Naturally occurring polyphenols as building blocks for supramolecular liquid crystals – substitution pattern dominates mesomorphism†
Received
23rd December 2020
, Accepted 9th March 2021
First published on 24th March 2021
Abstract
A modular supramolecular approach towards hydrogen-bonded liquid crystalline assemblies based on naturally occurring polyphenols is reported. The combination of experimental observations, crystallographic studies and semi-empirical analyses of the assemblies provides insight into the structure–property relationships of these materials. Here a direct correlation of the number of donor OH-groups as well as their orientation with the mesomorphic behavior is reported. We discovered that the number and orientation of the OH-groups have a stronger influence on the mesomorphic behavior of the supramolecular assemblies than the connectivity (e.g. stilbenoid or chalconoid) of the hydrogen bond donors. Furthermore, the photo-switching behavior of selected complexes containing azopyridine ligands was investigated. This study will help future scientists to gain a deeper understanding of the underlying mechanisms and structure–property relationships of supramolecular assemblies with mesomorphic behavior, which is still one of the major challenges in current science.
Design, System, Application
We investigated hydrogen-bonded assemblies (HBAs), consisting of naturally occurring polyphenols (HB donors) and alkylated stilbazoles (St) and azopyridines (Ap) (HB acceptors), concerning their liquid crystalline properties. To this end five different phenols, namely butein, isoliquiritigenin, resveratrol, piceatannol and oxyresveratrol, were assembled with St or Ap in all possible ratios yielding 36 HBAs, which were analyzed in detail using infrared spectroscopy, polarized optical microscopy, differential scanning calorimetry and small and wide angle X-ray scattering. We discovered mainly nematic phases for the complexes, whereas it is noteworthy that only the 1 : 3 complexes melted homogeneously leading to the assumption that this stoichiometry is favored for all complexes, which can be attributed to the substitution pattern of the phenols. Only oxyresveratrol reveals smectic behavior as a 1 : 3 complex and a nematic mesophase as a 1 : 4 complex. Additionally we discovered that the connectivity, chalconoid or stilbenoid, is less important for the mesomorphism than the substitution pattern of the phenols. This in-depth structure–property relationship of naturally occurring polyphenols will be appealing to a broader readership, since prediction of mesomorphic behavior is still challenging. We are sure that our study will help to gain a deeper understanding of the underlying principles in HBAs with liquid crystalline properties.
|
Introduction
Nature is an inexhaustible source of chemical precursors, drugs,1 and materials.2 Particularly in the context of green and sustainable chemistry, naturally occurring compounds have become the focus of recent research for the fabrication of functional materials such as polymers,3 (hydro)-gels4 and nanoparticles.5 One of the first nature-inspired examples of a well-known class of materials – liquid crystals – was found for cholesterol derivatives back in the year 1889, when Reinitzer discovered an unusual behavior for this compound showing an unknown aggregate state between liquid and crystalline at elevated temperature, which showed birefringence.6 Ever since, liquid crystalline materials have been studied intensively by research groups all over the world. In this regard, several approaches to obtain liquid crystalline materials have been investigated. The classic approach consists of the synthesis of mesogens such as biphenyls and more sophisticated molecules, containing an aromatic core unit which is functionalized with hydrocarbons or fluorocarbons.7 This synthetic approach suffers from several problems, such as long synthetic routes, expensive starting materials and inflexibility, which can be overcome by a supramolecular approach. Supramolecular chemistry provides an efficient approach towards the fabrication of functional materials by employing non-covalent interactions. Simple mixing of pre-tailored molecular building blocks provides access to a variety of functional materials by self-assembly and allows manipulation of the properties by variation of the components without the need for additional syntheses. In particular hydrogen- and halogen-bonded liquid crystals have been studied intensively by several groups in the past few years.8
Although a huge number of such systems have been reported, the complexity of the interplay of non-covalent interactions as well as their careful orchestration is still challenging and remains unpredictable over wide areas. Hence, stepwise variation of the structures remains the only way to gain deeper insights into the structure–property relationship of supramolecular liquid crystalline assemblies.
Recently Giese and co-workers established a modular approach towards hydrogen-bonded liquid crystalline materials with photo-responsive behavior by introducing alkylated azopyridine (Ap) or stilbazole (St) units as hydrogen acceptors and aromatic phenols such as phloroglucinol, resorcinol, hydroquinone and resveratrol (Res) as hydrogen donors.9 The latter, furthermore, responds to UV-light irradiation (300 nm) by undergoing photo-cyclisation to resveratrone, leading to a change in the mesophase.10 In this contribution, we expanded our scope to four additional, naturally occurring stilbenoids and chalconoids namely oxyresveratrol (Oxy), piceatannol (Pic), butein (But) and isoliquiritigenin (Iso) as hydrogen bond donors in combination with octyl-chain containing azopyridine (Ap8) and stilbazole (St8) as hydrogen bond acceptors (Fig. 1). Herein we report a systematic study on the structure–property relationship of hydrogen-bonded liquid crystals based on stilbenoids and chalconoids. Thereby, we focus on four aspects affecting the mesomorphic behavior: the role of the ratio of the hydrogen bond donor and acceptor, the influence of the hydrogen bond accepting moiety, the impact of the number and relative position of the OH-groups in the hydrogen bond donors and the role of the linking group of the hydrogen bond donating moiety.
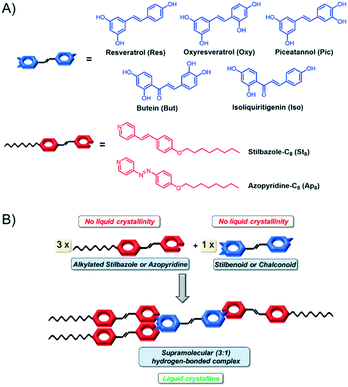 |
| Fig. 1 A) Molecular structures of the investigated stilbenoids and chalconoids (hydrogen donors, blue) as well as the alkylated stilbazoles and azopyridines (hydrogen acceptors, red). B) Schematic presentation of the modular approach towards hydrogen-bonded liquid crystalline assemblies with stilbenoids or chalconoids as core units. | |
Hydrogen-bonded mesogens were obtained by simple mixing of the acceptors and the donors, yielding a library of hydrogen-bonded assemblies, which were analyzed with respect to their mesomorphic behavior. Furthermore, computational analyses, small- and wide-angle X-ray scattering (SAXS, WAXS) and single X-ray diffraction analyses were performed to support the obtained findings for the mesomorphism.
Results and discussion
Formation of the hydrogen-bonded assemblies (HBAs)
The hydrogen-bonded assemblies (HBAs) were obtained by mixing acetonic solutions of the hydrogen bond donors (Res, Oxy, Pic, But, Iso) with the corresponding hydrogen bond acceptors (St8, Ap8, Fig. 1) in ratios of 1
:
4, 1
:
3, 1
:
2 and 1
:
1. By slow evaporation of the solvent a series of 36 hydrogen-bonded assemblies were obtained. To prove the formation of the hydrogen-bonded assemblies, IR spectroscopy was performed (ESI,† Fig. S1). Taking the Oxy(St8)3 assembly as a representative example, a shift of the OH vibration of oxyresveratrol upon formation of the HB assembly is observable. In addition to the shift of the OH band from ∼3170 cm−1 to ∼3060 cm−1, a sharpening and loss of intensity for this band can be observed. Furthermore, the band at 2650 cm−1 indicates the N–H interaction between the donor and acceptor moieties. This behavior can be observed for every HB assembly investigated in the present study (ESI,† Fig. S1). Since neither the hydrogen bond donating nor the accepting moiety shows LC properties prior to assembly formation, a further proof for the formation of the HBAs is given by the induction of liquid crystallinity. A summary of the mesogenic properties of the 1
:
3 complexes is given in Fig. 2.
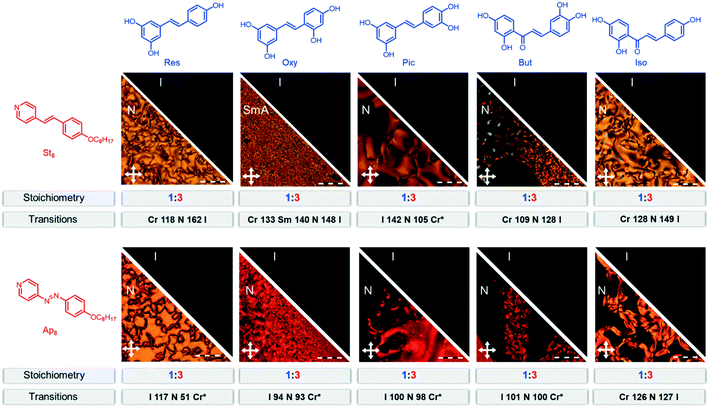 |
| Fig. 2 Summary of all hydrogen-bonded assemblies in the present study. Given are the transitions and transition temperatures as determined by POM with a heating and cooling rate of 10 °C min−1 (temperatures are given in °C). Transitions marked with * are monotropic and occur only on cooling. The scale bar represents 100 μm. The data of Res(St8)3 and Res(Ap8)3 have been reported before and are shown for comparative reasons.10 | |
Role of the ratio of the hydrogen bond donor
:
acceptor
Initial polarized optical microscopy (POM) studies revealed that complexes in the ratios of 1
:
1, 1
:
2 and 1
:
4 showed inhomogeneous melting behavior which was attributed either to free hydrogen bond donors or acceptors. For instance the POM and DSC data of the 1
:
4 mixtures of Pic and But, which contain in total four hydroxy-groups, showed two melting points, one for the 1
:
3 assembly and one additional which corresponds to the free hydrogen bond acceptors (St8 or Ap8) (Fig. S48–S63†). For the 1
:
4 complexes we additionally observed homogeneous melting for Oxy(St8)4 and Oxy(Ap8)4 (see ESI† Fig. S40 and S44). In contrast, all 1
:
3 assemblies show homogeneous melting behavior and defined phase transitions. We attribute this observation to the partial blocking of the hydrogen bond donors by inter- or intramolecular hydrogen bonding.11 Therefore, the following discussion focuses mainly on the 1
:
3 assemblies. Our hypothesis is supported by X-ray crystallography, which revealed the desired 1
:
3 complex between Pic and St8 even if a 1
:
2 ratio is used. This indicates that the formation of the 1
:
3 complex is favoured in the solid state (see the Description of the crystal structures section) which is in line with the phase separation and inhomogeneous melting of the 1
:
1 and 1
:
2 complexes. The influence of intra- and intermolecular hydrogen bonds on the complex stoichiometry will be discussed in detail in the following section (vide infra).
Influence of the hydrogen bond accepting moiety
The nature of the hydrogen bond accepting moiety, namely St or Ap, has a crucial impact on the temperature range of the mesophase and meting points of the assemblies. It is furthermore obvious that the stilbenoid complexes with Ap8 as well as the Ap8 complexes of the chalconoids show narrower temperature ranges than the comparable St8 complexes which could also be attributed to the higher tendency of Ap to crystallize (Fig. S2†). This observation is in agreement with the literature, showing that Ap is an inferior promesogen when compared to St.12 This observation can be attributed to the repulsion of the free electron pairs on the azo-linking group, destabilizing the mesophase of the corresponding assemblies.9a Since the results of the present study indicate very narrow temperature ranges for the liquid crystalline states of the Ap moieties, the following discussion will mainly focus on the results obtained for the stilbazole-based assemblies. Ap is nonetheless able to be switched from the trans to the cis state under UV-light irradiation (λex = 365 nm), which will be discussed in the “Photo-responsiveness of the HBAs” section (vide infra).
Impact of the number and relative position of the hydroxy-groups in the hydrogen bond donors
Obviously, the relative position of the hydroxy-groups plays a significant role in the mesomorphic properties. ortho-Positioned hydroxyl groups led to intramolecular hydrogen bonding, blocking one hydrogen bond donating moiety, as observed in the X-ray structure of the Pic complexes with Ap8 and St8 (vide infra). Furthermore, we found intermolecular hydrogen bonding of the remaining free OH-groups with adjacent Pic leading to the observed packing in the crystal lattice. To gain a deeper understanding of the intra- and intermolecular hydrogen bonding of the pure stilbenoids and chalconoids Pic, Iso and But which feature either ortho positioned OH-groups (Pic), a chalcone moiety (Iso) or both (But), we investigated their packing in the crystal lattice. In the crystal structure of Pic13 (CCDC 706345) (Fig. S66†) intermolecular hydrogen bonding was observed for one of the ortho positioned hydroxy-groups which is in accordance to the X-ray structure of the Ap8 and St8 complexes (vide infra). This behavior supports the preference for the 1
:
3 complexes, since one hydroxy-group participates in the packing in the crystal lattice and hence is unable to form a hydrogen bond with a hydrogen acceptor moiety. But14 (CCDC 1117309) and Iso15 (CCDC 916410), furthermore, feature intramolecular hydrogen bonds between the carbonyl-group and the adjacent hydroxy-group which can be observed in the crystal structure of the pure hydrogen bond donors, which have been described before (Fig. S67 and S68†).14,15 We noticed that, although this hydrogen bond is present in the structure of the pure compounds, the hydroxy-group participated in the HBA due to homogeneous melting of the Iso 1
:
3 complexes. Furthermore, the observations of Pic concerning ortho-positioned OH groups also apply for But, since homogeneous melting was only observed for the 1
:
3 complex and not for the 1
:
4 assemblies.
Role of the linking group of hydrogen bond donors
In this paragraph we aim for a direct comparison of the used stilbenoids to gain a deeper understanding of the structure–property relationship. The stilbenoids Res, Oxy and Pic differ exclusively in their hydroxyl substitution pattern on the second benzene unit whereas the other aromatic unit features two hydroxyl-groups in the meta position (Fig. 1). As already reported, Res(St8/Ap8) showed a nematic liquid crystalline phase with the typical “Schlieren” texture (Fig. 3 and ESI† Fig. S5 and S6).10 Here it was found that Pic is comparable with Res since only nematic structures were found for the 1
:
3 complexes with St8 and Ap8 (see Fig. 2 and 3).
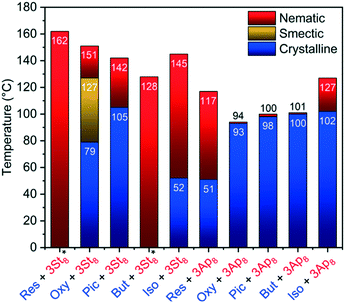 |
| Fig. 3 Summary of the LC properties of the 1 : 3 complexes reported in the present study, measured on cooling with a rate of 10 °C min−1 under a POM. Clearing points are reported and complexes marked with * undergo a transition into a glassy state, preserving the texture of the liquid crystalline phase (temperatures are given in °C). The data of Res(St8)3 and Res(Ap8)3 have been reported before and are shown for comparative reasons.10 | |
Comparing the Pic(Ap8)3 assembly with the corresponding Res(Ap8)3 assembly reveals a monotropic and very narrow temperature range of 2 °C of the nematic phase with simultaneous crystallization. Also in the case of the Pic(St8)3 assembly a drastic decrease in the temperature range of the mesophase was observed. In both cases the narrow temperature range of the mesophase is related to a significantly increased crystallization temperature. These findings were attributed to the fact that one vicinal OH group remains free and thus, does not bind to the hydrogen bond acceptor. However, in the densely packed crystalline phase, this free OH group interacts with neighbouring assemblies promoting the crystallization of the assemblies.13 This assumption is supported by single crystal X-ray diffraction analyses. For all donor
:
acceptor ratios (e.g. 1
:
3 and 1
:
4) only suitable crystals of the 1
:
3 complexes were obtained (see description of the crystal structures subchapter, vide infra). Interestingly, only Oxy(St8)3 shows a transition from the nematic (ΔTN = 23 °C) into a smectic phase (ΔTSmA = 48 °C). Upon cooling of the Oxy(St8)3 complex, first the “Schlieren” texture is observed, which undergoes a transition into a pseudo focal-conic texture (Fig. 2 and 3 and ESI† Fig. S9). The transition from the nematic to a smectic phase was confirmed by small angle X-ray scattering (SAXS) (Fig. 4 and S64 and S65†). Analysis of the radial averaged scattering patterns on the smectic mesophase reveals a periodicity of 4.3 nm and 0.45 nm for the long and short axis, respectively. The temperature range, in which the liquid-crystalline phase appears, is also significantly higher for Oxy (ΔT = 72 °C for St8) than for Pic (Fig. 2 and 3), which we attribute to the missing interference of intra- versus intermolecular hydrogen bonding for the Oxy-based assemblies. The 1
:
4 complexes of Oxy with Ap and St revealed only nematic behavior. To sum up, all three St8 complexes reveal mesomorphism over a broad temperature range revealing nematic (Res and Pic) or smectic (Oxy) phases.
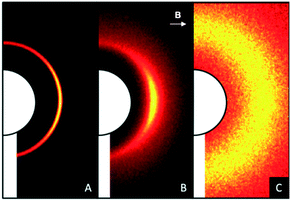 |
| Fig. 4 SAXS patterns of Oxy(St8)3 in a smectic (A), nematic (B) and isotropic (C) phase. The nematic phase (B) was oriented by a horizontal magnetic field B (arrow). | |
Besides stilbenoids, the liquid crystalline properties of the chalconoid HBAs of But and Iso were investigated, showing the characteristic “Schlieren” texture of a nematic phase for all assemblies. But and Iso reveal the same substitution pattern of the hydroxyl-groups like Pic and Res respectively, but differ in the linkage between the two arene moieties featuring a carbonyl group (chalcone backbone). It is noticeable that Iso(St8)3 forms an enantiotropic mesophase with a broad temperature range upon cooling (ΔTN = 93 °C), whereas But(St8)3 shows monotropic phase behavior with the formation of a glassy state preserving the nematic mesophase (Fig. 3 and ESI† Fig. S21–S27). The additional free vicinal hydroxy-group of But seems to broaden the temperature range of the liquid crystalline state in the St-complex and thus prevents the crystallization on cooling. However, if the Ap complexes of the two compounds are considered, the Iso-complex has a much broader temperature range of the nematic phase as its substitution pattern is comparable with that of Res, featuring a single hydroxy-group para-oriented to the double bond bridge.
Photo-responsiveness of the HBAs
If azopyridine is used as the hydrogen bonding acceptor, it allows for reversible photo-switching.9a Due to irradiation with UV-light (405 nm) reversible trans–cis isomerization is induced, which leads to a structural change, followed by a transition from the nematic to the isotropic state. Irradiating the complex Iso(Ap8)3 in its mesophase at 110 °C, using a commercially available laser pointer (5 mW) with a wavelength of 405 nm, a transition from the nematic to the isotropic state can be induced (Fig. 5). Before irradiating the sample the typical “Schlieren” texture of the nematic phase can be observed. After irradiation, with a wavelength of 405 nm, this texture vanishes and no birefringence is present, indicating the isotropic state. This transition can be attributed to the reversible trans–cis switching of the azo-compounds, which changes the shape of the molecule and therefore disturbs the orientation of the calamitic liquid crystals due to the bent shape.
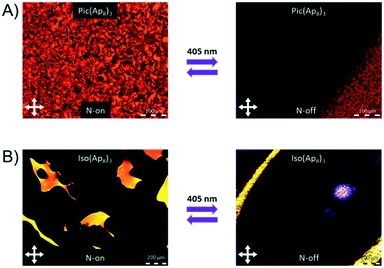 |
| Fig. 5 POM images showing the photo-switchability of the nematic phase of A) Pic(Ap8)3 at 105 °C and B) Iso(Ap8)3 at 110 °C with a wavelength of 405 nm. | |
This photo-switching is reversible and after removal of the UV light the “Schlieren” texture reappears within less than a second, which was reproduced for several cycles. A similar behavior was observed for Pic(Ap8)3 (Fig. 5) showing that this photo-isomerization is independent from the connectivity of the HB donors using either stilbenoids or chalconoids.
Description of the crystal structures
For two hydrogen-bonded assemblies the formation of the assembly was additionally confirmed by X-ray diffraction analysis of single crystals. The complex Pic(Ap8)3 crystallizes in the centrosymmetric space group P21/n with four molecules in the unit cell. The solid-state structure clearly shows the formation of hydrogen bonds between Pic and Ap8 (OH⋯Npy = 1.823, 1.912, 1.935 Å, O⋯Npy = 2.722, 2.761 and 2.755 Å, Fig. 6).
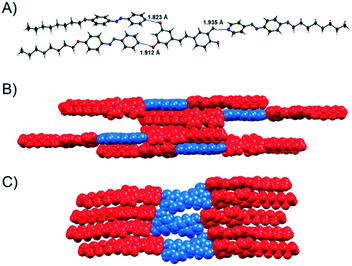 |
| Fig. 6 A) X-ray structure of the 3 : 1 Pic(Ap8)3 assembly; B) space filling model of the packing in side view and C) in top view. Colored in red: azopyridine (Ap8), colored in blue: piceatannol (Pic). | |
Pic was found to be disordered over two positions in the asymmetric unit. In the packing diagram a linear chain along the c-axis involving auxiliary hydrogen bond interactions between two adjacent piceatannol molecules (OH⋯O = 1.950 Å) and π⋯π interactions between pyridine–pyridine rings (3.348 Å; 3.352 Å) and phenyl–phenyl rings (3.375 Å, 3.388 Å) was formed (Fig. 6C). The 3D network is furthermore supported by numerous additional CH⋯π and π⋯π interactions. Replacing the azopyridine acceptor unit with St8 yielded the related solid state structure of Pic(St8)3. The three hydrogen bonds between the hydroxy-groups of the piceatannol and the pyridine nitrogen atom of stibazole-C8 are OH⋯Npy = 1.769, 1.811, and 1.831 Å (O⋯Npy = 2.691, 2.758 and 2.696 Å, Fig. 7A), respectively. These hydrogen bonds are slightly shorter compared to those found in the complex Pic(Ap8)3. In contrast to the linear chain found in the packing diagram of Pic(Ap8)3, which presents an alternating orientation of the Pic moiety, the solid state packing of Pic(St8)3 shows a parallel orientation of the Pic molecules, which are shifted with respect to each other (see Fig. 7C). Only OH⋯O (1.903 Å) and two types of CH⋯π interactions between pyridine–pyridine rings (2.898 Å) and pyridine–phenyl rings (2.826 Å) are involved in the formation of these chains (Fig. 7C). Additional CH⋯π and π⋯π interactions complete the 3D network. The parallel orientation of Pic(St8)3 is obviously beneficial for the formation of mesophases with a larger temperature range (see Fig. 2), whereas the linear orientation of Pic(Ap8)3 promotes faster crystallization.
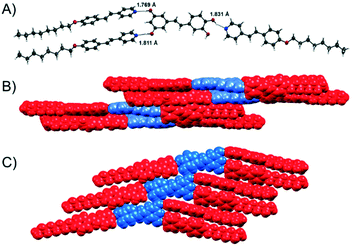 |
| Fig. 7 A) X-ray structure of the 3 : 1 supramolecular hydrogen-bonded Pic(St8)3 complex; B) space filling model of the packing in side view and C) in top view. Colored in red: stilbazole (St8), colored in blue: piceatannol (Pic). | |
Theoretical analysis of the crystal packing
To further understand the underlying mesogenic properties of the hydrogen-bonded assemblies, a packing analysis was performed to quantify the intramolecular interactions of the packing in the solid state. This packing cannot be directly correlated to the packing of the molecules in the mesogenic state, but can give, nonetheless, some insight into the predominant non-covalent interactions and therefore the liquid crystalline state.
The geometrical description of the crystal structures used above, in which we identified short intermolecular contacts and described them as contributors to the overall crystal packing, is common and easy to understand. However, this description gives only an incomplete picture of the factors that govern the crystal packing and it is of little help to quantify the intermolecular interactions in our structures. We thus used a methodology which is based on the intermolecular perturbation theory developed by Spackman,16 which is in turn based on the work of Gavezzotti,17 and implemented in the CrystalExplorer program.18 This methodology allows for direct and accurate quantification of the intermolecular interactions and recently provided us insight into the aggregation-induced-emission (AIE) behavior of a series of liquid crystalline aromatic thioethers,19 and explanation of subtle differences in the LC behavior of supramolecular liquid crystals based on natural products. Details on the methodology are given in the ESI.† In order to analyze the non-covalent interactions in the solid state a reference molecule in the crystal structure was defined (see Fig. 8 and 9 for details) and molecular pairs (structure determinants) with surrounding molecules were investigated with respect to their intermolecular interactions. In this respect, we selected the six most significant pairs (Fig. 8 and 9). In each structure determinant, the interaction energies are calculated and split into the individual contributions of electrostatics, polarization, dispersion and repulsion (ESI† Tables S2 and S3, for each individual contribution). Our structures are both disordered and we modelled them in the approximation that we are dealing with static disorder, so the disordered molecule can be in one conformation – or the other – in different cells. We present here the results for the most populated conformer of each of the two structures, but the conclusions do not change if the other two (less populated) conformers are considered. The main contributors to the crystal packing of this assembly are the two OH⋯Npy hydrogen bonds, which are formed by two Ap8 molecules and the “resorcinol” ring of the Pic molecule (44.9 and 44.2 kJ mol−1, Fig. 8, structure determinants 1 and 2).
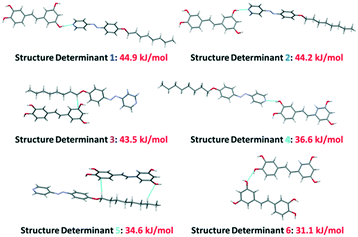 |
| Fig. 8 Supramolecular hierarchy of the six most stabilizing interactions in the Pic(Ap8)3 structure as provided by CrystalExplorer. Blue lines represent short intermolecular contacts below the sum of the van der Waals radii of the respective atoms. Colour chart: grey = C, white = H, red = O, and purple = N. | |
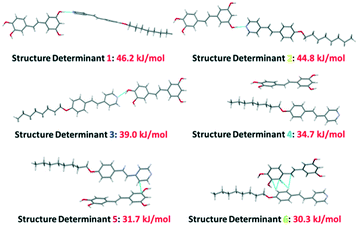 |
| Fig. 9 Supramolecular hierarchy of the six most stabilizing interactions in the Pic(St8)3 structure as provided by CrystalExplorer. Blue lines represent short intermolecular contacts below the sum of the van der Waals radii of the respective atoms. Colour chart: grey = C, white = H, red = O, and purple = N. | |
The third OH⋯Npy hydrogen bond ranks fourth in the interaction hierarchy with a reduced interaction energy (36.6 kJ mol−1, Fig. 8, structure determinant 4) compared to the other two. We attributed this fact to the lower acidity of the hydroxy-protons in the “catechol” ring compared to those in the resorcinol ring of the Pic molecule.20 Furthermore, due to the steric repulsion of the two vicinal OH groups, the weakest OH⋯Npy hydrogen bond has a slightly distorted geometry with an O–H⋯N angle of 159°. This angle could be compared with the reference O–H⋯N angle of 176° present in the “ideal” phenol–pyridine complex.21 Interestingly, the remaining hydroxy-group, which does not interact with the pyridine rings of the Ap8 molecules, is involved in hydrogen bonding with another hydroxy-group of a neighbouring Pic molecule (Fig. 8, structure determinant 6).
The hydrogen bonds of structure determinants 1, 2, 4 and 6 are dominated by electrostatics (Fig. 8 and 9 and ESI† Tables S2 and S3), as expected. Important contributions to the crystal packing are also given by structure determinants 3 and 5, which feature CHalk⋯π interactions that are dispersive in nature (Fig. 8 and 9).
Similarly to the structure previously described, the OH⋯Npy hydrogen bonding encompassing structure determinants 1–3 dominates the overall interaction ranking. There are however important differences between the packing of Pic(St8)3 (Fig. 9) and the packing of Pic(Ap8)3 (Fig. 8), due to the fact that, in the present case, π⋯π edge-to-face and stacked interactions are much more important energetically (structure determinants 4–6). Although the packing of Pic(St8)3 (even that of any assembly) in the solid state cannot be directly compared with that in the LC state, the increased relevance of π⋯π and stacked interactions is important to promote the LC behavior, as the LC phase might be suppressed, when these interactions are hindered or disrupted.22
Conclusions
In conclusion, we reported liquid crystalline assemblies based on three different stilbenoids and two chalconoids formed from hydrogen bonds with alkylated stilbazoles or azopyridines which were found to be photoresponsive. The obtained single crystals also proved the formation of the hydrogen-bonded assemblies and allowed us to quantify the intramolecular interactions. While we observe mainly nematic phases for the HBAs, the Oxy(St8)3 complex also showed a smectic liquid crystalline phase, as confirmed by SAXS and WAXS measurements. Furthermore, it was found that Res(Ap8)3 and Iso(Ap8)3 complexes reveal similar mesomorphic behavior, which can be attributed to their identical hydroxy-substitution pattern leading to comparable supramolecular assemblies despite their connectivity. As a result of these observations, it is suggested that the type of connectivity in the HB donors (e.g. with or without additional carbonyl-groups) of the phenolic units is less important than the number and position of the hydroxy-groups, which is remarkable since intramolecular hydrogen bonds in the chalconoids are present and need to be broken upon HBA formation. This observation supports the current understanding that the prediction of properties such as the kinds and range of mesophases as well as melting points and transition temperatures remains a challenging task. We expect for future experimental studies that naturally occurring polyphenolic compounds will lead to a plethora of novel liquid-crystalline complexes with different physical and chemical properties, helping to predict mesomorphism of supramolecular hydrogen-bonded complexes.
Conflicts of interest
There are no conflicts to declare.
Acknowledgements
Jun.-Prof. Jens Voskuhl acknowledges the Center for Nanointegration (CENIDE) for financial support. Jun.-Prof. Michael Giese is thankful for generous financial support from the Professor Werdelmann Foundation. Ass. Prof. Marco Saccone acknowledges PON R&I 2014–2020 – AIM (Attraction and International Mobility), project AIM 1813040 for financial support.
Notes and references
- T. Beghyn, R. Deprez-Poulain, N. Willand, B. Folleas and B. Deprez, Chem. Biol. Drug Des., 2008, 72, 3–15 CrossRef PubMed.
- Y. Liu, K. He, G. Chen, W. R. Leow and X. Chen, Chem. Rev., 2017, 117, 12893–12941 CrossRef PubMed.
- A. Kashirina, Y. Yao, Y. Liu and J. Leng, Biomater. Sci., 2019, 7, 3961–3983 RSC.
-
(a) K. Rajan, J. K. Mann, E. English, D. P. Harper, D. J. Carrier, T. G. Rials, N. Labbé and S. C. Chmely, Biomacromolecules, 2018, 19, 2665–2672 CrossRef PubMed;
(b) K. J. De France, T. Hoare and E. D. Cranston, Chem. Mater., 2017, 29, 4609–4631 CrossRef.
- G. Jutz and A. Böker, Polymer, 2011, 52, 211–232 CrossRef.
- M. Mitov, ChemPhysChem, 2014, 15, 1245–1250 CrossRef PubMed.
- P. Kirsch and M. Bremer, Angew. Chem., Int. Ed., 2000, 39, 4216–4235 CrossRef PubMed.
-
(a) H. Wang, H. K. Bisoyi, L. Wang, A. M. Urbas, T. J. Bunning and Q. Li, Angew. Chem., Int. Ed., 2018, 57, 1627–1631 CrossRef PubMed;
(b) M. Saccone, M. Spengler, M. Pfletscher, K. Kuntze, M. Virkki, C. Wölper, R. Gehrke, G. Jansen, P. Metrangolo, A. Priimagi and M. Giese, Chem. Mater., 2019, 31, 462–470 CrossRef.
-
(a) M. Pfletscher, C. Wölper, J. S. Gutmann, M. Mezger and M. Giese, Chem. Commun., 2016, 52, 8549–8552 RSC;
(b) M. Pfletscher, S. Hölscher, C. Wölper, M. Mezger and M. Giese, Chem. Mater., 2017, 29, 8462–8471 CrossRef;
(c) M. Saccone, K. Kuntze, Z. Ahmed, A. Siiskonen, M. Giese and A. Priimagi, J. Mater. Chem. C, 2018, 6, 9958–9963 RSC;
(d) R. Y. Dong, C. A. Michal, M. Saccone, M. Spengler, C. Wölper and M. Giese, Chem. Phys. Lett., 2018, 710, 39–44 CrossRef;
(e) M. Saccone, M. Pfletscher, E. Dautzenberg, R. Y. Dong, C. A. Michal and M. Giese, J. Mater. Chem. C, 2019, 7, 3150–3153 RSC.
- M. Blanke, J. Balszuweit, M. Saccone, C. Wölper, D. Doblas Jiménez, M. Mezger, J. Voskuhl and M. Giese, Chem. Commun., 2020, 56, 1105–1108 RSC.
- M. Saccone, M. Pfletscher, S. Kather, C. Wölper, C. Daniliuc, M. Mezger and M. Giese, J. Mater. Chem. C, 2019, 7, 8643–8648 RSC.
-
(a) Y. Chen, H. Yu, L. Zhang, H. Yang and Y. Lu, Chem. Commun., 2014, 50, 9647–9649 RSC;
(b) L. J. McAllister, C. Präsang, J. P. W. Wong, R. J. Thatcher, A. C. Whitwood, B. Donnio, P. O'Brien, P. B. Karadakov and D. W. Bruce, Chem. Commun., 2013, 49, 3946–3948 RSC;
(c) M. Pfletscher, M. Mezger and M. Giese, Soft Matter, 2018, 14, 6214–6221 RSC.
- M. Rossi, F. Caruso, C. Opazo and J. Salciccioli, J. Agric. Food Chem., 2008, 56, 10557–10566 CrossRef PubMed.
- S. Norio, U. Katsuhiko and S. Yoshio, Bull. Chem. Soc. Jpn., 1972, 45, 2274–2277 CrossRef.
- M. Rossi, F. Caruso, E. J. Crespi, J. Z. Pedersen, G. Nakano, M. Duong, C. McKee, S. Lee, M. Jiwrajka, C. Caldwell, F. Baffour, D. A. Karlin, G. Lidoff, S. Leone, V. Balducci, J. Miler and S. Incerpi, Biochimie, 2013, 95, 1954–1963 CrossRef PubMed.
- M. J. Turner, S. Grabowsky, D. Jayatilaka and M. A. Spackman, J. Phys. Chem. Lett., 2014, 5, 4249–4255 CrossRef PubMed.
-
(a) A. Gavezzotti, J. Phys. Chem. B, 2002, 106, 4145–4154 CrossRef;
(b) A. Gavezzotti, J. Phys. Chem. B, 2003, 107, 2344–2353 CrossRef.
-
J. J. M. M. J. Turner, S. K. Wolff, D. J. Grimwood, P. R. Spackman, D. Jayatilaka and M. A. Spackman, CrystalExplorer17 (2017), University of Western Australia, 2017, http://hirshfeldsurface.net Search PubMed.
-
(a) S. Riebe, M. Saccone, J. Stelzer, A. Sowa, C. Wölper, K. Soloviova, C. A. Strassert, M. Giese and J. Voskuhl, Chem. – Asian J., 2019, 14, 814–820 CrossRef PubMed;
(b) M. Saccone, S. Riebe, J. Stelzer, C. Wölper, C. G. Daniliuc, J. Voskuhl and M. Giese, CrystEngComm, 2019, 21, 3097–3105 RSC;
(c) M. Saccone, M. Blanke, C. G. Daniliuc, H. Rekola, J. Stelzer, A. Priimagi, J. Voskuhl and M. Giese, ACS Mater. Lett., 2019, 1, 589–593 CrossRef.
-
E. P. Serjeant and B. Dempsey, Ionisation Constants of Organic Acids in Aqueous Solution, Pergamon Press, 1979, p. 161 Search PubMed.
- T. Steiner, I. Majerz and C. C. Wilson, Angew. Chem., Int. Ed., 2001, 40, 2651–2654 CrossRef PubMed.
-
J. W. Steed and P. A. Gale, Liquid crystals formed from specific supramolecular interactions, John Wiley & Sons, Ltd, New York, 2012, pp. 3493–3515 Search PubMed.
Footnotes |
† Electronic supplementary information (ESI) available: Additional DSC, POM and IR measurements as well as information on the Hirshfeld calculations. CCDC Pic(Ap8)3 (1945777) and Pic(St8)3 (1942435). For ESI and crystallographic data in CIF or other electronic format see DOI: 10.1039/d0me00171f |
‡ The two authors contributed equally to this work. |
|
This journal is © The Royal Society of Chemistry 2021 |