1.49 (t, J = 7.2 Hz, 3 H). 13C NMR (CDCl3, 100 MHz) δ (ppm): 168.7, 166.8, 154.0, 138.1, 129.34; 129.0, 124.2, 63.9, 14.0.
Ethyl 3-(4-trifluoromethylphenyl)-1,2,4-oxadiazole-5-carboxylate (5e)
Yield = 2.460 g, 86% (white solid). 1H NMR (CDCl3, 400 MHz), δ (ppm): 8.28 (d, J = 8.0 Hz, 2 H), 7.77 (d, J = 8.1 Hz, 2 H), 4.59 (q, J = 7.1 Hz, 2 H), 1.50 (t, J = 7.1 Hz, 3 H). 13C NMR (CDCl3, 100 MHz) δ (ppm): 168.4, 167.1, 153.9, 133.6 (q, J = 32.8 Hz), 129.1, 128.1, 126.0 (q, J = 3.7 Hz), 123.6 (q, J = 272.8 Hz), 64.0, 13.9.
3-Aryl-1,2,4-oxadiazole-5-carbohydrazides (6a–e)25
In a round-bottom flask, ethyl 3-aryl-1,2,4-oxadiazole-5-carboxylate 5a–e (6 mmol) was dissolved in ethanol (15 mL) under magnetic stirring. Then, hydrazine hydrate (0.6 mL, 12 mmol) was added and a precipitate of the hydrazide was readily formed. The mixture was kept under stirring at room temperature for 1 h, after which the precipitated hydrazide was collected by vacuum filtration and washed with cold ethanol (50 mL). The solid was transferred to another balloon and dried under reduced pressure.
3-Phenyl-1,2,4-oxadiazole-5-carbohydrazide (6a)
Yield = 1.222 g, >95% (white solid). 1H NMR (DMSO-d6, 400 MHz), δ (ppm): 10.66 (br, 1 H), 8.05 (d, J = 7.9 Hz, 2 H), 7.65–7.57 (m, 3 H), 4.99 (br, 2 H). 13C NMR (DMSO-d6, 100 MHz) δ (ppm): 169.0, 167.9, 151.7, 131.8, 129.2, 127.0, 125.4.
3-(4-Methylphenyl)-1,2,4-oxadiazole-5-carbohydrazide (6b)
Yield = 1.086 g, 83% (white solid). 1H NMR (DMSO-d6, 400 MHz), δ (ppm): 7.92 (d, J = 8.2 Hz, 2 H), 7.38 (d, J = 7.9 Hz, 2 H), 2.38 (s, 3 H). 13C NMR (DMSO-d6, 100 MHz) δ (ppm): 168.9, 167.9, 151.8, 141.9, 129.8, 127.1, 122.7, 21.0.
3-(4-Methoxyphenyl)-1,2,4-oxadiazole-5-carbohydrazide (6c)
Yield = 1.264 g, 90% (white solid). 1H NMR (DMSO-d6, 400 MHz), δ (ppm): 7.98 (d, J = 9.0 Hz, 2 H), 7.13 (d, J = 9.0 Hz, 2 H), 3.84 (s, 3 H). 13C NMR (DMSO-d6, 100 MHz) δ (ppm): 168.8, 167.7, 162.0, 157.8, 151.8, 128.9, 117.8, 114.8, 55.4.
3-(4-Chlorophenyl)-1,2,4-oxadiazole-5-carbohydrazide (6d)
Yield =1.428 g, >95% (white solid). 1H NMR (DMSO-d6, 400 MHz), δ (ppm): 10.78 (br, 1 H), 8.04 (d, J = 8.7 Hz, 2 H); 7.66 (d, J = 8.7 Hz, 2 H), 4.95 (br, 2 H). 13C NMR (DMSO-d6, 100 MHz) δ (ppm): 169.2, 167.2, 151.6, 136.7, 129.5, 128.9, 124.4.
3-(4-Trifluoromethylphenyl)-1,2,4-oxadiazole-5-carbohydrazide (6e)
Yield = 1.306 g, 80% (white solid). 1H NMR (DMSO-d6, 400 MHz), δ (ppm): 10.84 (br, 1 H), 8.24 (d, J = 8.1 Hz, 2 H), 7.96 (d, J = 8.1 Hz, 2 H), 5.03 (br, 2H). 13C NMR (DMSO-d6, 100 MHz) δ (ppm): 169.5, 167.0, 151.6, 131.7 (q, J = 32.1 Hz), 129.4, 128.0, 126.3 (q, J = 3.6 Hz), 123.7 (q, J = 272.7 Hz).
(E)-3-Aryl-5-(5-(2-arylethen-1-yl)-1,3,4-oxadiazol-2-yl)-1,2,4-oxadiazoles (7aa–be)9,26
To a round-bottom, two-necked balloon, carboxylic acid 2a–b (0.5 mmol) and appropiate hydrazide 6a–e (0.5 mmol) were suspended in dichloromethane (7.0 mL), and triethylamine (5 equiv.) was added. Then, TBTU (1.1 equiv.) was added and the mixture was stirred at room temperature for 2 h. After completion, as verified by TLC, 4-toluenesulfonyl chloride (3 equiv.) was added to the reaction vessel and the mixture was stirred at room temperature for 2 h. The reaction was quenched using 5.0 mL of aqueous 35% ammonia solution, and stirring was continued for an additional 15 min. Dichloromethane (20 mL) was added and the organic phase was transferred to an Erlenmeyer flask, and then it was dried over anhydrous magnesium sulphate. The resulting phase was filtered and the solvent was removed by rotary evaporation under reduced pressure. The crude product was purified through flash column chromatography employing the hexane/ethyl acetate/dichloromethane (7
:
2
:
1, v/v) mixture, and the solvent was removed under reduced pressure.
(E)-3-Phenyl-5-(5-(2-phenylethen-1-yl)-1,3,4-oxadiazol-2-yl)-1,2,4-oxadiazole (7aa)
Yield = 0.114 g, 72% (white solid). mp = 151.8–153.8 °C. 1H NMR (CDCl3, 400 MHz), δ (ppm): 8.20 (d, J = 8.1 Hz, 2 H), 7.85 (d, J = 16.5 Hz, 1 H), 7.63–7.60 (m, 2 H), 7.57–7.51 (m, 3 H), 7.47–7.44 (m, 3 H), 7.13 (d, J = 16.5 Hz, 1 H). 13C NMR (CDCl3, 100 MHz) δ (ppm): 169.5, 166.2, 162.8, 152.6, 142.5, 134.1, 132.0, 130.8, 129.1, 129.0, 127.9, 127.7, 125.4, 108.3. HRMS-ESI(+) m/z, calcd. for C18H13N4O2 [M + H]+: 317.1039; found: 317.1026.
(E)-3-(4-Methylphenyl)-5-(5-(2-phenylethen-1-yl)-1,3,4-oxadiazol-2-yl)-1,2,4-oxadiazole (7ab)
Yield = 0.134 g, 81% (white solid); mp = 169.9–171.4 °C. 1H NMR (CDCl3, 400 MHz), δ (ppm): 8.08 (d, J = 8.2 Hz, 2 H), 7.84 (d, J = 16.5 Hz, 1 H), 7.62–7.59 (m, 2 H), 7.47–7.42 (m, 3 H), 7.32 (d, J = 7.9 Hz, 2 H), 7.12 (d, J = 16.4 Hz, 1 H), 2.43 (s, 3H). 13C NMR (CDCl3, 100 MHz) δ (ppm): 169.6, 166.2, 162.6, 152.7, 142.5, 142.5, 134.2, 130.8, 129.7, 129.1, 127.9, 127.7, 122.7, 108.4, 21.6. HRMS-ESI(+) m/z, calcd for C19H15N4O2 [M + H]+: 331.1195; found: 331.1197.
(E)-3-(4-Methoxyphenyl)-5-(5-(2-phenylethen-1-yl)-1,3,4-oxadiazol-2-yl)-1,2,4-oxadiazole (7ac)
Yield = 0.151 g, 87% (white solid); mp = 163.7–166.0 °C. 1H NMR (CDCl3, 400 MHz), δ (ppm): 8.12 (d, J = 8.9 Hz, 2 H), 7.83 (d, J = 16.5 Hz, 1 H), 7.62–7.59 (m, 2 H), 7.47–7.42 (m, 3 H), 7.12 (d, J = 16.5 Hz, 1 H), 7.01 (d, J = 8.9 Hz, 2 H), 3.87 (s, 3 H). 13C NMR (CDCl3, 100 MHz) δ (ppm): 169.2, 166.2, 162.6, 162.5, 152.7, 142.4, 134.1, 130.8, 129.4, 129.1, 127.9, 117.8, 114.5, 108.4, 55.4. HRMS-ESI(+) m/z, calcd. for C19H15N4O3 [M + H]+: 347.1144; found: 347.1142.
(E)-3-(4-Chlorophenyl)-5-(5-(2-phenylethen-1-yl)-1,3,4-oxadiazol-2-yl)-1,2,4-oxadiazole (7ad)
Yield = 0.130 g, 74% (white solid), mp = 176.7–179.2 °C. 1H NMR (CDCl3, 400 MHz), δ (ppm): 8.14 (d, J = 8.8 Hz, 2 H), 7.85 (d, J = 16.5 Hz, 1 H), 7.62–7.59 (m, 2 H), 7.51 (d, J = 8.8 Hz, 2 H), 7.48–7.43 (m, 3 H), 7.13 (d, J = 16.5 Hz, 1 H). 13C NMR (CDCl3, 100 MHz) δ (ppm): 168.8, 166.3, 163.0, 152.5, 142.7, 138.4, 134.1, 130.9, 129.4, 129.2, 129.1, 127.9, 124.0, 108.3. HRMS-ESI(+) m/z, calcd. for C18H12ClN4O2 [M + H]+: 351.0649; found: 351.0645.
(E)-3-(4-(Trifluoromethyl)phenyl)-5-(5-(2-phenylethen-1-yl)-1,3,4-oxadiazol-2-yl)-1,2,4-oxadiazole (7ae)
Yield = 0.086 g, 45% (white solid), mp = 192.0–195.3 °C. 1H NMR (CDCl3, 400 MHz), δ (ppm): 8.33 (d, J = 8.1 Hz, 2 H), 7.86 (d, J = 16.5 Hz, 1 H), 7.80 (d, J = 8.2 Hz, 2 H), 7.65–7.57 (m, 2 H), 7.49–7.41 (m, 3H), 7.14 (d, J = 16.5 Hz, 1 H). 13C NMR (CDCl3, 100 MHz) δ (ppm): 168.6, 166.4, 163.3, 152.5, 142.8, 134.2, 133.8 (q, J = 32.9 Hz), 130.9, 129.2, 128.9, 128.2, 128.0, 126.1 (q, J = 3.7 Hz), 123.6 (q, J = 272.5 Hz), 108.3. HRMS-ESI(+) m/z, calcd. for C19H12F3N4O2 [M + H]+: 385.0912; found: 385.0908.
(E)-3-Phenyl-5-(5-(2-(naphthalen-1-yl)ethen-1-yl)-1,3,4-oxadiazol-2-yl)-1,2,4-oxadiazole (7ba)
Yield = 0.101 g, 55% (yellow solid); mp = 155.8–159.8 °C. 1H NMR (CDCl3, 400 MHz), δ (ppm): 8.64 (d, J = 16.2 Hz, 1 H), 8.25–8.20 (m, 3 H), 7.93 (d, J = 8.2 Hz, 1 H), 7.89 (d, J = 8.2 Hz, 1 H), 7.85 (d, J = 7.2 Hz, 1 H), 7.63 (t, J = 7.7 Hz, 1 H), 7.57–7.51 (m, 5 H), 7.21 (d, J = 16.2 Hz, 1 H). 13C NMR (CDCl3, 100 MHz) δ (ppm): 169.6, 166.2, 162.8, 152.6, 139.4, 133.8, 132.0, 131.3, 131.2, 129.7, 129.0, 128.9, 127.8, 127.8, 127.2, 126.4, 125.5, 125.0, 123.0, 110.7. HRMS-ESI(+) m/z, calcd. for C22H15N4O2 [M + H]+: 367.1195; found: 367.1195.
(E)-3-(4-Methylphenyl)-5-(5-(2-(naphthalen-1-yl)ethen-1-yl)-1,3,4-oxadiazol-2-yl)-1,2,4-oxadiazole (7bb)
Yield = 0.114 g, 60% (yellow solid); m.p. = 135.4–139.1 °C. 1H NMR (CDCl3, 400 MHz), δ (ppm): 8.64 (d, J = 16.2 Hz, 1 H), 8.24 (d, J = 8.4 Hz, 1 H), 8.09 (d, J = 8.2 Hz, 2 H), 7.93 (d, J = 8.2 Hz, 1 H), 7.89 (d, J = 8.4 Hz, 1 H), 7.85 (d, J = 7.3 Hz, 1 H), 7.62 (t, J = 7.6 Hz, 1 H), 7.57–7.51 (m, 2 H), 7.33 (d, J = 8.0 Hz, 2 H), 7.20 (d, J = 16.2 Hz, 1 H), 2.43 (s, 3 H). 13C NMR (CDCl3, 100 MHz) δ (ppm): 169.6, 166.2, 162.6, 152.7, 142.5, 139.3, 133.8, 131.4, 131.3, 131.2, 129.8, 128.9, 127.7, 127.2, 126.5, 125.5, 125.0, 123.1, 122.7, 110.8, 21.6. HRMS-ESI(+) m/z, calcd. for C23H17N4O2 [M + H]+: 381.1352; found: 381.1364.
(E)-3-(4-Methoxyphenyl)-5-(5-(2-(naphthalen-1-yl)ethen-1-yl)-1,3,4-oxadiazol-2-yl)-1,2,4-oxadiazole (7bc)
Yield = 0.139 g, 70% (yellow solid); m.p. = 162.7–166.0 °C. 1H NMR (CDCl3, 400 MHz), δ (ppm): 8.65 (d, J = 16.2 Hz, 1 H), 8.25 (d, J = 8.5 Hz, 1 H), 8.15 (d, J = 9.0 Hz, 2 H), 7.94 (d, J = 8.1 Hz, 1 H), 7.90 (d, J = 7.7 Hz, 1 H), 7.86 (d, J = 7.3 Hz, 1 H), 7.63 (t, J = 7.6 Hz, 1 H), 7.58–7.52 (m, 2 H), 7.22 (d, J = 16.2 Hz, 1 H), 7.03 (d, J = 9.0 Hz, 2 H), 3.88 (s, 3 H). 13C NMR (CDCl3, 100 MHz) δ (ppm): 169.3, 166.2, 162.6, 162.5, 152.8, 139.4, 133.8, 131.5, 131.3, 131.2, 129.5, 128.9, 127.2, 126.5, 125.5, 125.0, 123.1, 117.9, 114.5, 110.8, 55.4. HRMS-ESI(+) m/z, calcd. for C23H17N4O3 [M + H]+: 397.1301; found: 397.1305.
(E)-3-(4-Chlorophenyl)-5-(5-(2-(naphthalen-1-yl)ethen-1-yl)-1,3,4-oxadiazol-2-yl)-1,2,4-oxadiazole (7bd)
Yield = 0.118 g, 59% (yellow solid); m.p. = 179.4–181.6 °C. 1H NMR (CDCl3, 400 MHz), δ (ppm): 8.66 (d, J = 16.2 Hz, 1 H), 8.25 (d, J = 8.5 Hz, 1 H), 8.16 (d, J = 8.8 Hz, 2 H), 7.96 (d, J = 8.2 Hz, 1 H), 7.91 (d, J = 8.1 Hz, 1 H), 7.87 (d, J = 7.2 Hz, 1 H), 7.64 (t, J = 8.4 Hz, 1 H), 7.59–7.55 (m, 2 H), 7.52 (d, J = 8.8 Hz, 2 H), 7.23 (d, J = 16.2 Hz, 1 H). 13C NMR (CDCl3, 100 MHz) δ (ppm): 168.8, 166.3, 162.9, 152.5, 139.5, 138.3, 133.8, 131.3, 131.2, 131.2, 129.4, 129.0, 128.9, 127.2, 126.5, 125.5, 125.0, 123.8, 123.0, 110.5. HRMS-ESI(+) m/z, calcd. for C22H14ClN4O2 [M + H]+: 401.0805; found: 401.0790.
(E)-3-(4-(Trifluoromethyl)phenyl)-5-(5-(2-(naphthalen-1-yl)ethen-1-yl)-1,3,4-oxadiazol-2-yl)-1,2,4-oxadiazole (7be)
Yield = 0.085 g, 39% (yellow solid); m.p. = 203.2–206.4 °C. 1H NMR (CDCl3, 400 MHz), δ (ppm): 8.72 (d, J = 16.2 Hz, 1 H), 8.38 (d, J = 8.1 Hz, 2 H), 8.27 (d, J = 8.5 Hz, 1 H), 7.98 (d, J = 8.2 Hz, 1 H), 7.93 (d, J = 7.7 Hz, 1 H), 7.90 (d, J = 7.1 Hz, 1 H), 7.83 (d, J = 8.2 Hz, 2 H), 7.66 (t, J = 7.6 Hz, 1 H), 7.61–7.56 (m, 2 H), 7.27 (d, J = 16.20 Hz, 1 H). 13C NMR (CDCl3, 100 MHz) δ (ppm): 168.6, 166.4, 163.2, 152.5, 139.7, 133.8, 133.6, 131.4, 131.3, 131.2, 129.0, 128.8, 128.2, 127.3, 126.5, 126.1 (q, J = 3.3 Hz), 125.6, 125.1, 123.6 (q, J = 273.4 Hz), 123.0, 110.6. HRMS-ESI(+) m/z, calcd. for C23H14F3N4O2 [M + H]+: 435.1069; found: 435.1053.
Results and discussion
Synthesis of the target compounds
The target compounds 7aa–be were obtained following the method previously adapted by our group,9 from Stabile et al. (2010),26 which involves the condensation of a carboxylic acid and a carbohydrazide, followed by cyclodehydration. Firstly, (E)-3-arylacrylic acids (2a–b) were prepared through Knoevenagel–Doebner condensation of aldehydes (1a–b) and malonic acid in pyridine.22 1,2,4-Oxadiazole-5-carbohydrazides (6a–e) were obtained using procedures from the literature, in three steps. In the first, nitriles (3a–e) were treated with hydroxylamine hydrochloride and aqueous sodium hydroxide in ethanol, giving the respective amidoximes (4a–e).23 These were reacted with ethyl oxalyl chloride under basic conditions (DIPEA) in THF, forming ethyl 1,2,4-oxadiazole-5-carboxylates (5a–e).24 The esters were then converted to hydrazides (6a–e), employing hydrazine hydrate in ethanol at room temperature25 (Scheme 1).
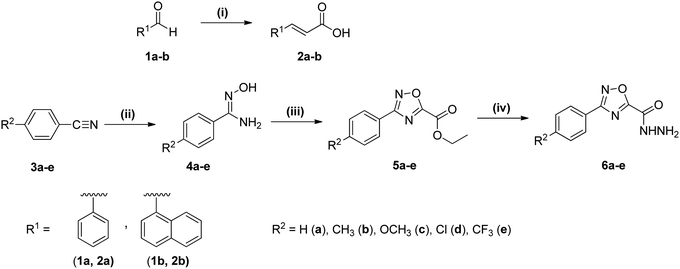 |
| Scheme 1 Synthetic route to acid 2 and to hydrazides 6a–e. Reaction conditions: (i) 1a–b, malonic acid, piperidine, pyridine, 100 °C, 15 h; (ii) NH2OH·HCl, EtOH, r. t., 15 h; (iii) EtO2CC(O)Cl, DIPEA, THF, reflux, 2 h; and (iv) NH2NH2·H2O, EtOH, r. t., 1 h. | |
Finally, the 1,3,4-oxadiazolyl-1,2,4-oxadiazoles 7aa–be were prepared through TBTU-promoted condensation of 3-arylacrylic acids 2a–b with the 1,2,4-oxadiazole-5-carbohydrazides 6a–e in the presence of triethylamine, followed by cyclodehydration of the diacylhydrazine intermediates employing 4-toluenesulfonyl chloride via a one-pot procedure. A substituent series of 10 compounds were prepared for comparison. The yields of examples bearing electron-withdrawing groups were lower than those of the analogs bearing electron-donating groups. The results are shown in Table 1.
Table 1 Substituent series of the 1,3,4-oxadiazolyl-1,2,4-oxadiazoles 7aa–bea
The identity of compounds 7aa–be was confirmed by means of 1H and 13C NMR spectroscopy and high-resolution mass spectroscopy (HRMS), as shown in Synthetic procedures section. 1H and 13C spectra of compounds 7aa–be are shown in the ESI† (Fig. S1–S20), as well as their HRMS spectra (Fig. S21–S30, ESI†). For the X-ray data of compound 7aa, see the ESI† (Fig. S31).
UV-Vis absorption and fluorescence emission properties of derivatives 7aa–ae and 7ba–be
The UV-Vis absorption and steady-state fluorescence emission spectra of derivatives 7aa–ae in CHCl3 are shown in Fig. 2 and the photophysical data are listed in Table 2. All derivatives showed transition bands located in the ultraviolet (UV) range, around 250–400 nm. The electronic transitions which can be related to π → π* type-transitions are observed and no significant shift was observed when changing the solvent polarity (see Table 2). The presence of a naphthyl moiety in compounds 7ba–be caused a significant change in the maximum absorption of the less energetic transition. This fact can be attributed to the greater contribution of aromaticity from the naphthyl group. The UV-Vis spectra of compounds 7aa–ae in DMSO and 7ba–be in CHCl3 and DMSO are shown in the ESI† (see Fig. S32 and S33).
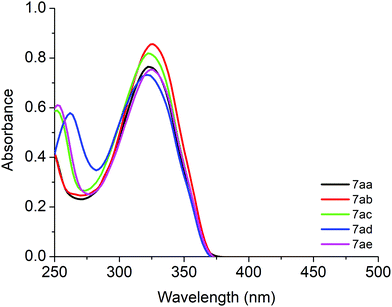 |
| Fig. 2 UV-Vis absorption spectra of derivatives 7aa–ae in CHCl3 solution ([ ] = 2.00 × 10−5 M). | |
Table 2 Photophysical data of compounds 7aa–ae and 7ba–be
Compound |
λ,a nm (ε; M−1 cm−1) |
Emission,b nm (Φf) |
SSc (nm) |
E
0–0
(eV) |
[ ] = 2.00 × 10−5 M.
[ ] = 10−6 M range at 298 K using 9,10-diphenylanthracene (DPA) in CHCl3 as the standard (Φf = 0.65).
Stokes shift equation: Δλ = λemission − λabsorption.
E
0–0 = 1240/λ (in eV); sh = shoulder.
|
In CHCl3 |
7aa
|
323 (38 250) |
426 (0.103) |
103.0 |
3.48 |
7ab
|
325 (42 700) |
432 (0.158) |
107.0 |
3.44 |
7ac
|
252 (29 350), 322 (40 850) |
429 (0.212) |
107.0 |
3.48 |
7ad
|
262 (28 850), 321 (36 500) |
425 (0.147) |
104.0 |
3.55 |
7ae
|
253 (30 450), 325 (37 650) |
429 (0.170) |
104.0 |
3.46 |
7ba
|
261 (sh), 359 (38 300) |
473 (0.274) |
114.0 |
3.04 |
7bb
|
360 (43 100) |
491 (0.335) |
131.0 |
3.03 |
7bc
|
356 (40 250) |
480 (0.015) |
124.0 |
3.15 |
7bd
|
260 (52 950), 356 (36 300) |
482 (0.357) |
126.0 |
3.06 |
7be
|
359 (40 400) |
481 (0.482) |
122.0 |
3.06 |
|
In DMSO |
7aa
|
324 (39 050) |
426 (0.097) |
102.0 |
3.50 |
7ab
|
326 (40 350) |
433 (0.154) |
107.0 |
3.42 |
7ac
|
325 (39 600) |
428 (0.199) |
103.0 |
3.43 |
7ad
|
259 (31 100), 323 (37 550) |
425 (0.134) |
102.0 |
3.52 |
7ae
|
325 (39 750) |
429 (0.168) |
104.0 |
3.40 |
7ba
|
259 (32 150), 357 (31 800) |
473 (0.300) |
116.0 |
3.00 |
7bb
|
357 (27 700) |
490 (0.348) |
133.0 |
2.94 |
7bc
|
258 (39 400), 349 (19 250) |
480 (0.142) |
131.0 |
3.16 |
7bd
|
256 (47 250), 355 (23 950) |
481 (0.377) |
126.0 |
2.97 |
7be
|
256 (46 200), 356 (25 450) |
481 (0.501) |
125.0 |
3.00 |
As an example, the steady-state fluorescence emission spectra of compounds 7ba–be in argon saturated CHCl3 solution are shown in Fig. 3. In general, all heterocycles contain one emission peak in the blue to cyan range (400 to 500 nm). As an example, emission band shifts (∼50–60 nm) can be seen when comparing derivatives 7aa (phenyl) and 7ba (naphthyl) in the same solvent. All the compounds showed fluorescence emission with moderate quantum yields (Φf) in both CHCl3 and DMSO solutions. The Φf values for these compounds also show differences; for example, compound 7ba (naphthyl and Ph groups), compound 7bc (naphthyl and 4-OCH3Ph groups) and compound 7be (naphthyl and 4-CF3Ph groups) in CHCl3 solution have distinct Φf values, which explains the influence of the donor/acceptor electronic properties, attributed to the resonance stabilization structures in the excited state (Table 2).
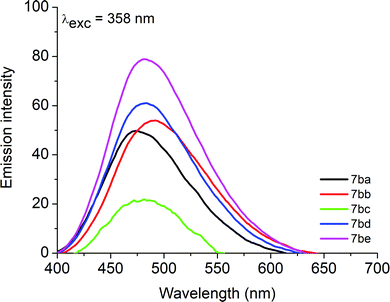 |
| Fig. 3 Steady-state fluorescence emission spectra of derivatives 7ba–be (λexc = 358 nm) in argon saturated chloroform solution ([ ] = 1.00 × 10−6 M). | |
Large Stokes shifts (SS) were observed in all derivative compounds, mainly in DMSO solution, and can be assigned to the ICT state that exists in these structures (Table 2). Steady-state fluorescence emission spectra of compounds 7aa–ae in CHCl3 and DMSO and 7ba–be in DMSO are shown in the ESI† (see Fig. S34 and S35). The visible appearance of representative examples 7aa and 7ba in solution (DMSO) is shown in the ESI† (see Fig. S36).
DNA-binding assays by UV-Vis absorption analysis
The interactions of compounds 7aa–ae and 7ba–be with the CT-DNA model were studied via UV-Vis spectroscopy in DMSO(2%)/Tris–HCl buffer pH 7.2 mixture solution, in the 250–500 nm range. All compounds interact with CT-DNA, producing an absorbance change in the ultraviolet region. As an example, the effect of different concentrations of DNA in the titration on the absorption spectra of compounds 7aa and 7ba is shown in Fig. 4.
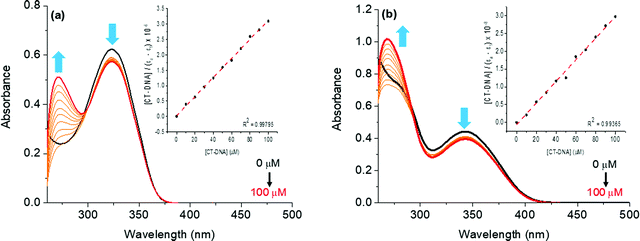 |
| Fig. 4 UV-Vis titration absorption spectra of derivatives (a) 7aa and (b) 7ba, in DMSO (2%)/Tris–HCl buffer (pH 7.2) solution. The concentration of CT-DNA ranged from 0 to 100 μM. The inset graph shows the plot of [DNA]/(εa − εf) versus [DNA]. | |
In this experiment, upon increasing the concentration of CT-DNA (0–100 μM range) in the solution of derivatives 7aa and 7ba, hypochromic shifts were observed in the transition bands around 300–360 nm. For all derivatives, no hypso- or bathochromic shifts were observed, indicating the non-electrostatic interaction of the molecules and CT-DNA (Fig. 4). The decreased intensity of the related transition bands could be accounted for by the interaction of the aromatic portion of the compounds, probably via hydrophobic forces (van der Waals, H-bonding or π-stacking), with the DNA biomolecule or the presence of an aromatic moiety in the structure via covalent interactions, as previously reported in the literature.27
The hypochromicity parameter (H%) and intrinsic binding constant (Kb) values for the compounds were calculated and are summarized in Table 3. In the present study, the derivatives demonstrated strong binding forces to CT-DNA (Kb ∼ 105 to 106 M−1). These binding constant values are associated to the stability of the compound–DNA complex in solution, while the Gibbs free energy indicates the spontaneity of the derivative–DNA binding process (Table 3). The UV-Vis CT-DNA spectra of derivatives 7ab–ae and 7bb–be can be found in the ESI† and presented a similar behavior (Fig. S37–S44, ESI†). Moreover, molecular docking calculations between compounds and DNA were performed, reinforcing the experimental results.
Table 3 Hypochromicity (H%), bathochromic shift (Δλ), intrinsic binding constant (Kb), Stern–Volmer quenching constant (KSV), quenching rate constant (kq) and apparent binding constant (Kapp) values for the interactions of compounds with calf thymus DNA (CT-DNA)
Compound |
Absorption-DNA |
Emission EB-DNA |
H
(%) |
Δλb (nm) |
K
b
(M−1) |
ΔG° d (kcal mol−1) |
Q
(%) |
K
SV
(M−1) |
k
q
(M−1 s−1) |
K
app
(M−1) |
H(%) = (Absinitial − Absfinal)/(Absinitial) × 100.
Δλ (nm) = λfinal − λinitial.
Intrinsic binding constant by UV-Vis CT-DNA analysis.
Gibbs free energy (R = 1.987 kcal mol−1 K−1 and T = 298 K).
Q(%) = (Eminitial − Emfinal)/(Eminitial) × 100.
Stern–Volmer quenching EB-DNA constant (KSV) determined using steady-state fluorescence emission spectra.
Bimolecular Stern–Volmer quenching rate EB-DNA constant (kq) determined using steady-state fluorescence emission spectra (EB-DNA – τ0 = 23 ns).
DNA-binding apparent constant (Kapp).
|
7aa
|
8.00 |
0.0 |
1.07 × 106 |
−8.22 |
10.00 |
7.48 × 102 |
3.25 × 1010 |
6.44 × 105 |
7ab
|
7.70 |
0.0 |
4.07 × 106 |
−9.01 |
7.40 |
5.24 × 102 |
2.28 × 1010 |
6.58 × 105 |
7ac
|
8.65 |
0.0 |
1.16 × 106 |
−8.27 |
12.10 |
1.26 × 103 |
5.48 × 1010 |
6.51 × 105 |
7ad
|
8.50 |
0.0 |
6.76 × 105 |
−7.95 |
5.30 |
4.41 × 102 |
1.92 × 1010 |
6.77 × 105 |
7ae
|
11.00 |
0.0 |
2.00 × 106 |
−8.59 |
8.20 |
9.28 × 102 |
4.03 × 1010 |
6.70 × 105 |
7ba
|
10.80 |
0.0 |
4.94 × 105 |
−7.76 |
9.65 |
1.24 × 103 |
5.39 × 1010 |
6.47 × 105 |
7bb
|
7.10 |
0.0 |
2.15 × 106 |
−8.63 |
61.40 |
1.69 × 104 |
7.34 × 1011 |
4.38 × 106 |
7bc
|
11.30 |
0.0 |
1.00 × 105 |
−6.82 |
13.20 |
1.37 × 103 |
5.95 × 1010 |
6.48 × 105 |
7bd
|
9.00 |
0.0 |
1.87 × 106 |
−8.550 |
57.20 |
1.49 × 104 |
6.48 × 1011 |
4.48 × 106 |
7be
|
9.05 |
0.0 |
3.65 × 106 |
−8.945 |
69.00 |
2.50 × 104 |
1.08 × 1012 |
3.85 × 106 |
EB-DNA competitive assays through steady-state fluorescence emission analysis
In order to further confirm the binding affinity of the studied compounds with CT-DNA, competitive binding assays via fluorescence quenching analysis were conducted in the presence of the commercial intercalator (EB) into CT-DNA solution. Steady-state fluorescence emission analysis of a fixed concentration of the CT-DNA:EB adduct in the presence of derivatives 7aa and 7ba is shown in Fig. S45–S54 in the ESI,† which depict CT-DNA:EB without and in the presence of 7ab–ae and 7bb–be.
The CT-DNA
:
EB adduct formation shows a strong fluorescence emission at λ = 645 nm when excited at λexc = 510 nm and the corresponding fluorescence intensity emission decreased upon successive addition of the compounds under study, which is in accordance with some heterocyclic compounds reported in the literature.9,27 This fact could be assigned to the competition of derivatives with the intercalator EB into CT-DNA strands. The Stern–Volmer quenching constant (KSV) and bimolecular quenching rate constant (kq) values are presented in Table 3. In this context, the KSV values suggest a weak competition mode of EB-binding (KSV ∼ 102 M−1) in the case of phenyl derivatives 7aa–ae and a good competition mode of EB-binding (KSV ∼ 103–104 M−1) in the case of naphthyl derivatives 7ba–be. Moreover, the kq values for all derivatives indicated a probably static interaction (kq ∼ 1010–1011 M−1 s−1) between derivatives and the CT-DNA
:
EB adduct (see Table 3). Again, molecular docking calculations were performed, corroborating the experimental results.
HSA-binding by fluorescence quenching emission assays
HSA-binding properties can be easily determined by steady-state fluorescence emission assays through the fluorescence quenching of tryptophan (Trp) and/or tyrosine (Tyr) amino acid residues. The HSA pocket structure presents one and seventeen Trp and Tyr amino acid residues, respectively.28 Therefore, the interaction between human serum albumin and derivatives was determined through the fluorescence quenching assays.
As an example, the steady-state fluorescence emission spectra of HSA without and in the presence of successive additions of derivative 7aa (ranging from 0 to 100 μM) at room temperature (298 K) are shown in Fig. 5, while Fig. S55–S63 in the ESI† depict the spectra for the other derivatives. HSA exhibited a strong fluorescence emission peak at λem = 329 nm when excited at 290 nm. Fluorescence intensities of HSA gradually decreased upon successive additions of each compound under study, and a slight blue shift was also observed in all cases, which suggests that 7aa–ae/7ba–be interact with the albumin structure, mainly with the main fluorophores present in the biomacromolecule, and the shift may be explained by conformational changes and/or perturbations in the microenvironment around the fluorophores of the albumin upon ligand binding.
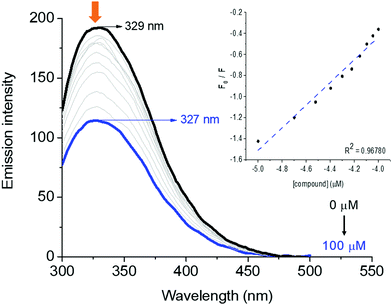 |
| Fig. 5 HSA-binding emission spectra with derivative 7aa in DMSO (2%)/Tris–HCl buffer (pH 7.2) solution. The concentration of the compound ranged from 0 to 100 μM. The inset graph shows the plot of F0/F versus [compound]. | |
The fluorescence quenching in a biomolecule can be induced by different mechanisms, which are in general classified into dynamic and static processes. In order to evaluate the main fluorescence quenching mechanism behavior induced by compounds 7aa–ae and 7ba–be, the well-known Stern–Volmer approximation was applied in this study (see the Experimental section).
The values of Stern–Volmer quenching (KSV) and bimolecular quenching rate (kq) constants for the interaction between HSA and the derivatives are shown in Table 4. In all cases, moderate KSV values (102–104 M−1) and high kq values, which are about one/two orders of magnitude larger (e.g. 1011–1012 M−1 s−1) than the diffusional collision quenching rate constant (kdiff ≈ 7.40 × 109 M−1 s−1, according to the Smoluchowski–Stokes–Einstein theory at 298 K),29 indicate that the main fluorescence quenching mechanism is a static process. Therefore, there is a ground-state association between HSA:7aa–ae and HSA:7ba–be.
Table 4 HSA-binding data of derivatives 7aa–ae and 7ba–be
Compound |
Q
(%) |
K
SV
(M−1) |
k
q
(M−1 s−1) |
K
a
(M−1) |
ΔG0 e (kcal mol−1) |
n
|
Quenching = (Intinitial − Intfinal)/Intinitial × 100.
Stern–Volmer quenching HSA constant determined using steady-state fluorescence emission spectra.
Stern–Volmer quenching rate HSA constant determined using steady-state fluorescence emission spectra (τ0 = 5.67 × 10−9 s).
Modified Stern–Volmer binding HSA constant determined using steady-state fluorescence emission spectra.
Gibbs free-energy for the HSA–molecule interaction.
Number of binding sites.
|
7aa
|
17.80 |
1.55 × 103 |
2.73 × 1011 |
1.10 × 103 |
−4.14 |
1.90 |
7ab
|
21.70 |
2.32 × 103 |
4.09 × 1011 |
1.12 × 103 |
−4.16 |
1.88 |
7ac
|
19.40 |
1.40 × 103 |
2.47 × 1011 |
1.24 × 103 |
−4.22 |
1.29 |
7ad
|
8.55 |
8.96 × 102 |
1.58 × 1011 |
1.68 × 102 |
−3.03 |
0.70 |
7ae
|
13.75 |
8.20 × 102 |
1.44 × 1011 |
2.07 × 102 |
−3.16 |
0.78 |
|
7ba
|
40.80 |
2.79 × 103 |
4.92 × 1011 |
3.87 × 103 |
−4.90 |
1.07 |
7bb
|
51.25 |
9.88 × 103 |
1.74 × 1012 |
5.20 × 103 |
−5.06 |
1.32 |
7bc
|
53.95 |
1.02 × 104 |
1.80 × 1012 |
7.50 × 103 |
−5.28 |
1.86 |
7bd
|
29.50 |
3.86 × 103 |
6.80 × 1011 |
3.33 × 103 |
−4.80 |
0.95 |
7be
|
28.45 |
3.27 × 103 |
5.76 × 1011 |
3.96 × 103 |
−4.90 |
1.12 |
The fact that the Ka values for each compound are in the order of 102–103 M−1 (Table 4) indicates moderate interaction ability. In addition, the numbers of binding sites (n) for all compounds are in the range of 0.70–1.90 (Table 4), suggesting the presence of one or two possible binding sites in the HSA subunits (depending on the ligand structure). Overall, the presence of different units (phenyl or naphthyl) in the organic ligands under study causes variation in the binding constant values and there is an indication that both derivatives 7aa–ae and 7ba–be can be possibly transported and biodistributed by serum albumin in the human bloodstream.
Molecular docking calculations for the interactions between biomacromolecules and 7aa–ae/7ba–be
Molecular docking is an attractive tool in drug design since it can evaluate biomacromolecule–drug interactions from an atomic point of view, gaining insights into the experimental results.30 Thus, in order to complement the experimental data and predict the best-fit orientation of naphthylethenyl-substituted 1,3,4-oxadiazolyl-1,2,4-oxadiazoles within DNA strands and HSA, as well as identify the main intermolecular forces involved in the interaction process, molecular docking calculations were carried out.
Table 5 shows the docking score value (dimensionless) for all synthetic compounds under study into DNA and HSA structures. For DNA, the highest docking score value was obtained in the minor groove (e.g. 71.5 and 23.2 for DNA:7aa in the minor and major grooves, respectively), suggesting that 7aa–ae and 7ba–be interact preferentially in the minor groove of DNA strands (the same theoretical region for the intercalator EB presented in this work), which is in accordance with the previous theoretical evaluation described in the literature for EB (Table 5).31 On the other hand, for HSA, the docking score values for the three main possible binding sites (sites I, II, and III in the subdomains IIA, IIIA, and IB, respectively) suggest that naphthylethenyl-substituted 1,3,4-oxadiazolyl-1,2,4-oxadiazoles could interact into sites II and III (not necessarily at the same time – docking scores are quite similar, Table 5); however, since site II presented the highest docking score value (e.g. 62.9, 84.3, and 80.3 for HSA:7aa in sites I, II, and III, respectively) all compounds bind preferentially in the subdomain IIIA. The same binding pocket in HSA was theoretically described for 1,3,4-oxadiazole derivatives of fatty acid.32
Table 5 Docking score value (dimensionless) for the interactions of DNA and HSA with derivatives 7aa–ae and 7ba–be
Compound |
DNA |
HSA |
Minor groove |
Major groove |
Site I |
Site II |
Site III |
7aa
|
71.5 |
23.2 |
62.9 |
84.3 |
80.3 |
7ab
|
75.0 |
20.1 |
65.8 |
92.9 |
85.0 |
7ac
|
76.2 |
23.0 |
67.4 |
91.6 |
86.0 |
7ad
|
76.1 |
24.5 |
65.9 |
84.8 |
79.9 |
7ae
|
78.5 |
27.8 |
73.6 |
87.4 |
84.8 |
|
7ba
|
87.1 |
31.2 |
72.7 |
93.9 |
87.4 |
7bb
|
82.2 |
30.5 |
73.1 |
92.1 |
88.1 |
7bc
|
81.3 |
30.0 |
72.0 |
92.1 |
87.3 |
7bd
|
79.4 |
29.9 |
70.6 |
91.7 |
87.6 |
7be |
85.8 |
30.8 |
76.0 |
90.9 |
90.0 |
EB
|
65.7 |
28.7 |
— |
— |
— |
Fig. 6 shows the best docking pose of 7aa–e and 7ba–be in the minor groove of DNA. From the experimental data, the intrinsic binding constant (Kb) values for compounds 7aa, 7ab, 7ac, and 7ae are in the same order (106 M−1) and interesting molecular docking calculations indicated practically the same pose inside DNA strands (superposition in Fig. 6), corroborating the experimental results. The same theoretical and experimental trend was observed for compounds 7ba–be (superposition in Fig. 6). Finally, Table S3 (ESI†) shows the main nucleobase of DNA that interacts with naphthylethenyl-substituted 1,3,4-oxadiazolyl-1,2,4-oxadiazoles, and according to the docking results van der Waals interactions are the main intermolecular forces involved in the binding process in the minor groove of DNA, also corroborating the hypothesis of intermolecular forces raised in the experimental spectroscopic data.
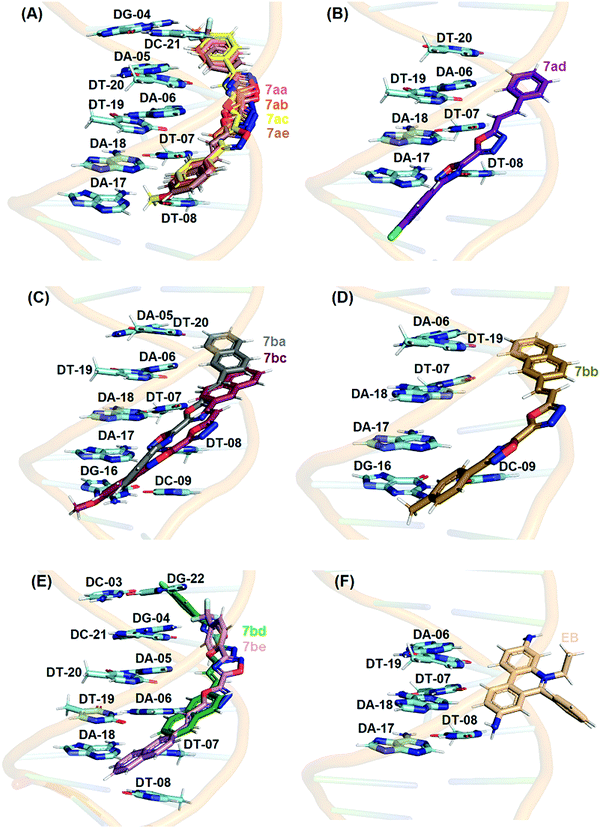 |
| Fig. 6 Best docking pose for the interactions (A) DNA:7aa–ac/ae, (B) DNA:7ad, (C) DNA:7ba/bc, (D) DNA:7bb, (E) DNA:7bd/be, and (F) DNA:EB in the minor groove. Selected nucleobases are in stick representation in cyan, while 7aa–ae, 7ba–be, and EB structures are also in stick representation, but in different colors, as shown in the figure. Color labeling of elements: hydrogen, nitrogen, chlorine, fluorine, and oxygen are in white, dark blue, light green, light blue, and red, respectively. | |
In the case of HSA studies of 7aa–ae and 7ba–be, molecular docking results suggested van der Waals and hydrogen bonding as the main intermolecular forces involved in the binding process into site II (Table S4–ESI†); as an example, the hydrogen atom from the hydroxyl group of Tyr-411 and Ser-489 residues is a potential donor for hydrogen bonding with oxygen and nitrogen atoms from the 1,3,4-oxadiazolyl moiety in the 7aa–ac structures, within a distance of 2.10 and 1.90 Å, respectively, while van der Waals interactions occur for all chemical moieties of the 7aa–ac structures with Ile-388, Lys-414, Val-415, Val-433, Cys-437, Leu-453, Leu-457, Leu-460, and Phe-488 residues within a distance of 1.30, 2.80, 2.30, 3.30, 2.50, 2.40, 3.00, 2.80, 3.60, and 1.90 Å, respectively. From the experimental data for HSA, the modified Stern–Volmer binding constant (Ka) values indicated that 7aa–ac, 7ad/ae and 7ba–be are of the same order of magnitude (103, 102, and 103 M−1, respectively) and Fig. 7 shows a clear superposition of these compounds, corroborating the spectroscopic data.
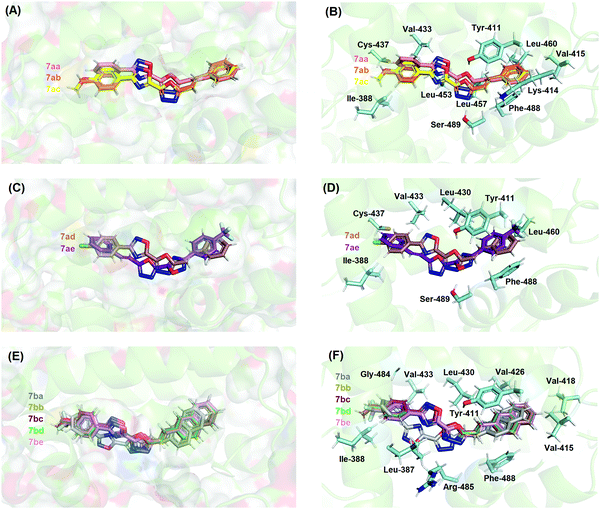 |
| Fig. 7 (A, C and E) Protein surface for the best docking pose for (A) HSA:7aa–ac, (C) HSA:7ad/ae and (E) HSA:7ba–be in site II (subdomain IIIA). (B, D and F) Amino acid residues which interact with (B) 7aa–ac, (C) 7ad/ae and (E) 7ba–be in site II. Selected amino acid residues are in stick representation in cyan, while 7aa–ae and 7ba–be structures are also in stick representation, but in different colors, as shown in the figure. Color labeling of elements: hydrogen, nitrogen, chlorine, fluorine, and oxygen are in white, dark blue, light green, light blue, and red, respectively. | |
Conclusions
The two series of 1,3,4-oxadiazolyl-1,2,4-oxadiazole derivatives were readily accessible through the employed condensation–cyclodehydration method, despite variations in the yields, and all the obtained compounds were successfully isolated and characterized. All derivatives 7aa–be displayed observable fluorescence. When phenyl and naphthyl derivatives were analyzed through UV-Vis spectroscopy, significant differences were observed between the two series. Absorbance values, which were bathochromic for naphthyl (356–360 nm) when compared to phenyl derivatives (321–325 nm), and the large Stokes shifts observed indicate a highly conjugated structure along the molecules. The strong interaction of the compounds with CT-DNA, as elucidated by UV-Vis titration, EB displacement emission analysis and theoretical molecular docking, gave a potential target for biochemical applications, which could also be favored by the moderate interaction with the important transporter protein HSA, as verified by the fluorescence-monitored titration and docking analysis. This study contributes to the understanding of 1,3,4-oxadiazolyl-1,2,4-oxadiazole as a versatile scaffold for the design of electronically conjugated molecules for physical–chemical and biochemical assays.
Authors’ contribution
J. C. Mayer, L. Dornelles and B. A. Iglesias proposed the idea for the work. J. C. Mayer conducted the synthesis and characterization analysis. D. F. Back conducted the X-ray measurements. T. V. Acunha conducted the biomolecular assays. O. A. Chaves conducted the molecular docking analysis. B. A. Iglesias, J. C. Mayer and L. Dornelles wrote the manuscript.
Conflicts of interest
There are no conflicts of interest to declare.
Acknowledgements
This research was supported by the Brazilian funding agencies: Coordenação de Aperfeiçoamento de Pessoal de Nível Superior (CAPES and CAPES/PROEX – Finance Code 001) and Conselho Nacional de Desenvolvimento Científico e Tecnológico (CNPq – Universal proc. 409150/2018-5 and PG-2018 grants process 304711/2018-7).
References
-
(a) D. S. Patel, J. R. Avalani and D. K. Raval, J. Braz. Chem. Soc., 2012, 23(10), 1951–1954 CrossRef CAS;
(b) H.-J. Li, Y.-Q. Zhang and L.-F. Tang, Tetrahedron, 2015, 71(40), 7681–7686 CrossRef CAS;
(c) Z. Wang, H. Zhang, B. J. Killian, F. Jabeen, G. G. Pillai, H. M. Berman, M. Mathelier, A. J. Sibble, J. Yeung, W. Zhou and P. J. Steel, Eur. J. Org. Chem., 2015, 5183–5188 CrossRef CAS.
-
(a) L. A. Kayukova, Pharm. Chem. J., 2005, 39(10), 539–547 CrossRef CAS;
(b) M. R. Yadav, S. T. Shirude, D. S. Puntambekar, P. J. Patel, H. B. Prajapati, A. Parmar, R. Balaraman and R. Giridhar, Acta Pharm., 2007, 57(1), 13–30 CAS.
-
(a) Z. Li, P. Zhan and X. Liu, Mini-Rev. Med. Chem., 2011, 11(13), 1130–1142 CrossRef CAS PubMed;
(b) Y. Zhang, C. Zuniga, S.-J. Kim, D. Cai, S. Barlow, S. Salman, V. Coropceanu, J.-L. Brédas, B. Kippelen and S. Marder, Chem. Mater., 2011, 23(17), 4002–4015 CrossRef CAS;
(c) J. Boström, A. Hogner, A. Llinàs, E. Wellner and A. T. Plowright, J. Med. Chem., 2012, 55(5), 1817–1830 CrossRef PubMed;
(d) N. Y. M. Abdo and M. M. Kamel, Chem. Pharm. Bull., 2015, 63(5), 369–376 CrossRef CAS PubMed.
- K. Du, X. Cao, P. Zhang and H. Zheng, Bioorg. Med. Chem. Lett., 2014, 24(12), 5318–5320 CrossRef CAS PubMed.
- M. D. Cullen, B.-L. Deng, T. L. Hartman, K. M. Watson, R. W. Buckheit, C. Pannecouque, E. De Clercq and M. Cushman, J. Med. Chem., 2007, 50(20), 4854–4867 CrossRef CAS PubMed.
- M. A. Tantray, I. Khan, H. Hamid, M. S. Alam, A. Dhulap and A. Kalam, RSC Adv., 2016, 6(49), 43345–43355 RSC.
-
(a) A. H. Frazer, W. Sweeny and F. T. Wallenberger, J. Polym. Sci., Part A: Gen. Pap., 1964, 2(3), 1157–1169 CrossRef CAS;
(b) R. Agneeswari, V. Tamilavan, M. Song, J.-W. Kang, S.-H. Jin and M. H. Hyun, J. Polym. Sci., Part A: Polym. Chem., 2013, 51(10), 2131–2141 CrossRef CAS;
(c) S. D. Ganesh, V. K. Pai, M. Y. Kariduraganavar and M. B. Jayanna, Int. Scholarly. Res. Not., 2014, ID 790702 Search PubMed.
-
(a) H. Gallardo, R. Cristiano, A. A. Vieira, R. A. W. Neves Filho, R. M. Srivastava and I. H. Bechtold, Liq. Cryst., 2008, 35(7), 857–863 CrossRef CAS;
(b) S. Chidirala, H. Ulla, A. Valaboju, M. R. Kiran, M. E. Mohanty, M. N. Satyanarayan, G. Umesh, K. Bhanuprakash and V. J. Rao, J. Org. Chem., 2016, 81(2), 603–614 CrossRef CAS PubMed.
- J. C. P. Mayer, A. C. Sauer, B. A. Iglesias, T. V. Acunha, D. F. Back, O. E. D. Rodrigues and L. Dornelles, J. Organomet. Chem., 2017, 841, 1–11 CrossRef CAS.
-
(a) C. Gao, S.-y. Liu, X. Zhang, Y.-k. Liu, C.-d. Qiao and Z.-e. Liu, Spectrochim. Acta, Part A, 2016, 156, 1–8 CrossRef CAS PubMed;
(b) K. Nozeret, F. Loll, G. M. Cardoso, C. Escudé and A. S. Boutorine, Biochimie, 2018, 149, 122–134 CrossRef CAS PubMed;
(c) F. Shamsi, B. Aneja, P. Hasan, B. Zeya, M. Zafaryab, S. H. Mehdi, M. M. A. Rizvi, R. Patel, S. Rana and M. Abid, ChemistrySelect, 2019, 4(41), 12176–12182 CrossRef CAS.
- G. M. Sheldrick, Acta Crystallogr., Sect. A: Found. Crystallogr., 2008, 64(1), 112–122 CrossRef CAS PubMed.
- L. J. Farrugia, J. Appl. Crystallogr., 1997, 30(5), 565 CrossRef CAS.
-
(a) H. G. Bonacorso, T. P. Calheiro, B. A. Iglesias, I. R. C. Berni, E. N. da Silva Júnior, J. B. T. Rocha, N. Zanatta and M. A. P. Martins, Tetrahedron Lett., 2016, 57, 5017–5021 CrossRef CAS;
(b) G. Heinrich, S. Schoof and H. Gusten, J. Photochem., 1974, 3, 315–320 CrossRef CAS.
- D. P. Heller and C. L. Greenstock, Biophys. Chem., 1994, 50, 305–312 CrossRef CAS PubMed.
- N. P. Debia, J. J. P. Rodríguez, C. H. Silveira, O. A. Chaves, B. A. Iglesias, F. S. Rodembusch and D. S. Lüdtke, J. Mol. Liq., 2020, 309, 113092 CrossRef CAS.
- R. Krüger, B. Iepsen, A. M. E. Larroza, M. G. Fronza, C. H. Silveira, A. C. Bevilacqua, M. H. Köhler, P. C. Piquini, E. J. Lenardão, L. Savegnago, B. A. Iglesias and D. Alves, Eur. J. Org. Chem., 2020, 348–361 CrossRef.
-
https://www.wavefun.com/[accessed in June 2020].
- M. Wardell, Z. Wang, J. X. Ho, J. Robert, F. Ruker, J. Ruble and D. C. Carter, Biochem. Biophys. Res. Commun., 2002, 291(4), 913–918 CrossRef PubMed.
- H. R. Drew, R. M. Wing, T. Takano, C. Broka, S. Tanaka, K. Itakura and R. E. Dickerson, Proc. Natl. Acad. Sci. U. S. A., 1981, 78(4), 2179–2183 CrossRef CAS PubMed.
-
https://www.ccdc.cam.ac.uk/solutions/csd-discovery/components/gold/[accessed in June 2020].
-
https://pymol.org/2/[accessed in June 2020].
- S. W. Leong, S. M. M. Faudzi, F. Abas, M. F. F. M. Aluwi, K. Rullah, L. K. Wai, M. N. A. Bahari, S. Ahmad, C. L. Tham, K. Shaari and N. H. Lajis, Molecules, 2014, 19(10), 16058–16081 CrossRef PubMed.
- R. M. Srivastava, M. C. Pereira, W. W. M. Faustino, K. Coutinho, J. V. Dos Anjos and S. De Melo, J. Monatsh. Chem., 2009, 140(11), 1319–1324 CrossRef CAS.
- F. Huguet, A. Melet, R. A. De Sousa, A. Lieutaud, J. Chevalier, L. Maigre, P. Deschamps, A. Tomas, N. Leulliot, J. M. Page and I. Artaud, ChemMedChem, 2012, 7(6), 1020–1030 CrossRef CAS PubMed.
- T. Huhtiniemi, T. Suuronen, V. M. Rinne, C. Wittekindt, M. Lahtela-Kakkonen, E. Jarho, E. A. A. Wallén, A. Salminen, A. Poso and J. Leppänen, J. Med. Chem., 2008, 51(15), 4377–4380 CrossRef CAS PubMed.
- P. Stabile, A. Lamonica, A. Ribecai, D. Castoldi, G. Guercio and O. Curcuruto, Tetrahedron Lett., 2010, 51, 4801–4805 CrossRef CAS.
-
(a) H. G. Bonacorso, M. B. Rodrigues, B. A. Iglesias, C. H. Silveira, S. C. Feitosa, W. C. Rosa, M. A. P. Martins, C. P. Frizzo and N. Zanatta, New J. Chem., 2018, 42(12), 10024–10035 RSC;
(b) M. B. Rodrigues, S. C. Feitosa, C. W. Wiethan, W. C. Rosa, C. H. Silveira, A. B. Pagliari, M. A. P. Martins, N. Zanatta, B. A. Iglesias and H. G. Bonacorso, J. Fluorine Chem., 2019, 221, 84–90 CrossRef CAS.
- X. Li, X. Cui, X. Yi and S. Zhong, J. Mol. Liq., 2017, 241, 577–583 CrossRef CAS.
-
M. Montalti, A. Credi, L. Prodi and M. T. Gandolfi, Handbook of Photochemistry, CRC Press, Taylor & Francis, 3rd edn, 2006 Search PubMed.
- X.-Y. Meng, H.-X. Zhang, M. Mezei and M. Cui, Curr. Comput.-Aided Drug Des., 2011, 7(2), 146–157 CrossRef CAS PubMed.
- M. A. Husain, H. M. Ishqi, T. Sarwar, S. U. Rehman and M. Tabish, Med. Chem. Commun., 2017, 8, 1283–1296 RSC.
- K. Laskar, P. Alam, R. H. Khan and A. Rauf, Eur. J. Med. Chem., 2016, 122, 72–78 CrossRef CAS PubMed.
Footnote |
† Electronic supplementary information (ESI) available. CCDC 2027432 (7aa). For ESI and crystallographic data in CIF or other electronic format see DOI: 10.1039/d0nj04530f |
|
This journal is © The Royal Society of Chemistry and the Centre National de la Recherche Scientifique 2021 |