DOI:
10.1039/D1MD00163A
(Research Article)
RSC Med. Chem., 2021,
12, 2053-2059
ItaCORMs: conjugation with a CO-releasing unit greatly enhances the anti-inflammatory activity of itaconates†
Received
8th May 2021
, Accepted 11th October 2021
First published on 15th October 2021
Abstract
Endogenous itaconate as well as the gasotransmitter CO have recently been described as powerful anti-inflammatory and immunomodulating agents. However, each of the two agents comes along with a major drawback: Whereas itaconates only exert beneficial effects at high concentrations above 100 μM, the uncontrolled application of CO has strong toxic effects. To solve these problems, we designed hybrid prodrugs, i.e. itaconates that are conjugated with an esterase-triggered CO-releasing acyloxycyclohexadiene–Fe(CO)3 unit (ItaCORMs). Here, we describe the synthesis of different ItaCORMs and demonstrate their anti-inflammatory potency in cellular assays of primary murine immune cells in the low μmolar range (<10 μM). Thus, ItaCORMs represent a promising new class of hybrid compounds with high clinical potential as anti-inflammatory agents.
Introduction
Dimethyl fumarate (DMF; 1) represents one of the structurally simplest active pharmaceutical ingredients. Since its accidental discovery, it has become one of the most frequently used immunomodulatory and anti-inflammatory agents in the clinics, especially for the treatment of psoriasis and multiple sclerosis.1 As an electrophilic molecule DMF was shown to covalently modify cysteine residues, thereby inactivating proteins such as the glycolytic enzyme glyceraldehyde 3-phosphate dehydrogenase (GAPDH) and causing down-regulation of aerobic glycolysis. Another relevant target of DMF is the Kelch-like ECH-associated protein 1 (KEAP1) which is involved in inflammatory processes by suppressing the (erythroid-derived 2)-related factor 2 (Nrf2).2
The endogenous metabolite itaconic acid (IA; 2) has recently been identified as an even stronger anti-inflammatory and immunoregulatory agent3 also modulating macrophage function by activating Nrf2 via alkylation of cysteine residues of KEAP1. Moreover, the cell-permeable derivative 4-octyl itaconate (4-OI; 3) proved to be a strong protective agent against lipopolysaccharide (LPS) induced lethality of human peripheral blood mononuclear cells (PBMCs) in vivo by reducing cytokine production.4 In another study, the extensive metabolic rewiring of inflammatory macrophages, including regulation of the IκBζ–ATF3 inflammatory axis, was shown to be linked to the induction of electrophilic stress caused by IA and even stronger by its membrane-permeable derivative dimethyl itaconate (DMI; 4) by selectively inhibiting a subset of cytokines, including IL-6 and IL-12.5 These effects were confirmed in subsequent studies, and the promising potential of itaconate-derived compounds as therapeutic agents is reflected by intense ongoing research activities in leading laboratories.6
Another most simple anti-inflammatory, immunomodulating and cytoprotective molecule is carbon monoxide (CO), a so-called gasotransmitter which, like NO, displays highly beneficial effects at very low concentrations.7 To avoid the risks and toxicity associated with administration of CO by inhalation, the development of methods for the targeted delivery of CO, particularly through the use of CO-releasing molecules (CORMs), has become a fascinating field of drug discovery.8 While a variety of CORMs have been reported in the literature, most of them do not meet the requirements for pharmaceutical application. A promising concept to meet the challenge of controlled (intracellular) CO release is the use of enzyme-triggered CORMs (ET-CORMs).9 In this context, we introduced acyloxydiene–Fe(CO)3 complexes activated by enzyme-induced hydrolysis of the ester moiety.10 In contrast to the stable ester prodrugs, the resulting dienol–Fe(CO)3 complexes, such as rac-5, undergo rapid oxidation under physiological conditions, leading to the release of up to three molecules of CO causing the expected pronounced biological effects (Scheme 1). Noteworthy, simultaneously formed cyclohexenone (6) and ferric ions (Fe3+) were found not to affect cell viability at the relevant concentrations.11 Another by-product of the enzymatic ester cleavage of ET-CORMs is a carboxylic acid corresponding to the acyloxy unit. While first studies on our esterase-triggered system were conducted only with biologically non-active acid residues, such as acetate or homologous alkanoic acids, we were aware that the ET-CORM concept ideally allows a bioactive acid to be concomitantly released together with CO which could act synergistically. Hence, methyl fumarate-derived ET-CORMs (FumET-CORMs) were presented as one of the first bifunctional CORMs which exhibited a substantially improved anti-inflammatory effect as compared to the clinically used drug DMF (1).12 In a somewhat related fashion, (untriggered) hybrid CORMs, derived from fumarates and Co, Mn or Ru carbonyl complex units have been reported by Motterlini and coworkers.13
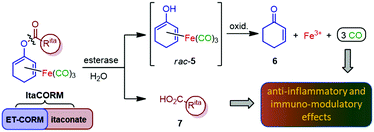 |
| Scheme 1 Design of anti-inflammatory compounds (ItaCORMs) acting by intracellular esterase-triggered release of both carbon monoxide (CO) and an itaconate (7). | |
Inspired by the promising properties of the FumET-CORMs and taking into account the significantly higher bioactivity of DMI (4)3–5 compared to DMF (1), we now envisioned to synthesize and investigate itaconate-derived ET-CORMs (ItaCORMs) which we expected to exhibit even more powerful anti-inflammatory effects (Scheme 1).
Results
Synthesis of ItaCORMs
According to our previously established procedure, ItaCORMs were prepared from the cyclohexenone-derived silyl dienol ethers rac-9a or rac-9b, respectively (Scheme 2).9c For this purpose, the silyl group of rac-9a/b was first cleaved off with TBAF in THF and the resulting iron-complexed dienolate alkoxides were reacted with the respective itaconate-derived acid chlorides prepared in situ using Ghosez's reagent (10).14 The required mono-alkyl itaconates were either commercially obtained (12a/12b) or synthesized (11a/11b) from itaconic acid following a modified literature protocol.15 These compounds were also employed as controls in the biological investigations (vide infra). As an additional reference substance, the known acetic acid-derived ET-CORM rac-8 was prepared as previously described.10
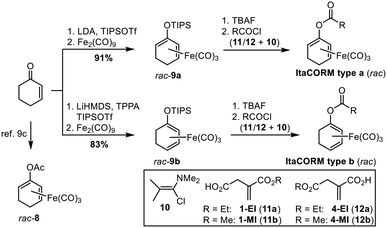 |
| Scheme 2 Synthesis of various itaconate-derived acyloxycyclohexadiene–Fe(CO)3 complexes as potential CO-releasing molecules (ItaCORMs). LDA = lithium diisopropylamide; TIPSOTf = triisopropylsilyl trifluoromethanesulfonate; TPPA = tripyrolidinophosphoric acid triamide; TBAF = tetrabutylammonium fluoride. | |
According to the general protocol (Scheme 2), a set of eight (racemic) ItaCORMs were prepared in good to excellent yield (Fig. 2). Noteworthy, initial attempts to perform the esterification of the intermediate dienolate–Fe(CO)3 complexes under Steglich-type conditions (previously used to prepare fumarate-derived CORMs)12 completely failed in the itaconate series.
The prepared (racemic) ItaCORMs were all characterized by the common spectroscopic methods and proved to be stable and easy to handle compounds, which can be stored for longer periods of time in the fridge without decomposition. The structures of the methyl ester derivatives ItaCORM3b and ItaCORM4b were additionally confirmed by single crystal X-ray analysis. (Fig. 3).
In vitro CO-release
To probe the proposed ability of the ItaCORMs to undergo enzyme-triggered cleavage/oxidation under concomitant carbon monoxide (CO) release (Scheme 1) samples of the complexes were treated with pig liver esterase (PLE) in a DMSO/phosphate buffer mixture, and the CO release was monitored over a period of 100 h by means of headspace gas chromatography. While virtually no CO (≤0.05 equiv.) was released in the absence of PLE (control) we found that significant amounts of CO were liberated from the ItaCORMs in the presence of PLE (Fig. 4). Interestingly, but not completely unexpected,10,12 the ItaCORMs of type a, with the acyloxy substituent at the “inner” position of the cyclohexadiene–Fe(CO)3 moiety (Fig. 4A), showed a considerably slower CO release in comparison to the “outer” series (Fig. 4B). We also found that ItaCORMs4a and 4b, derived from 4-methyl itaconate (12b) displayed a stronger tendency to release CO than the isomers derived from 1-methyl itaconate (11b). In this particular assay, the “best” CO-releasing molecule of the investigated compounds proved to be ItaCORM4b, which released three full equivalents of CO within 17 hours (Fig. 4B). We would like to emphasize, however, that these in vitro-measured CO-release kinetics reflect the sum of several steps including of the ester cleavage and the oxidation of the primary dienol–Fe(CO)3 complexes of type rac-5 (Scheme 1).
Biological studies
To investigate the expected anti-inflammatory activity of ItaCORMs we employed an established in vitro model for the analysis of inflammatory responses utilizing bone marrow-derived dendritic cells (BMDCs) stimulated by lipopolysaccharide (LPS).12,16 At first, we determined the cytotoxicity of ItaCORMs1a/b and 2a/2b by means of the XTT assay,12 and an assay based on the release of lactate dehydrogenase (LDH).17 While these compounds showed no substantial toxicity up to a concentration of 15 μM, a rapid decrease of the cell viability was observed at concentrations of ≥25 μM for all ItaCORMs with the exception of ItaCORM1a (see Fig. SI-9†). Therefore, all subsequent experiments were performed using non-toxic concentrations ≤15 μM.
As previously shown, the envisaged induction of an anti-inflammatory type II phenotype of dendritic cells importantly depends both on the regulation of HO-1 and STAT1 signaling pathways and on the associated inhibition of inflammatory cytokines IL-23 and IL12-p70.12 Hence, we next analyzed the effects of ItaCORMs on these signaling mechanisms (Fig. 5). And indeed, HO-1 was strongly upregulated upon treatment of the cells with ItaCORMs1b, 2a and 2b, in contrast to STAT1, which remained more or less unchanged. Noteworthy, the effects of these dual-active compounds proved to be much stronger than those of the control substances 1-EI (11a), 4-EI (12a) and rac-8, respectively, which only release either itaconic acid or CO (Fig. 1).
 |
| Fig. 1 Dimethyl fumarate and itaconic acid derivatives displaying pronounced anti-inflammatory effects. | |
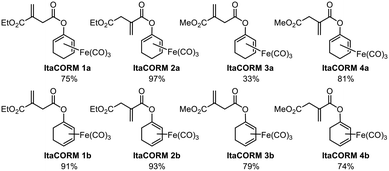 |
| Fig. 2 Itaconate-derived CO-releasing molecules (ItaCORMs) synthesized according to Scheme 2. | |
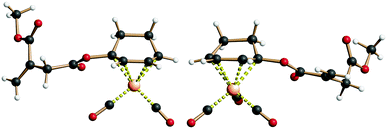 |
| Fig. 3 Structure of ItaCORM3b (left) and ItaCORM4b (right) in the crystalline state. | |
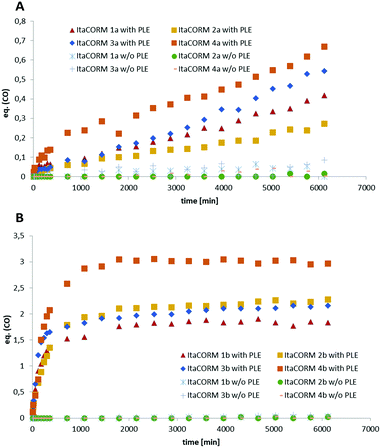 |
| Fig. 4 Quantification of the PLE-triggered CO release properties of ItaCORMs. A: ItaCORMs of type a. B: ItaCORMs of type b. Each complex (36 μmol) was dissolved in 0.2 mL of DMSO and 1.0 mL of phosphate buffer (pH 7.4) before 15 mg of PLE (≥24 units per mg) were added. The resulting mixture was stirred at 37 °C and the released CO was measured via headspace gas chromatography. Samples of each ItaCORM in the absence of PLE were analyzed in via the same method as a control. | |
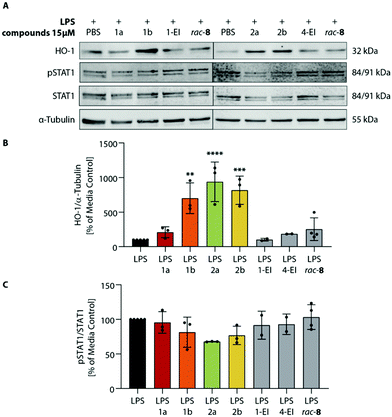 |
| Fig. 5 Modulation of HO-1 and STAT1 signaling pathways by ItaCORMs. BMDCs were stimulated with 1 μg ml−1 LPS and co-incubated with PBS, in the presence of 15 μM of ItaCORMs, or 1-EI, 4-EI and rac-8 as control substances. After 2 h, cell lysates were harvested and analyzed by Western blot using HO-1, STAT1, pSTAT1 and α-tubulin antibodies. (A) Representative Western blots. As loading control α-tubulin was used. (B and C) The video-densiometric quantification of the HO-1/α-tubulin (B) and pSTAT1/STAT1 (C) ratios are shown in percent of the LPS-treated control group. Values are means ± SEM (n = 2–5); **, p ≤ 0.01; ****, p ≤ 0.0001 (ANOVA with Tukey post hoc test). | |
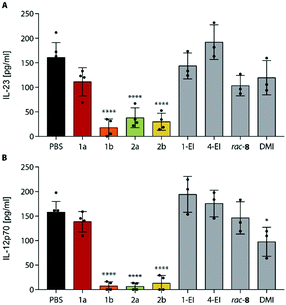 |
| Fig. 6 Strong inhibition of inflammatory cytokines by ItaCORMs. Concentrations of IL-23 (A) and IL-12p70 (B) were determined in the supernatant of BMDCs stimulated with 1 μg ml−1 LPS and co-incubated with PBS, 15 μM ItaCORMs, or 1-EI, 4-EI, rac-8, and DMI as control substances for 24 h. Values are means ± SEM (n = 3–6); *, p ≤ 0.05; ****, p ≤ 0.0001 (ANOVA with Tukey post hoc test). | |
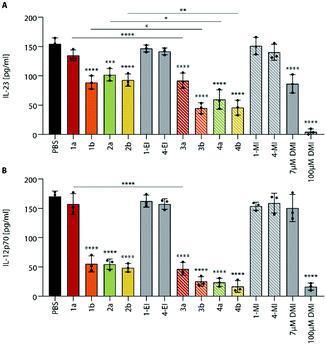 |
| Fig. 7 Comparison of ethyl itaconate (fully colored bars) and methyl itaconate (dashed bars) derived ItaCORMs. BMDCs were stimulated with 1 μg ml−1 LPS and co-incubated with PBS, ethyl-ItaCORMs1a, 1b, 2a, 2b, methyl-ItaCORMs3a, 3b, 4a, 4b or control compounds (7 μM). After 24 h of incubation, cell culture supernatants were collected and analyzed for IL-23 (A) and IL-12p70 (B) cytokine levels. Values are means ± SEM (n = 3); *, p ≤ 0.05; **, p ≤ 0.01; ***, p ≤ 0.001; ****, p ≤ 0.0001 (ANOVA with Tukey post hoc test). | |
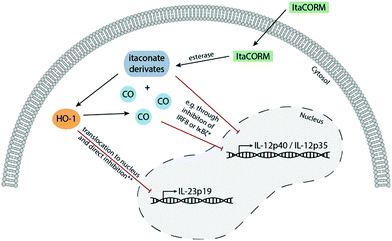 |
| Fig. 8 Possible mechanisms of the anti-inflammatory activity of ItaCORMs. After cleavage of ItaCORMs by esterases, itaconate and CO are released into the cytoplasm of the cell. This results in the induction of heme oxygenase (HO-1), which, after translocation into the nucleus, is responsible for the inhibition of IL-23 expression. Furthermore, itaconate and CO cause decreased levels of Il-12, possibly due to inhibition of transcription factors, e.g. the interferon regulatory factor 8 (IRF8) or IκBζ as the inhibitor of NF-κBζ. | |
As another activity, we also observed a strong downregulation of inflammatory cytokines IL-23 and IL-12p70 upon treatment of LPS-stimulated BMDCs with ItaCORMs (Fig. 6). While ItaCORM1a again showed only minor effects (as in the HO-1 induction), ItaCORMs1b, 2a, and 2b caused a pronounced reduction of cytokine levels. Again, the latter compounds proved to be much more potent than any of the controls applied at the same concentration. Noteworthy, dimethyl itaconate (DMI), used as a highly active benchmark compound in the recent literature,5 showed only a minor effect at these concentrations.
Since the itaconate esters derived from different alcohols may differ in their biological activity,5 we also investigated the methyl itaconate-derived ItaCORMs3a, b and 4a, b in comparison to the ethyl esters (ItaCORMs1a, 1b, 2a, 2b) with regard to their ability to inhibit the expression of inflammatory cytokines (Fig. 7).
We found, that at the low concentration of 7 μM the methyl esters caused an even stronger decrease of IL-23 and IL-12p70 than the initially studied ethyl esters. As Fig. 7 reveals, the relative activity within the two series proved to be similar. Remarkably, an almost complete suppression of IL-23 was observed upon treatment of BMDCs with ItaCORMs3b, 4a or 4b at a concentration of 15 μM (ESI† Fig. S10). At this concentration, IL-12p70 totally disappeared in the presence of all ItaCORMs investigated except for 1a and 3a, the latter being also highly active at this concentration. Fig. 7 also indicates again the superb anti-inflammatory efficacy of the ItaCORMs as compared to 7 μM dimethyl itaconate (DMI), which displayed comparable effects only at much higher concentrations (100 μM).
Discussion
While gaseous CO is highly toxic upon inhalation it has beneficial cellular effects at low concentrations. Physiologically, CO is produced by HO-1, the enzyme that catalyzes the rate-limiting step in heme degradation. CO has been shown to mediate the beneficial effects of HO-1, including protection against oxidative stress, modulation of the inflammatory response and regulation of angiogenesis.18 Our data that ItaCORMs induce HO-1 at low concentrations therefore point to a positive feed-forward mechanism that leads to sustained activation of the HO-1/CO axis. Furthermore, ItaCORMs as presented in this study combine esterase-triggered (intracellular) co-release of CO and an anti-inflammatory itaconate. The results indicate that the two agents then synergistically activate two pathways: one leading to HO-1-dependent downregulation of IL-23 and the other one resulting in repression of IL-12p70, presumably via inhibition of IRF8 or IκBζ (Fig. 8). Together, this promotes an anti-inflammatory type II immune response.19 Regarding this, ItaCORMs proved to be by far more effective than the single treatment with an itaconate, CO alone or other related CORMs. Particularly, the concentrations of itaconates required to induce anti-inflammatory cellular effects in the absence of a CO source are in the range of 100 μM.4,5 This is about 10 times higher than the effective concentration of ItaCORMs described in the study.
Experimental
General procedure for the synthesis of ItaCORMs
(Reactions were run on a 127–520 μmol scale under argon.) In a Schlenk tube, a 0.3 M solution of the respective itaconic monoester (11 or 12) (1.4–1.5 eq.) in CH2Cl2 was cooled to 0 °C. Ghosez's reagent (1.5–1.7 eq.) was added and the mixture was stirred for 1 h (M1). In a separate Schlenk tube, a 0.3–0.5 M solution of complex rac-9a or rac-9b (1.0 eq.) in CH2Cl2 was cooled to 0 °C and TBAF (1 M in THF, 1.0 eq.) was added dropwise under stirring (M2). After 10 min solution M2 was transferred dropwise into M1 at 0 °C. The resulting solution was stirred at 0 °C for 10 min and then for 16–21 h at room temperature. The solvent was removed under reduced pressure and the crude product was purified by column chromatography on SiO2 (Cyhex/EtOAc; 20/1 to 5/1) to give the respective ItaCORM. For details, including substance data, PLE-triggered CO-release experiments and biochemical investigations, see the ESI.†
Mice
Wildtype C57BL/6J mice were maintained and bred under specific pathogen-free conditions at the premises of the Eberhard Karls University Tübingen. Mouse handling and isolation of cells from bone marrow were approved by the “Einrichtung für Tierschutz, Tierärztlichen Dienst und Labortierkunde” and the “Regierungspräsidium Tübingen” and were performed with female and male animals aged 8 weeks and older. All animal procedures were performed in accordance with the Guidelines for Care and Use of Laboratory Animals of the University of Tübingen Germany. All animal experiments were approved by the local authorities (Regierungspräsidium Tübingen, Germany).
Isolation of BMDCs
Bone marrow derived dendritic cells were isolated as previously described.20 In brief, bone marrow cells were obtained from the femurs and tibias of mice. Differentiation of cells into BMDCs was performed by culture in DMEM media supplemented with 10% fetal calf serum, 1% non-essential amino acids, 1% sodium pyruvate, 1% HEPES, 1% antibiotics, 25 μM β-mercaptoethanol and 20 ng ml−1 recombinant granulocyte-macrophage colony-stimulating factor (GM-CSF). Refreshment with new cell culture medium was performed on days 3 and 6. After 7 days, harvest of immature BMDCs was performed by careful collection of non-adherent cells.
Western blot analysis
BMDCs were treated as indicated in the figure description and protein expression was analyzed as previously described.12 Briefly, cell lysates were separated by SDS-PAGE and blotted onto polyvinylidene difluoride transfer membranes. Protein detection was performed by incubation with HO-1, STAT1, pSTAT1 and α-tubulin antibodies and using the Odyssey SA Infrared Imaging System.
Cytokine analysis
BMDCs were stimulated with LPS and co-incubated with the respective ItaCORMs or control substances as indicated in the figure legend. After 24 h, supernatants of each culture condition were collected and assayed for IL-12p70 and IL-23 levels. ELISA was performed using DuoSet ELISA Mouse IL-12p70, DuoSet ELISA Mouse IL-23 and DuoSet Ancillary Reagent Kit 2 (R&D Systems).
Conclusions
We have synthesized and chemically characterized a new class of acyloxydiene–Fe(CO)3 complexes which upon esterase-triggered cleavage disintegrate to concomitantly release carbon monoxide (CO) and an itaconate as two important anti-inflammatory and immunomodulating agents. Some of these compounds, especially the 4-methyl itaconate-derived ItaCORMs4a and 4b were then shown in cellular assays to induce HO-1 and to strongly inhibit the expression of inflammatory cytokines IL-23 and IL-12p70 at low micromolar concentration. ItaCORMs4a and 4b therefore represent particularly promising anti-inflammatory agents which may deserve further clinical evaluation.
Author contributions
T. W. and H.-G. S. initiated research; B. M. K. performed chemical syntheses and CO-release studies; B. B. performed biochemical investigations; J.-M. N. performed X-ray crystal structure analyses; B. M. K., B. B., T. W. and H.-G. S. wrote the manuscript.
Conflicts of interest
There are no conflicts to declare.
Acknowledgements
This work was supported by the German Science Foundation (project SCHM 857-20-1) and the Wilhelm Sander-Stiftung (Project No. 2020.100.1).
Notes and references
- For recent reviews, see:
(a) H. A. Blair, Drugs, 2019, 79, 1965–1976 CrossRef PubMed;
(b) S. Kourakis, C. A. Timpani, J. B. de Haan, N. Gueven, D. Fischer and E. Rybalka, Pharmaceuticals, 2020, 13, 306 CrossRef CAS PubMed ; and ref. cited therein.
-
(a) M. D. Kornberg, P. Bhargava, P. M. Kim, V. Putluri, A. M. Snowman, N. Putluri, P. A. Calabresi and S. H. Snyder, Science, 2018, 360, 449–453 CrossRef CAS PubMed;
(b) M. S. Brennan, M. F. Matos, B. Li, X. Hronowski, B. Gao, P. Juhasz, K. J. Rhodes and R. H. Scannevin, PLoS One, 2015, 10, e0120254 CrossRef PubMed.
-
(a) R. Li, P. Zhang, Y. Wang and K. Tao, Oxid. Med. Cell. Longevity, 2020, 2020, 5404780 Search PubMed;
(b) A. Hooftman and L. A. J. O'Neill, Trends Immunol., 2019, 40, 687–698 CrossRef CAS PubMed;
(c) L. A. J. O'Neill and M. N. Artyomov, Nat. Rev. Immunol., 2019, 19, 273–281 CrossRef PubMed;
(d) M. Sano, T. Tanaka, H. Ohara and Y. Aso, Appl. Microbiol. Biotechnol., 2020, 104, 9041–9051 CrossRef CAS PubMed.
- E. L. Mills, D. G. Ryan, H. A. Prag, D. Dikovskaya, D. Menon, Z. Zaslona, M. P. Jedrychowski, A. S. H. Costa, M. Higgins, E. Hams, J. Szpyt, M. C. Runtsch, M. S. King, J. F. McGouran, R. Fischer, B. M. Kessler, A. F. McGettrick, M. M. Hughes, R. G. Carroll, L. M. Booty, E. V. Knatko, P. J. Meakin, M. L. J. Ashford, L. K. Modis, G. Brunori, D. C. Sévin, P. G. Fallon, S. T. Caldwell, E. R. S. Kunji, E. T. Chouchani, C. Frezza, A. T. Dinkova-Kostova, R. C. Hartley, M. P. Murphy and L. A. O'Neill, Nature, 2018, 556, 113–117 CrossRef CAS PubMed.
- M. Bambouskova, L. Gorvel, V. Lampropoulou, A. Sergushichev, E. Loginicheva, K. Johnson, D. Korenfeld, M. E. Mathyer, H. Kim, L.-H. Huang, D. Duncan, H. Bregman, A. Keskin, A. Santeford, R. S. Apte, R. Sehgal, B. Johnson, G. K. Amarasinghe, M. P. Soares, T. Satoh, S. Akira, T. Hai, C. de Guzman Strong, K. Auclair, T. P. Roddy, S. A. Biller, M. A. Jovanovic, E. Klechevsky, K. M. Stewart, G. J. Randolph and M. N. Artyomov, Nature, 2018, 556, 501–504 CrossRef CAS PubMed.
-
(a) M. Chen, H. Sun, M. Boot, L. Shao, S.-J. Chang, W. Wang, T. T. Lam, M. Lara-Tejero, E. H. Rego and J. E. Galán, Science, 2020, 369, 450–455 CrossRef CAS PubMed;
(b) A. Swain, M. Bambouskova, H. Kim, P. S. Andhey, D. Duncan, K. Auclair, V. Chubukov, D. M. Simons, T. P. Roddy, K. M. Stewart and M. N. Artyomov, Nat. Metab., 2020, 2, 594–602 CrossRef CAS PubMed;
(c) A. Hooftman, S. Angiari, S. Hester, S. E. Corcoran, M. C. Runtsch, C. Ling, M. C. Ruzek, P. F. Slivka, A. F. McGettrick, K. Banahan, M. M. Hughes, A. D. Irvine, R. Fischer and L. A. J. O'Neill, Cell Metab., 2020, 32, 468–478.e467 CrossRef CAS PubMed;
(d) S.-T. Liao, C. Han, D.-Q. Xu, X.-W. Fu, J.-S. Wang and L.-Y. Kong, Nat. Commun., 2019, 10, 5091 CrossRef PubMed;
(e) J. Domínguez-Andrés, B. Novakovic, Y. Li, B. P. Scicluna, M. S. Gresnigt, R. J. W. Arts, M. Oosting, S. J. C. F. M. Moorlag, L. A. Groh, J. Zwaag, R. M. Koch, R. ter Horst, L. A. B. Joosten, C. Wijmenga, A. Michelucci, T. van der Poll, M. Kox, P. Pickkers, V. Kumar, H. Stunnenberg and M. G. Netea, Cell Metab., 2019, 29, 211–220.e215 CrossRef PubMed;
(f) W. Qin, K. Qin, Y. Zhang, W. Jia, Y. Chen, B. Cheng, L. Peng, N. Chen, Y. Liu, W. Zhou, Y.-L. Wang, X. Chen and C. Wang, Nat. Chem. Biol., 2019, 15, 983–991 CrossRef CAS PubMed.
-
(a) Key reference: R. Motterlini and L. E. Otterbein, Nat. Rev. Drug Discovery, 2010, 9, 728–743 CrossRef CAS PubMed;
(b) U. Goebel and J. Wollborn, Intensive Care Med. Exp., 2020, 8, 2 CrossRef PubMed;
(c) C. Steiger, C. Hermann and L. Meinel, Eur. J. Pharm. Biopharm., 2017, 118, 3–12 CrossRef CAS PubMed;
(d) R. Motterlini and R. Foresti, Am. J. Physiol., 2017, 312, C302–C313 CrossRef PubMed.
- For leading reviews, see:
(a) C. C. Romao, W. A. Blattler, J. D. Seixas and G. J. Bernardes, Chem. Soc. Rev., 2012, 41, 3571–3583 RSC;
(b) U. Schatzschneider, Br. J. Pharmacol., 2015, 172, 1638–1650 CrossRef CAS PubMed;
(c) K. Ling, F. Men, W.-C. Wang, Y.-Q. Zhou, H.-W. Zhang and D.-W. Ye, J. Med. Chem., 2018, 61(7), 2611–2635 CrossRef CAS PubMed;
(d) X. Ji and B. Wang, Acc. Chem. Res., 2018, 51, 1377–1385 CrossRef CAS PubMed;
(e) C. P. Hopper, L. K. De La Cruz, K. V. Lyles, L. K. Wareham, J. A. Gilbert, Z. Eichenbaum, M. Magierowski, R. K. Poole, J. Wollborn and B. Wang, Chem. Rev., 2020, 120, 13273–13311 CrossRef CAS PubMed , and ref. cited therein; for selected recent work, see also:;
(f) X. Ji, C. Zhou, K. Ji, R. E. Aghoghovbia, Z. Pan, V. Chittavong, B. Ke and B. Wang, Angew. Chem., Int. Ed., 2016, 55, 15846–15851 CrossRef CAS PubMed;
(g) X. Ji, K. Damera, Y. Zheng, B. Yu, L. E. Otterbein and B. Wang, J. Pharm. Sci., 2016, 105, 406–416 CrossRef CAS PubMed;
(h) L. K. C. De La Cruz, S. L. Benoit, Z. Pan, B. Yu, R. J. Maier, X. Ji and B. Wang, Org. Lett., 2018, 20, 897–900 CrossRef CAS PubMed;
(i) L. K. De La Cruz, X. Yang, A. Menshikh, M. Brewer, W. Lu, M. Wang, S. Wang, X. Ji, A. Cachuela, H. Yang, D. Gallo, C. Tan, L. Otterbein, M. de Caestecker and B. Wang, Chem. Sci., 2021, 12, 10649–10654 RSC.
-
(a) X. Ji, Z. Pan, C. Li, T. Kang, L. K. C. De La Cruz, L. Yang, Z. Yuan, B. Ke and B. Wang, J. Med. Chem., 2019, 62, 3163–3168 CrossRef CAS PubMed;
(b) X. Wang, X. Chen, L. Song, R. Zhou and S. Luan, J. Mater. Chem. B, 2020, 8, 9325–9334 RSC;
(c) S. Romanski, B. Kraus, U. Schatzschneider, J. M. Neudörfl, S. Amslinger and H.-G. Schmalz, Angew. Chem., Int. Ed., 2011, 50, 2392–2396 CrossRef CAS PubMed;
(d) N. S. Sitnikov, Y. Li, D. Zhang, B. Yard and H.-G. Schmalz, Angew. Chem., Int. Ed., 2015, 54, 12314–12318 CrossRef CAS PubMed;
(e) N. S. Sitnikov, Y. B. Malysheva, A. Y. Fedorov and H.-G. Schmalz, Eur. J. Org. Chem., 2019, 6830–6837 CrossRef CAS.
-
(a) S. Romanski, H. Rücker, E. Stamellou, M. Guttentag, J.-M. Neudörfl, R. Alberto, S. Amslinger, B. Yard and H.-G. Schmalz, Organometallics, 2012, 31, 5800–5809 CrossRef CAS;
(b) S. Romanski, B. Kraus, M. Guttentag, W. Schlundt, H. Rucker, A. Adler, J.-M. Neudörfl, R. Alberto, S. Amslinger and H.-G. Schmalz, Dalton Trans., 2012, 41, 13862–13875 RSC.
- S. Romanski, E. Stamellou, J. T. Jaraba, D. Storz, B. K. Krämer, M. Hafner, S. Amslinger, H.-G. Schmalz and B. A. Yard, Free Radical Biol. Med., 2013, 65, 78–88 CrossRef CAS PubMed.
- B. Bauer, A.-L. Göderz, H. Braumüller, J. M. Neudörfl, M. Röcken, T. Wieder and H.-G. Schmalz, ChemMedChem, 2017, 12, 1927–1930 CrossRef CAS PubMed.
-
(a) J. L. Wilson, S. F. Kobeissi, S. Oudir, B. Haas, B. Michel, J.-L. Dubois Randé, A. Ollivier, T. Martens, M. Rivard, R. Motterlini and R. Foresti, Chem. – Eur. J., 2014, 20, 14698–14704 CrossRef CAS PubMed;
(b) R. Motterlini, A. Nikam, S. Manin, A. Ollivier, J. L. Wilson, S. Djouadi, L. Muchova, T. Martens, M. Rivard and R. Foresti, Redox Biol., 2019, 20, 334–348 CrossRef CAS PubMed;
(c) Z. El Ali, A. Ollivier, S. Manin, M. Rivard, R. Motterlini and R. Foresti, Redox Biol., 2020, 34, 101521 CrossRef CAS PubMed.
- F. Munyemana, I. George, A. Devos, A. Colens, E. Badarau, A.-M. Frisque-Hesbain, A. Loudet, E. Differding, J.-M. Damien, J. Rémion, J. Van Uytbergen and L. Ghosez, Tetrahedron, 2016, 72, 420–430 CrossRef CAS.
- K. Achiwa, P. A. Chaloner and D. Parker, Organomet. Chem., 1981, 218, 249–260 CrossRef CAS.
- A. Madaan, R. Verma, A. T. Singh, S. K. Jain and M. Jaggi, J. Biol. Methods, 2014, 1, e1 CrossRef.
- T. Wieder, C. E. Orfanos and C. C. Geilen, J. Biol. Chem., 1998, 273, 11025–11031 CrossRef CAS PubMed.
- A. Loboda, A. Jozkowicz and J. Dulak, Vasc. Pharmacol., 2015, 74, 11–22 CrossRef CAS PubMed.
- K. Ghoreschi, J. Brück, C. Kellerer, C. Deng, H. Peng, O. Rothfuss, R. Z. Hussain, A. R. Gocke, A. Respa, I. Glocova, N. Valtcheva, E. Alexander, S. Feil, R. Feil, K. Schulze-Osthoff, R. A. Rupec, A. E. Lovett-Racke, R. Dringen, M. K. Racke and M. Röcken, J. Exp. Med., 2011, 208, 2291–2303 CrossRef CAS PubMed.
- A. Madaan, R. Verma, A. T. Singh, S. K. Jain and M. Jaggi, J. Biol. Methods, 2014, 1, e1 CrossRef.
Footnote |
† Electronic supplementary information (ESI) available: Synthetic procedures, substance data, spectra, CO-release studies, and cell-biological studies. CCDC 2077461 and 2077462. For ESI and crystallographic data in CIF or other electronic format see DOI: 10.1039/d1md00163a |
|
This journal is © The Royal Society of Chemistry 2021 |
Click here to see how this site uses Cookies. View our privacy policy here.