DOI:
10.1039/D1MA00216C
(Review Article)
Mater. Adv., 2021,
2, 3234-3250
Technologies of lithium recycling from waste lithium ion batteries: a review†
Received
11th March 2021
, Accepted 27th April 2021
First published on 28th April 2021
Abstract
The consumption of lithium-based materials has more than doubled in eight years due to the recent surge in demand for lithium applications as lithium ion batteries. The lithium-ion battery market has grown steadily every year and currently reaches a market size of $40 billion. Lithium, which is the core material for the lithium-ion battery industry, is now being extracted from natural minerals and brines, but the processes are complex and consume a large amount of energy. In addition, lithium consumption has increased by 18% from 2018 to 2019, and it can be predicted that the depletion of lithium is imminent with limited lithium reserves. This has led to the development of technologies to recycle lithium from lithium-ion batteries. This article focuses on the technologies that can recycle lithium compounds from waste lithium-ion batteries according to their individual stages and methods. The stages are divided into the pre-treatment stage and lithium extraction stage, while the latter is divided into three main methods: pyrometallurgy, hydrometallurgy, and electrochemical extraction. Processes, advantages, disadvantages, lithium extraction efficiency, price, environmental pollution and the degree of commercialization of each method are compared and analyzed quantitatively. Despite the growing attention and the development of various lithium recycling technologies, less than 1 percent of lithium is recycled currently. We propose future needs to improve the recycling technologies from waste lithium materials and hope that this article can stimulate further interest and development in lithium recycling.
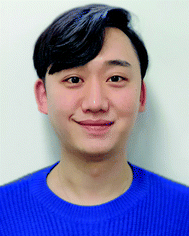
Hyuntae Bae
| Hyuntae Bae graduated with a BS in chemistry from Gyeongsang National University 2014. In 2014, he joined the group of Prof. Youngsik Kim at the Ulsan National Institute of Science and Technology (UNIST), where his research currently focuses on recycling lithium from the waste lithium-ion batteries. |
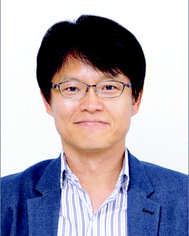
Youngsik Kim
| Youngsik Kim is a professor at School of Energy and Chemical Engineering at Ulsan National Institute of Science & Technology (UNIST). He received his PhD (2006, advisor: Prof. Steve W. Martin) in Materials Science and Engineering from Iowa State University. After, he joined Prof. John B. Goodenough's group at The University of Texas at Austin as a post-doctoral fellow. He conducted research in areas including lithium- and sodium-ion batteries. Particularly, he is an inventor of the ‘sea-water battery’, which is developed as an alternative option for grid-scale energy storage. He is also the CEO of energy solution company, 4TOONE Corporation. |
1. Introduction
Discussions regarding lithium-based technology have dominated the field of energy research in recent years. From the first commercialization in 1991, the lithium-ion battery has been a core energy technology and it has been continuously researched for several decades for the development of the future energy market.1–7 Lithium is attracting attention as it is a key element of lithium-ion batteries. However, lithium is not evenly distributed around the world and is a limited element. Furthermore, with the rapid development of electric vehicle (EV) and energy storage system (ESS) technologies, the demand for lithium is rapidly increasing. Lithium consumption has more than doubled over the last decade.8,9 With a continuous consumption increase of lithium, our future energy market will suffer tremendous damage.
For these reasons, lithium is expected to become more valuable, highlighting the importance of lithium recycling. This paper explains the current state of lithium and emphasizes the importance of lithium recycling. In addition, this paper lists the various techniques used for lithium recycling, along with the process, advantages, and drawbacks of each method and concludes with recommendations for research and development in lithium recycling technologies.
2. Current state of lithium
2.1 Lithium usage
In 2011, 30% of the lithium consumption was for ceramics and glass, followed by batteries and greases/castings.10,11 However, the application of batteries to small electronics such as smartphones and laptops, as well as larger systems such as electric vehicles (EVs) and energy storage systems (ESS), has caused 60% of the lithium consumption to be associated with batteries12 (Fig. 1a).
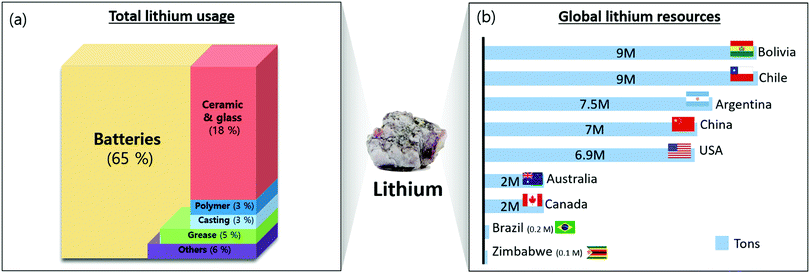 |
| Fig. 1 (a) Distribution of total lithium usage in 2019; (b) distribution of global lithium resources. | |
Lithium is preferred as the material for batteries owing to three properties: (1) it is the lightest metal (2) it has the highest electrochemical potential of all metals, and (3) it has the highest energy density among all metals.13 In addition to batteries, lithium is also used in ceramics, glass, and greases/castings with particular chemical properties. Adding lithium to ceramics increases the glass melt rate and decreases their viscosity and melt temperature. Additionally, the high thermal expansion coefficient of lithium makes ceramics resilient to heat shock and increases mechanical strength.14 Lithium grease is useful in concentrating oil to produce high-temperature grease,15 and lithium additives are used in casting to increase fluidity.16 Organic lithium materials are used as strong bases in polymers to form carbon–carbon bonds.17
2.1 Lithium production from nature resources
Even though lithium is used widely, its reserves and production are not evenly distributed across the world. South American nations, such as Chile, Bolivia, and Argentina, own more than 70% of the global lithium deposits18 (Fig. 1b). Australia produces the most lithium (43.5% of global production), followed by Chile (32.8%).19 Argentina and China export a significant quantity of lithium19 (Fig. 1b).
Lithium is extracted from two natural sources: ores and salt lakes. Lithium can be extracted from spodumene, petalite, and eucryptite, and the composition of the lithium in these ores ranges from 2% to 5.5%20 (Table 1). The minerals are crushed, separated by gravity flotation, concentrated, and heat-processed for water leaching, acid treatment, and pressure leaching to produce the lithium solution. Na2CO3 was added to the lithium solution to extract lithium sediments.18,21,22
Table 1 Various lithium ores and their lithium content
Ore |
Chemical formula |
Li contained (wt%) |
Spodumene |
LiAlSi2O6 |
3.73 |
Petalite |
LiAlSi4O10 |
2.09 |
Amblygonite |
(Li,Na)AlPO4(F,OH) |
3.44 |
Lepidolite |
K(Li,Al)3(Si,Al)4O10(F,OH)2 |
3.58 |
Eucryptite |
LiAlSiO4 |
5.51 |
The second method for extracting lithium is from a salt lake or brine. Lithium brines exist in Argentina, Bolivia, Chile, China, and the USA.21 The lithium grade and magnesium/lithium ratio of the brine are important factors that affect the production cost and time.23–28Table 2 lists the lithium content and Mg/Li ratio of the brines currently used in production.21 Brine contains 0.02 to 5% Li+, along with other ions such as Na+, K+, Cl−, and SO42−.27 Lithium is extracted from brine using the precipitation method. Water is evaporated from the brine in a solar pond, followed by the precipitation of NaCl and KCl, and the concentration of lithium in the solution. Next, Ca(OH)2 is added to the concentrated lithium brine to remove Mg and sulfate via precipitation. The remaining Ca and Na2CO3 in the concentrate are removed via precipitation using CaCO3. This fluid then undergoes filtration and washing and is heated to 80–90 °C to react with Na2CO3 and form Li2CO3.27 (Fig. 2) While solar evaporation followed by precipitation is a popular method, the procedure is time-consuming, lasting from 18 to 24 months.28 For brines with a high Mg/Li ratio, Al is used to precipitate lithium–aluminate. This method exhibits superior lithium separation and precipitation rates.29 This method involves the addition of AlCl3, H2O, NaOH, and amorphous Al(OH)3, followed by adjusting the temperature and pH to extract lithium as LiAlO2 (eqn (1)).
| LiCl + 2AlCl3 + 6NaOH → LiCl·2Al(OH)3 H2O + 6NaCl | (1) |
Table 2 Properties of lithium salt lakes
Salt lake |
Country |
Li contained (%/ton) |
Mg/Li |
Atacama |
Chile |
0.15 |
6.4 |
Hombre muerto |
Argentina |
0.062–5 |
1.37 |
Rincon |
Argentina |
0.033 |
8.61 |
Uyuni |
Bolivia |
0.035 |
18.6–21.5 |
Silver peak |
USA |
0.023 |
1.43 |
Zhabuye lake |
China |
0.12 |
Low |
DXC salt lake |
China |
0.04 |
0.22 |
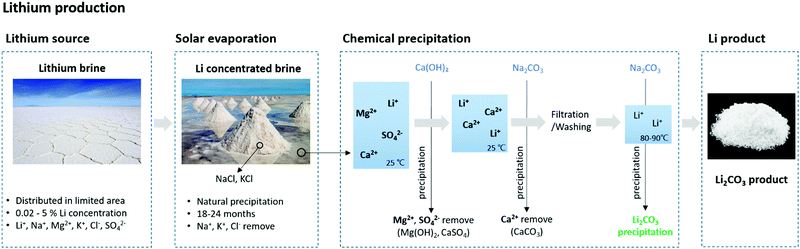 |
| Fig. 2 Process example of Lithium extraction processes from natural lithium brine via chemical precipitation. | |
While extracting lithium from ores ensures higher lithium concentration, the production cost is higher than that of brine extraction because of the more energy-intensive procedure. Thus, 87% of lithium is produced by brine extraction.30 Lithium also exists in seawater, which is quite abundant, but its concentration is too low (0.17 ppm) to be economical.31,32 However, there are ongoing research efforts to reduce the cost of lithium extraction.
2.3 Demand for lithium recycling
The price of Li2CO3, which is the commonly traded form of lithium in the global market, has increased by 2.6 times from 6500 dollar per metric tons in 2015 to 17
000 dollar per metric tons in 201833 (Fig. S1, ESI†). There has been a steady increase in the adoption of lithium-battery-powered personal electronics, electronic vehicles (EVs), and ESS owing to the thrust on green energy. These trends have expanded the lithium-ion battery market to $44 billion in 2020.34 The growth in the ESS and EV battery markets has further accelerated this expansion. To illustrate the potential increase in demand, it must be noted that while smartphones, laptops, and tablets contain approximately 2 g, 6 g, and 20 g of lithium per device, respectively,35 an EV battery requires approximately 20 kg of lithium, which is 1000 times that of a smartphone, and a 10 MWh ESS requires at least 700 kg of lithium.35,36 As the demand for lithium-ion batteries increases, the increase in lithium prices can weaken the lithium-ion battery market.
Lithium consumption rose from 49
100 tons in 2018 to 57
700 tons in 2019, registering an increase of 18%.37 Global lithium reserves are estimated to be approximately 17 billion tons. If the 18% annual increase in consumption continues, global lithium reserves will be exhausted. In 30 years, the demand for lithium will be approximately 63 million tons, which will be 40 million tons more than the remaining reserves (Fig. 3). Although more lithium reserves may eventually be discovered, the increase in demand is predicted to accelerate owing to the expanding market for lithium-ion batteries. An increasing number of agencies across the world forecast shortages of lithium and these predictions have gained much research interest in recent years.38,39
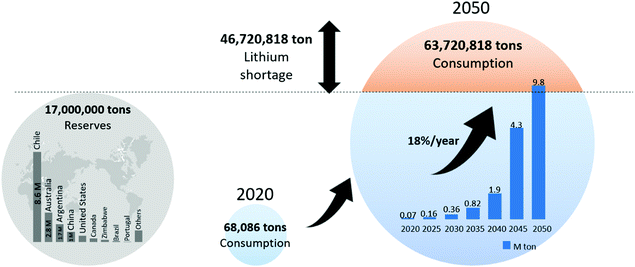 |
| Fig. 3 Global lithium reserves and lithium depletion scenario after 30 years when lithium consumption increasing rate is 18% per year. | |
3. Waste lithium-ion battery and pre-treatment
3.1 Waste lithium-ion batteries
Research on lithium recycling has focused mainly on discarded lithium-ion batteries. Lithium-ion batteries function by the movement of Li+ ions and electrons, and they consist of an anode, cathode, electrolyte, and separator. The cathode, depending on its usage and capacity, consists of lithium-containing compounds such as LiFePO4 and LiNi0.3Mn0.3Co0.3O2. The anode consists of carbon materials like graphite that can accept lithium ions. The cathode and the anode are connected to aluminum or copper foil with a polymer binder such as polyvinylidene fluoride (PVDF), for electrical conduction and stability. Electrolytes are usually liquids and act to deliver lithium ions between the cathode and anode. These are usually lithium salts (e.g., LiPF6) dissolved in organic solvents such as ethylene carbonate (EC) or dimethyl carbonate (DMC). Polymers such as polypropylene (PP) or polyethylene (PE) are used as separators to prevent physical contact and direct electron transfer between the cathode and the anode.
A lithium-ion battery can last up to three years in a small electronic device, and from five to ten years in a larger device; this is shorter than the lifespan of other batteries, considering that Ni–Cd batteries last from fifteen to twenty years, and lead-acid batteries last from five to ten years.40–44 Currently, 80% of lithium-ion batteries are used for small electronics, with EV and ESS applications accounting for less than 20%.45 Lithium-ion battery disposal was estimated to be 10
700 tons in 2012.46 This value has increased progressively each year, with an estimate of up to 250
000 tons in 2020.45 With the large-scale adoption of EVs and ESSs, the number of lithium-ion batteries in service is expected to increase. Discarded lithium-ion batteries are collected by government authorities or companies authorized by the manufacturer. Consumers normally dispose of lithium-ion batteries in a government-designated area or these are directly collected by the relevant agency. However, only 2–5% of lithium-ion batteries are collected in Australia, the EU, and the US47 (Fig. 4). The reason for the low collection rate is the lack of consumer awareness, as well as the tendency to resell electronics rather than recycle them. Although it may vary by country, there is a lack of legal and physical infrastructure for widespread collection and efficient, safe, and economical transportation of the disposed lithium-ion batteries. Major improvements are required to significantly increase the collection rate.
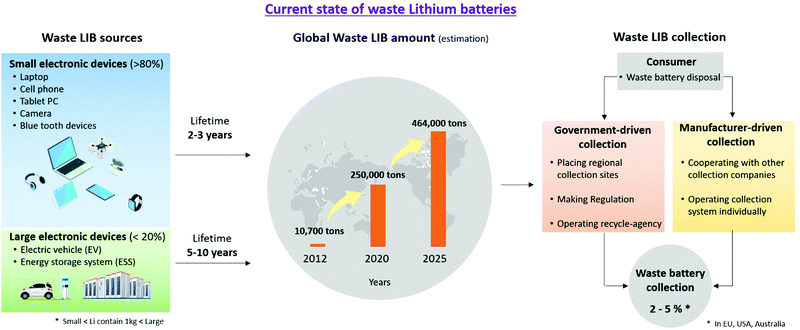 |
| Fig. 4 Sources, quantity and collection of discarded lithium-ion batteries. | |
Recycling of lithium-ion batteries has been steadily researched since the 1990s and has recently gained traction. However, most research focuses not on lithium but on more expensive materials, such as cobalt. Therefore, little focus has been placed on extracting lithium from disposed batteries. Cobalt is the most expensive material in a lithium-ion battery; thus, there has been considerable research interest in reducing the cobalt content or replacing it by using LiFePO4 or LiNi0.3Mn0.3Co0.3O2.48 However, lithium is an irreplaceable key component in lithium-ion batteries, and it is used in the cathode, electrolyte and anode. Therefore a comprehensive review of the recycling of lithium from lithium-ion battery recycling is needed.
Lithium can be extracted from a lithium-ion battery via two major processes. Owing to the difficulty of separating lithium from the packed battery, the disposed battery is subjected to a pre-treatment process to separate the lithium-containing active material(cathode, anode) from the peripheral parts(plastic, polymer). Next, lithium is chemically separated from the active materials using pyrometallurgy, hydrometallurgy and electrochemical extraction methods (Fig. 5). The two processes, pre-treatment and lithium extraction, are explained.
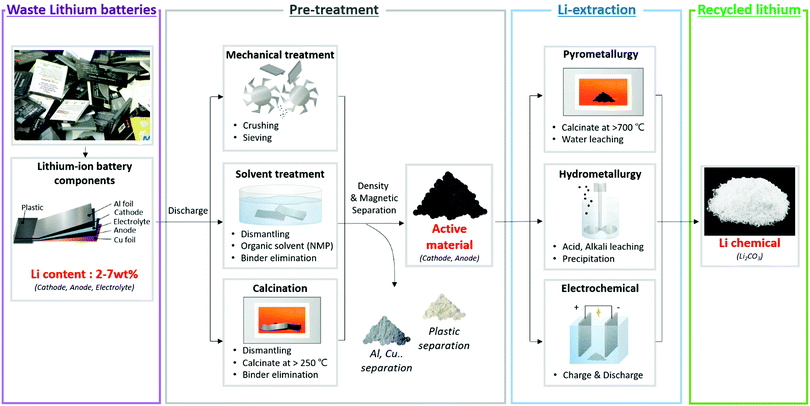 |
| Fig. 5 Schematic diagram of the overall lithium recycling stages and methods from discarded lithium-ion batteries. | |
3.2 Pre-treatment methods for waste lithium-ion battery
Quantifying the remaining power of a discarded lithium-ion battery is difficult. Even after depleting charge of the battery, the battery might still have some power remained. In addition, because lithium-ion batteries contain various materials, it is not efficient to treat the batteries directly. Therefore, a pre-treatment process is essential. Waste lithium-ion batteries can be pre-treated and separated safely only when they are fully discharged. If not, the battery can explode or emit toxic gases due to local short-circuiting. Hauck and Kurrat reviewed various methods for discharging batteries using electronic techniques and conductive liquids.49 The most used method is to submerge the battery in a conductive solution, such as a NaCl solution. Although NaCl is the most popular conductive solution, there is ongoing research on the discharge rate and corrosion rate of other conductive liquids.50,51 Methods to separate the active material from the disposed battery can be broadly categorized as mechanical separation, solution treatment separation, and calcination treatment separation (Fig. 6).
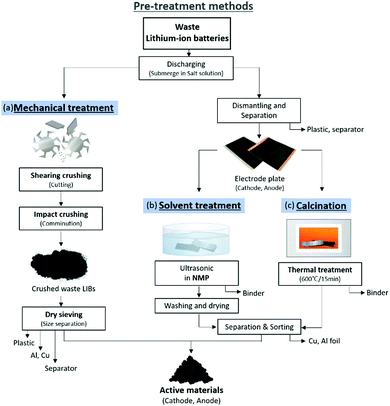 |
| Fig. 6 Example of pre-treatment technology and processes of waste LIBs; (a) mechanical (b) solvent treat and (c) calcination. | |
3.2.1 Mechanical pre-treatment.
Mechanical pre-treatment is a treatment method that involves physically grinding the discharged lithium-ion batteries.54–57 Different compartments of the battery have different mechanical properties, and so, a grinder that can uniformly grind all the compartments is needed. Generally, a grinding chamber is created by blocking off air. Various tools, such as hammer mills, granulator machines and blade crushers are used. After grinding, the active material (cathode, anode) and miscellaneous (Al, Cu) are separated by utilizing the difference in density and magnetic properties. Zhang et al. created battery pieces by shear crushing a waste battery discharged via NaCl solution immersion.52 They used impact crushing equipment fitted with blade crushers and ground the battery for 20 s at 3000 rpm. The crushed pieces were categorized into four size groups via dry sieving. The larger pieces of plastic, Al, Cu and polymers were separated from the cathode and anode materials of 0.075 mm. After removing the hydrocarbon material on the surface, LiCoO2 and graphite were separated via flotation (Fig. 6a). Mechanical pre-treatment is the most common method of lithium-ion battery separation owing to its simplicity and scalability. However, setting up a stable separation setup is essential, and this method can result in the production of noise, dust, and harmful gases. In addition, it is difficult to ensure the perfect separation of all materials using this method.
3.2.2 Solvent pre-treatment.
Solvent pre-treatment utilizes solutions and solvents to separate the active materials from the Al and Cu foils in the lithium-ion battery.58–61 This method removes the additive binder material that strengthens the foil contact with the active materials, thereby separating the active materials. The cathode and anode were combined with a binder on Al and Cu foil. By stripping off the binder, the active material can be separated from the metal foil. A well-known method is to insert the electrode plate into an organic solvent (N-methyl-2-pyrrolidine (NMP)) at 100 °C to remove the binder and separate the Al and Cu foils. He et al. discharged the lithium-ion battery by submerging it in a NaCl solution and separated the battery by hand using sharp nose pliers.60 After separating the plastic, metal case, electrode plate, and separator, the cathode was cut into small pieces. The cathode pieces were placed in NMP at 80 °C for 2 h, thereby dissolving the binder and separating the Al foil and cathode material (Fig. 6b). Most batteries use a PVDF binder to attach the active materials, but some use PTFE binders, which require the selection of an appropriate organic solvent. Furthermore, this method requires the use of organic solvents around 100 °C, which increases the cost and creates harmful waste products.
3.2.3 Calcination pre-treatment.
Calcination per-treatment occurs in the temperature range of 150–500 °C to remove carbon and organic material from the discarded lithium-ion battery.62–65 In addition, by calcinating at 250 °C to 350 °C, the PVDF binder (which connects the active materials and metal foil) can be removed, thereby reducing the adherence of the active materials on the Al and Cu foils. Yang et al. cut the cathode parts into small pieces and placed them in a tube furnace in a nitrogen atmosphere at 550–650 °C.65 Then, the cathode material and the current collector (Al foil) were easily separated using gravity separation (Fig. 6c). However, calcination treatment requires expensive calcination equipment, is energy-intensive, and can emit toxic gases.
Various methods can be used to pre-treat discarded lithium-ion batteries. Each method has its advantages and drawbacks, and a more scalable method needs to be developed for use on a commercial scale. Table 3 lists the results and conditions of the different pre-treatment methods.
Table 3 Summary of condition and residue parameters of pre-treatment processes of waste LIBs investigated in the literature
Method |
Residue |
Condition |
Ref. |
Mechanical |
Al, Cu/LCO/graphite |
• Discharge, blade crusher 3000 rpm/20 s |
52
|
Mechanical |
Active materials |
• Hammer mill (10 mm), spouted bed elutriation |
53
|
Mechanical |
Electrode powders |
• Granulator machine/10 min |
54
|
Mechanical |
Cathode powders |
• Granulator machine/10 min, shredding |
55
|
Mechanical |
Electrode powders |
• Discharge, twin-shaft shredder |
56
|
• Hammer mill (5 mm control grid)/10 min |
• Granulator machine/10 min |
Mechanical |
Active materials |
• Discharge, vertical impact crusher |
57
|
• Sieving and screening |
• Falcon series centrifugal concentrator |
Solvent |
Cathode powders |
• Discharge, manually dismantling and separation |
58
|
• NMP 60 °C/1 h, 700 °C/5 h |
Solvent |
Cathode powders |
• Discharge, manually dismantling and separation |
59
|
• Ultrasonication in NMP, 800 °C/2 h |
Solvent |
Cathode powders |
• Manually dismantling and separation |
60
|
• NMP, ultra-sonication 80 °C/2 h |
Solvent |
Cathode powders |
• Manually dismantling and separation |
61
|
• NMP, 100 °C/2 h, 700 °C/2 h |
Thermal |
Cathode powders |
• Discharge, manually dismantling and separation |
62
|
• Vacuum pyrolysis |
Thermal |
Active materials |
• Discharge, manually dismantling and separation |
63
|
• 300 °C/30 min |
Thermal |
Cathode powders |
• Dismantling and separation |
64
|
• 500 °C, ANVIL (air-jet-separation) |
Thermal |
Active materials |
• Discharge, manually dismantling and separation |
65
|
• 600 °C/15 min in N2 condition |
4. Lithium extraction technologies from pre-treated waste lithium-ion battery
Various methods are used to extract lithium from the active material obtained from the pre-treatment. Most methods focus on the extraction of Co, Ni, or Mn; however, this study focused on the extraction of lithium. In addition, this review excludes methods that have leaching solutions as the end product and focuses on technologies that produce usable lithium compounds such as Li2CO3 and Li3PO4. Fig. 4 shows the three lithium extraction methods: pyrometallurgy, hydrometallurgy, and electrochemical extraction.
4.1 Pyrometallurgy
Pyrometallurgy uses high temperatures to remove organic material via evaporation and causes reactions in the cathode and anode to make lithium soluble in water.66–76 Lithium was then recycled from the aqueous solution. The pre-treated active materials were powdered and subjected to calcination. At temperatures higher than 700 °C, the lithium metal oxide of the cathode and anode react to form Li2CO3 and metal oxides. The reactions that occur are shown in eqn (2)–(6). | C + 12LiMO2 → 6Li2O + 4M3O4 + CO2 | (2) |
| C + 4LiMO2 → 2Li2O + 4MO + CO2 | (3) |
The calcinated powder then undergoes water leaching to dissolve the lithium (Li2CO3) in the water. The metal oxide does not dissolve in the water. After water leaching via filtration, the undissolved metal oxide and the aqueous solution were separated to produce a Li2CO3 solution, followed by water evaporation to finally obtain Li2CO3 (Fig. 7). This method can process large numbers of disposed lithium-ion batteries, and the process is simple. Li et al. recycled lithium via pyrometallurgy using LiCoO2 and graphite from a pre-treated lithium-ion battery.67 The separated active materials were calcined in nitrogen at 1000 °C for 30 min to obtain Co, Li2CO3, and graphite compounds. This compound was submerged in water to separate lithium using wet magnetic separation. Pindar et al. separated lithium-ion batteries and calcinated them at 800 °C for 45 min to create a lithium-containing powder, which was dissolved in a mass of water 50 times more than the powder mass.72 This resulted in Li2CO3 with LiF mixed at a 90% lithium retrieval rate. Xiao et al. used pyrometallurgy to separate lithium from LiMn2O4, LiCoO2, and LiMnxCoyNizO2 mixed with graphite. The cathode material was separated from the battery and calcinated at 700 °C in vacuum for 30 min. The calcinated powder was a metal oxide, Li2CO3, which was then reacted with water (25 g L−1) for separation.68
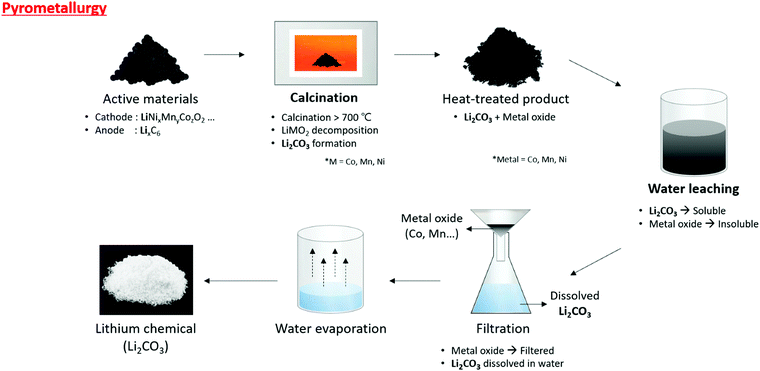 |
| Fig. 7 Overall schematic of lithium recycling from pre-treated waste LIB components by pyrometallurgy process. | |
Some pyrometallurgy uses additional acids for the roasting to higher the lithium extraction efficiency. Liu et al. used nitric acid to nitrate the lithium ion-battery scraps and roasted them at 250 °C for 60 min. After roasting, by leaching with water, lithium solution was produced and with carbonation, Li2CO3 was produced with low-temperature roasting.73 Li et al. used sulfation roasting to recycle Li2SO4 from NCM523 material by using H2SO4 with the process of roasting and water leaching.76
Chlorination roasting is also used in pyrometallurgy, which uses sintered lithium slag (xLi2O·yCaO·zAl2O3·nSiO2) with chlorine donor to form LiCl after the roasting. Chang et al. used CaCl2 and roasted it at 1000 °C for 90 min with LiAl(SiO3)2 to transform it into LiCl71 (eqn (7)).
| CaCl2 + Li2O → CaO + 2LiCl | (7) |
The biggest drawback of the pyrometallurgy method for lithium recycling is that additional steps are required after calcination. This additional process usually involves dissolving the product in water or solvent and separating it.
However, the low solubility of Li2CO3 (13 g L−1) requires a large amount of solvent. In addition, the pyrometallurgy method requires complicated calcination equipment and can cause the emission of harmful gases. Table 4 summarizes the details of the pyrometallurgy research.
Table 4 Summary of condition and residue parameters of pyrometallurgy processes of waste LIBs investigated in the literature
Spent materials |
Residue |
Efficiency |
Purity |
Condition |
Ref. |
Electrode materials |
Li concentrate |
>90% |
>99% |
• 1700 °C–1750 °C |
66
|
LiCoO2 + C |
Co + Li2CO3 + C |
98.93% |
— |
• 1000 °C/30 min in O2 free |
67
|
C + LiMn2O4, LiCoO2, LiMnxCoyNizO2 |
Li2CO3 + metal oxide |
81.90% |
99.7% |
• 700 °C/30 min |
68
|
LiMn2O4 + C |
Li2CO3 + MnO |
91.3% |
99.7% |
• 800 °C/45 min |
69
|
Cathode materials |
Li2CO3 |
84.7% |
— |
• 650 °C/3 h |
70
|
• Water leaching [100 °C/0.5 h] |
LIB lithium slag |
LiCl |
Evaporate 97.45% |
— |
• 1200 °C/1 h |
71
|
• 1000 °C/90 min with CaCl2 |
Cathode materials |
Li2CO3 solution |
>90% |
— |
• 800 °C/45 min |
72
|
LIB scraps |
Li2CO3 |
90% |
99.95% |
• 70 °C/5 h HNO3 |
73
|
• Roasting 100–300 °C |
LiCoO2 |
Li2CO3 solution |
36% |
— |
• 700 °C with carbon |
74
|
Electrode materials |
Li2CO3 |
80% |
98% |
• Carbothermal 850 °C/45 min |
75
|
4.2 Hydrometallurgy
Hydrometallurgy is the most used method for lithium extraction. It ionizes the lithium in the pre-treated active materials with acids and bases, followed by leaching to obtain Li+ solutions from which lithium can be extracted. Inorganic acids such as sulfuric acid, hydrochloric acid, and nitric acid were used. Heat or redox reactions using H2SO3, NH2OH, and H2O2 were applied to increase the leaching efficiency. Among the redox agents, H2O2 is the most common reductant because of its low cost and non-toxicity. H2O2 can increase the leaching reaction rate owing to its strong reducibility. However, using an acid with a low pH can result in the emission of harmful gases such as Cl2 and NOx, which affects the environment adversely. Thus, the use of weak acids, such as oxalic acid or citric acid, is being examined. Lithium compounds can be created using acids or bases for leaching, followed by precipitation, solvent extraction, or selective adsorption77–103 (Fig. 8).
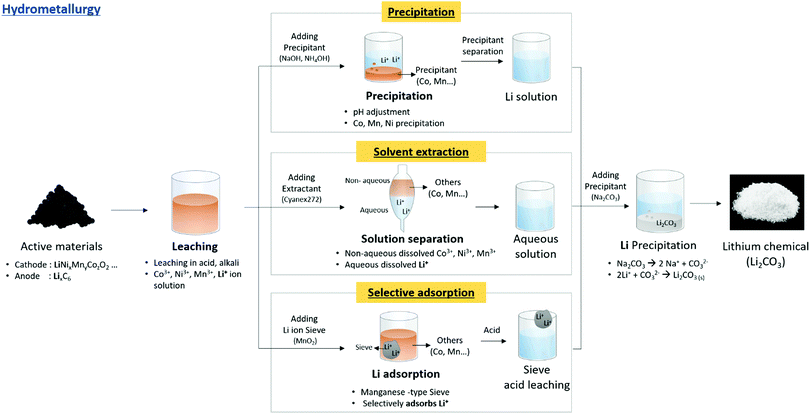 |
| Fig. 8 Overall schematic of lithium recycling from pre-treated waste LIB components by hydrometallurgy process. | |
4.2.1 Precipitation.
Precipitation is the most used method for separating lithium from leached solutions. This method uses the difference in the solubility of metal compounds, which is dependent on the specific pH and temperature.77–97 Materials with low solubility, such as transition metal hydroxides or oxalates, are precipitated. Therefore, precipitants such as NaOH, Na3PO4, and Na2CO3 are mainly used to precipitate other dissolved metals, followed by lithium extraction in the form of Li2CO3 or Li3PO4 by reacting Li+ and precipitants. For example, Li2CO3 has a higher tendency to form low-solubility Li2CO3 under high pH conditions because of the formation of CO32− ions. Also, the solubility decreases at higher temperatures (12.9 g L−1 at 25 °C, 10.8 g L−1 at 40 °C), which makes precipitation easier. Compared to other compounds (LiOH – 129 g L−1, LiCl – 815 g L−1 at 25 °C) Li2CO3 has a substantially lower solubility, which makes it easier to precipitate. This method is the safest, most economical, and most efficient (retrieval efficiency) among hydrometallurgy methods. Gao et al. precipitated Li2CO3 from separated LiNi0.3Mn0.3Co0.3O2 electrode material.84 LiNi0.3Mn0.3Co0.3O2 was leached using 2M formic acid at 70 °C and H2O2 as reductant, followed by an increase in pH when using NaOH and NH4OH solutions. The increase in pH precipitates Co, Mn, and Ni as hydroxides, which are separated. A saturated Na2CO3 solution was added to the solution to create Li2CO3. This method reportedly retrieved 98.22% of lithium. Yang et al. used precipitation to separate lithium from LiFePO4, a lithium-ion battery cathode.85 LiFePO4 cathodes are difficult to leach and so, the crystal structure needs to be destroyed via calcination or chemical treatment before leaching. To destroy the crystal structure, LiFePO4 was ball-milled with DEDTA-2Na, and the leaching rate was increased by mechanochemical activation. Leaching was performed for 20 min using 0.6 M H3PO4 (50 g L−1), followed by stirring for 9 h at 90 °C to separate FePO4 and adding 5 M of NaOH to precipitate lithium as Li3PO4. Natarajan et al. obtained Li2CO3 from a cathode containing Co and Mn. Using 3 M acetic acid and H2O2, the anode material was leached, followed by the addition of (NH4)2S to precipitate Co as CoS, and the addition of Na2CO3 to precipitate Mn as MnCO3.86 After the removal of Co and Mn, Na2CO3 was added to the solution to precipitate Li2CO3. Recycling lithium from the anode material (graphite) is investigated by Yang et al. They collected graphite by two stage calcination process. After, with 1.5 M of HCl, lithium was almost 100% leached into a liquor. By adjusting the pH and adding the sodium carbonate to the solution, Li2CO3 was precipitated.
These precipitation methods are most researched, but fine control of the operating conditions (pH, Li concentration, and temperature) is needed to produce the required property profile.
4.2.2 Solvent extraction.
Solvent extraction uses a two-phase system to separate lithium from the leached cathode material.98–102 It uses relative solubility to separate ions from polar and nonpolar liquids. Nonpolar extractants are mainly used to separate valuable metals (Co, Ni, Mn), and lithium is separated from the stratified solution. Cyanex272, PC-88a, and D2EHPA were used to separate Co, Ni, and Mn. Zhang et al. used solvent extraction to separate lithium from LiCoO2.98 The cathode material was leached using HCl, and PC-88A was used to separate the Co and Li ions. An increase in pH caused PC-88A to extract cobalt more efficiently. Using this property, at pH 6.7, cobalt was separated using an organic solvent, and lithium was separated as an aqueous solution. After cobalt extraction, the separated raffinate (lithium aqueous solution) was added to Na2CO3 to precipitate Li2CO3. Chen et al. extracted lithium from LiNi0.3Mn0.3Co0.3O2.100 They leached the LiNi0.3Mn0.3Co0.3O2 powder using 2 M H2SO4 in 70 °C for 90 min, followed by 0.1 M Na-Cyanex272 at pH 6 to separate cobalt and manganese (as an organic solvent), and lithium and nickel (as an aqueous solution). Cobalt and manganese were separated from the organic solvent using 0.2 M Na-D2EHPA at pH 2.95. Lithium and nickel are separated from the aqueous solution at pH 9 using dimethylglyoxime (DMG), which has low reactivity with lithium but high reactivity with nickel in pH 9. The nickel is separated as a solid, leaving a solution with only lithium. Na2CO3 was added to the solution to obtain Li2CO3. Recently, a compound called Cyanex936P was developed, which can separate lithium from other alkaline metals. This has the potential to be a great extractant for lithium-ion battery recycling. Solvent extraction is a shorter process than precipitation but requires additional extractant chemicals such as cyanex272 and PC-88A, along with expensive solvents. In addition, detailed control of the conditions, such as pH and solvent concentration, is needed because the extraction efficiency is greatly affected.
4.2.3 Selective adsorption.
Selective adsorption separates lithium using a lithium-ion sieve to absorb the dissolved lithium.103 Lithium-ion sieves are inorganic adsorbents that are extremely selective for lithium in a lithium-containing solution and originate from the technology used to extract lithium from solutions such as lithium brines. As lithium is the smallest metal ion, lithium-ion sieves use a vacancy to allow only lithium ions. Lithium manganese oxide has the highest selectivity, capacity, and stability among the inorganic solvents. In addition, it has low toxicity, which makes it the most commonly used material. Wang et al. leached lithium from lithium-ion batteries using an NH3–H2O–NH4HCO3 solution with H2O2.103 A manganese-type lithium-ion sieve was used to selectively adsorb lithium with the leached Li, Co, and Ni solutions. The lithium-adsorbed lithium-ion sieve was separated and dissolved in HCl. Then, NaOH and Na2CO3 were added to separate Li2CO3. This is a simple method using lithium-ion selective sieves and allows for low contamination; however, spinel-structured manganese-type lithium-ion sieves are very costly and require an additional leaching process to separate the lithium combined with MnO2.
Generally, the hydrometallurgy method guarantees high recovery rates and does not require additional equipment, making it quite popular in the laboratory and the method closest to commercialization. However, this method requires a high volume of acid and base for leaching (proportional to the lithium-ion battery), which can incur additional chemical costs. In addition, the backend costs of disposing the used solutions can be high. Table 5 shows different hydrometallurgy methods, lithium extraction conditions, and results, with a focus on studies that resulted in the production of the final compound after leaching.
Table 5 Summary of condition and residue parameters of hydrometallurgy processes of waste LIBs investigated in the literature
Spent materials |
Method |
Residue |
Efficiency |
Purity |
Condition |
Ref. |
LiCoO2 |
Precipitation |
Li2SO4 |
90% |
— |
• 4 M H2SO4, H2O2 [80 °C/4 h] |
77
|
• Ethanol, LiOH |
LIB scraps |
Precipitation |
LiF |
50% |
>99 wt% |
• 500 °C/5 h, KHSO4 |
78
|
• 9 M H2SO4, 30 wt% H2O2 (3 mL g−1) [90–100 °C] |
• 6 M NaOH |
Cathode materials |
Precipitation |
Li2CO3 |
80 ± 1% |
96.97% |
• 4 M HCl, [80 °C] (20 g L−1) |
79
|
• Na2CO3 [100 °C] |
Cathode materials |
Precipitation |
Li2CO3 |
71% |
— |
• 2 M H2SO4, 2% H2O2 (33g L−1) [60 °C] |
80
|
• Na2CO3 [50 °C] |
Mixed cathode materials |
Precipitation |
Li2CO3 |
80% |
— |
• 4 M H2SO4, 30 wt% H2O2 (50 g L−1) [70–80 °C] |
81
|
• NaOH, Na2CO3 [40 °C] |
LiNi0.3Mn0.3Co0.3O2 |
Precipitation |
Li2CO3 |
Leaching 99.7% |
— |
• 3.0 M trichloroacetic acid, 4.0 vol% H2O (50 g L−1) [70–80 °C] |
82
|
• Saturated Na2CO3 solution |
Cathode materials |
Precipitation |
Li3PO4 |
89% |
— |
• Citric acid + H2O2 |
83
|
• C4H8N2O2, (NH4)2C2O4, D2EHPA [55 °C] |
• 0.5 M Na2PO4 |
LiNi0.3Mn0.3Co0.3O2 |
Precipitation |
Li2CO3 |
98.22% |
99.9% |
• 2 M formic acid, 2 vol% H2O2 (50 g L−1) [70 °C] |
84
|
• Saturated Na2CO3 [20–60 °C] |
LiFePO4 |
Precipitation |
Li3PO4 |
82.55% |
96.5% |
• Ball mill with EDTA–2Na, 0.6 M H3PO4 (50 g L−1) |
85
|
Cathode material + graphite |
Precipitation |
Li2CO3 |
Leaching 99.9% |
99.4% |
• 3 M acetic acid, 7.5%vol H2O2 (20 g L−1) [70 °C/40 min] |
86
|
• Saturated Na2CO3 |
LiFePO4 |
Precipitation |
Li2CO3 |
95.05% |
99.95 wt% |
• 0.8 M CH3COOH, 6 vol% H2O2 (120 g L−1) [50 °C/30 min] |
87
|
• Saturated Na2CO3 [95 °C] |
Cathode materials |
Precipitation |
Li2CO3 |
>90% |
99.93% |
• 3.5 M acetic acid (40 g L−1), H2O2 4vol% [60 °C] |
88
|
• Saturated Na2CO3 [20–60 °C] |
Cathode materials |
Precipitation |
Li3PO4 |
85.56% |
— |
• Oxidation at 600 °C, 0.28 M H2SO4 + H2O2 (16 g L−1) [85 °C/2 h] |
89
|
• NaOH, Na3PO4 |
Anode |
Precipitation |
Li2CO3 |
Leaching ≈ 100% |
>99% |
• 1.5 M HCl (100 g L−1) |
91
|
• CO2 |
LiNi0.3Mn0.3Co0.3O2 |
Precipitation |
Li2CO3 |
Leaching 99% |
— |
• 2 M H2SO4 + 4 vol% H202 (50g L−1) [50 °C/2 h] |
90
|
• KMnO4, C4H8N2O2 |
• Na2CO3 [90 °C] |
LiNi0.5Mn0.3Co0.2O2 |
Precipitation |
Li2CO3 |
76% |
>99.5% |
• 1 M of oxalic acid (10g L−1) [95 °C/12 h] |
92
|
• 5 M K2CO3 [80 °C/4 h] |
LiFePO4 |
Precipitation |
Li2CO3 |
99.35% |
— |
• Ball mill with citric acid, H2O2 (20 g g−1) |
93
|
• Saturated Na2CO3 [95 °C] |
Cathode |
Precipitation |
Li2CO3 |
38% |
99.48% |
• 1 M H2SO4, 5 vol% H2O2 [60 °C/1 h], sonication |
94
|
• 2 M NaOH |
LiFePO4 |
Precipitation |
Li2CO3 |
80% |
— |
• 4 M MSA acid (80 g L−1) 18%, H2O2 |
95
|
• 5% NaOH solution, 30% Na2CO3 [96 °C/30 min] |
LiNi0.5Co0.2Mn0.3O2 |
Precipitation |
Li2CO3 |
91.23% |
99% |
• Sodium persulfate (400 g L−1) [85 °C] |
96
|
• Na2CO3 |
LiNixMnyCo1−x−yO2 |
Precipitation |
Li3PO4 |
Leaching 99.1% |
— |
• 750 °C/3 h, 2.75 M H3PO4 [40 °C/10 min] |
97
|
• 10 M NaOH |
LIB scraps |
Solvent extraction |
Li2CO3 |
80% |
>98% |
• 4 M H2SO4 (100 g L−1) glucose [90 °C] |
99
|
• D2HEPA, CYANEX 272, saturated Na2CO3 |
LiCoO2 |
Solvent extraction |
Li2SO4 Solution |
Leaching 100% |
>99.5% |
• 600 °C/2 h, 2.25 M H2SO4 [80 °C/30 min] |
71
|
• PC88A |
LiNi0.3Mn0.3Co0.3O2 |
Solvent extraction |
Li2CO3 |
Leaching 94% |
— |
• 2 M H2SO4 H2O2 (33 g L−1) [70 °C] |
100
|
• Cyanex 272 |
• Saturated Na2CO3 |
LIB scraps |
Solvent extraction |
Li2CO3 |
72% |
99.7% |
• H2SO4–H2O2 mixture |
101
|
• NaOH, D2EHPA, Cyanex 272, kerosene, Na2CO3 [95 °C] |
LiCoO2 |
Solvent extraction |
Li2CO3 |
86.2% |
74.2% |
• 0.5 M HCl [60 °C] |
102
|
• Cyphos IL-101, saturated Na2CO3 [60 °C] |
LiNi0.5Co0.2Mn0.3O2 |
Selective adsorption |
Li2CO3 |
Leaching 81.2% |
>99.5% |
• NH3·H2O (367.5 g L−1) + NH4HCO3 (140 g L−1) + H2O2 (63.24 g L−1) [manganese type lithium ion-sieves |
103
|
• 0.5 M HCl leaching (10 g L−1) |
• NaOH, Na2CO3 |
4.3 Electrochemical extraction
Electrochemical extraction methods separate lithium from pre-treated active materials using a lithium-selective transmissive membrane. This method extracts lithium from the powder state by putting the active material powder from the pre-treated waste lithium-ion battery in water and separating the lithium using a Li-ion conductive ceramic solid electrolyte. When the pre-treated active material is placed in water, the lithium in the powdered cathode does not dissolve, whereas lithium in the anode dissolves in water to form LiOH(aq). During charging, the dissolved and undissolved lithium can be separated via the solid ceramic electrolyte to form lithium metal. When charging occurs, the Li+ dissolved in water can be separated by the oxygen evolution reaction (OER) (eqn (8)). | 2LiOH → 2Li+ + 2e− + 1/2O2 + H2O | (8) |
The undissolved Li+ in the cathode powder can be separated by the same reaction as that of delithiation in the cathode material (eqn (9) and (10))
| LiMO2 → Li+ + e− + MO2 (M = Co, Mn, Ni) | (9) |
| LiFePO4 → Li+ + e− + FePO4 | (10) |
The charging potential differs depending on the type of cathode material in the waste. When the active material is placed in water, the aqueous solution becomes a strong base owing to the influence of LiOH. The OER reaction during charging differs in operating voltage depending on the pH, and in strongly basic conditions (pH > 11), the OER reaction occurs below 3.6 V.104 In the case of the LiFePO4 cathode powder, the delithiation reaction potential is 3.5 V, which is lower than the voltage of the OER reaction in the strong base, and Li+ is directly extracted from the cathode powder. However, for LiCoO2 (3.9 V), LiMn2O4 (4.0 V), and LiNi0.3Mn0.3Co0.3O2 (3.7 V), because the delithiation potential is higher than that of the strong base OER reaction, Li+ extraction from the solution occurs first, rather than from the cathode powder. However, during charging, the pH of the aqueous solution decreases because of the characteristics of the OER reaction, and the operating voltage of the OER reaction gradually increases. When the potential of the OER reaction exceeds that of the cathode powder material, Li+ extraction from the powder becomes possible. Through this OER reaction and the reaction for each cathode material, Li+ can be extracted and separated from both solution and powder, and when Li+ can no longer be extracted, the charging of the system is automatically completed.
After charging, the separated Li+ is discharged to water, passes through another ceramic solid electrolyte, and undergoes an oxygen reduction reaction (ORR) with water and electrons to form an aqueous LiOH solution. LiOH is a strong base that is used for CO2 capture because it is highly reactive with CO2 forming Li2CO3 (Fig. 9). The formation of Li2CO3 can be expressed as follows (eqn (11) and (12)):
| 2Li+ + 2e− + 1/2O2 + H2O → 2LiOH | (11) |
| 2LiOH + 2CO2 → 2Li2CO3 + H2O | (12) |
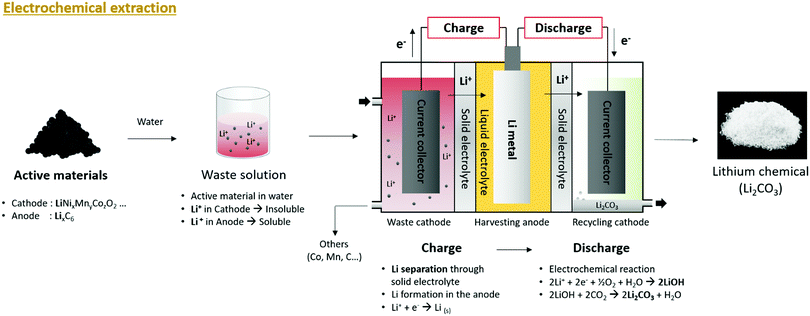 |
| Fig. 9 Overall schematic of lithium recycling from pre-treated waste LIB components by electrochemical extraction process. | |
Electrochemical extraction is a technology that can afford Li2CO3 powder without the need for drying or precipitation. When the system is continuously discharged, Li2CO3 powder precipitates naturally. When discharge occurs, the concentration of Li+ in the water increases gradually, followed by an increase in the concentration of Li2CO3. In addition, owing to the ORR, H2O is consumed and the concentration of Li2CO3 increases relative to the decrease in the quantity of the solvent. This leads to the natural precipitation of Li2CO3.
An electrochemical system was developed to separate lithium from the cathode, anode, and electrolyte from a discarded lithium-ion battery.105 This device uses a Li1+x+yTi2−xAlxP3−ySiyO12 solid electrolyte that transmits lithium selectively and has physically separated liquid cathode and anode parts. The liquid cathode contained LiFePO4, LixC6, and 1 M LiPF6-EC:DMC. Through electrochemical charging, lithium is separated and passed through the solid electrolyte to separate the lithium metal from the anode. In later studies, an advanced system was developed to separate lithium from LiFePO4, LiMn2O4, and LiNi0.3Mn0.3Co0.3O2 cathode powders.106 This system is composed of a waste cathode, harvesting anode, and recycling cathode, and each compartment is physically separated by a solid electrolyte. In the actual electrochemical system, a polymer film was used as the body of the system, solid electrolytes were attached to both sides, and an anode was placed in the middle to produce a harvesting anode. A harvesting anode was inserted between the waste cathode and recycling cathode to separate the battery into three compartments (Fig. 10a). The fabricated electrochemical lithium extraction system can extract lithium through electrochemical charging and discharging, as shown in Fig. 10b. The cathode powder from the lithium-ion battery contacted the waste cathode current collector of the waste cathode. During electrochemical charging, Li+ extraction from the cathode powder occurs, passing through the solid electrolyte, followed by an electrochemical reaction at the harvesting anode to form metallic lithium (Fig. 10c). By discharging the separated lithium from the harvesting anode to the distilled water, Li2CO3 can be formed via a reaction with water, oxygen, and CO2 (Fig. 10c). Actual discarded lithium-ion batteries were tested using this system and it was proved that Li2CO3 can be recycled.
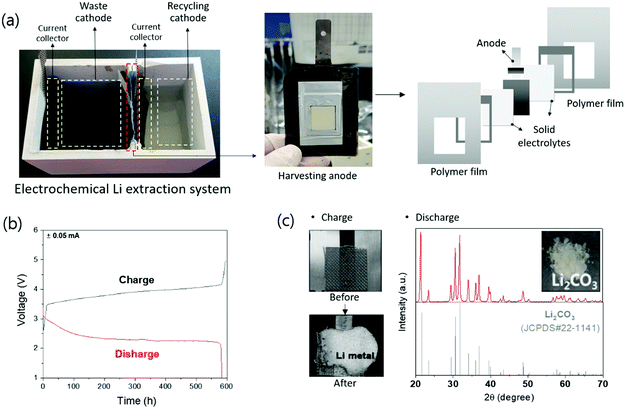 |
| Fig. 10 (a) Prismatic design of electrochemical Li-extraction process. (b) Charge and discharge voltage profile during Li-extraction. (c) Li metal formation after charging and Li2CO3 formation after discharging. | |
The electrochemical extraction method can extract 75–95% of lithium from active material powder during recycling, and it can extract not only Li2CO3 compounds but also lithium metal if necessary. The recycled lithium metal was analyzed using inductively coupled plasma analysis and showed a purity of 99%. In an additional experiment, the purity of the final material, Li2CO3, was analyzed via the acid–base titration method (Standardization Administration of the People's Republic of China (SAC) method), and the purity was 99.6%.
This method only requires charging and discharging and does not require additional chemical compounds or an increase in temperature. With this system, lithium-ion batteries can be recycled in an environment-friendly manner using only water. In addition, by using a special solid electrolyte that allows only lithium to pass through, high-purity lithium can be separated and be used as an energy storage system. However, this method requires the setting up of an electrochemical system and solid electrolyte and has only been proven on a laboratory scale; further research is required before it can be scaled up for commercialization. Table 6 lists the research methods using electrochemical extraction. To scale up the system, research is being conducting to increase the efficiency and speed of Li extraction by designing a cell structure, expanding the area of the solid electrolyte and stacking the system. In addition, since this electrochemical lithium extraction method can extract lithium from LiOH, as well as other lithium solutions in any form, studies are being conducted not only on Li-ion batteries but also on Li-containing glasses.
Table 6 Summary of condition and residue parameters of electrochemical recycling processes of waste LIBs investigated in the literature
Spent materials |
Residue |
Efficiency |
Purity |
Condition |
Ref. |
LiFePO4, C6, LiPF6 |
Li metal |
— |
— |
Current 0.1 mA cm−2 |
105
|
Cathode powders, LIB scraps |
Li metal, Li2CO3 |
75–92% |
99% (metal) |
Current 0.05 mA |
106
|
Three methods to produce lithium compounds from a pre-treated lithium-ion battery have been discussed: pyrometallurgy, hydrometallurgy, and electrochemical extraction. These techniques are still under research, and the methods can be combined to overcome their respective drawbacks.
Table 7 lists quantitative metrics on the pyrometallurgy, hydrometallurgy, and electrochemical lithium recycling methods. These metrics include the recycling efficiency, additional cost of chemicals, operating temperature, complexity, pollutant production, and commercialization level. The methods only consider the lithium extraction process after pre-treatment, as the pre-treatment methods can vary depending on the lithium-ion battery type and quantity. The lithium recycling efficiency and purity is obtained from available literature regarding the methods. For the additional cost of chemicals, the price was normalized per kilogram of discarded active material powder, after considering the required amount and price.107–109 Operating temperature considers the temperature range needed for lithium extraction. For complexity, the essential steps for each method are examined. Pollutants include different polluting materials that can result from each recycling process, and the level of commercialization separates the methods that already have been commercialized and methods that are only developed at a laboratory level.
Table 7 Quantitative calculation table for each factors of lithium recycling technologies from waste LIBs
Li recycling method |
Recycle efficiency (%) |
Purity (%) |
Additional chemical cost ($ kg−1) |
Temperature (°C) |
Complexity |
Potential pollutions |
Commercial readiness |
Pyrometallurgy |
80–98.9 |
98–99.95 |
1.894 m−3 (flowing gas (N2), depends on furnace size) |
650–1700 |
2 Steps |
Volatile organic compounds |
Commercialized [Accurec GmbH] |
• Oxygen free roasting |
• Wet magnetic separation |
Hydrometallurgy [precipitation] |
76–98.2 |
96.5–99.97 |
3.18 (CH2O2, H2O2etc.) |
50–100 |
3 Steps |
Inorganic waste |
Commercialized [TOXCO (Retriev)] |
• Leaching |
Acidic waste |
• Other metal precipitation (pH adjustment) |
• Li precipitation |
Hydrometallurgy [solvent extraction] |
72–86.2 |
74.2–99.7 |
73.3 (H2SO4, PC88A etc.) |
60–90 |
4 Steps |
Inorganic waste |
On research |
• Leaching |
Organic waste |
• Solvent extraction |
Acidic waste |
• Solution separation |
|
• Precipitation |
|
Hydrometallurgy [selective adsorption |
Leaching 81.2 |
>99.5 |
14.9 (NH3, HCl etc. |
30 |
4 Steps |
Acidic waste |
On research |
• Leaching |
• Sieve adsorption |
• Sieve leaching |
• Precipitation |
Electrochemical extraction |
75–92 |
>99 (metal) |
— |
Room temp. |
2 Steps |
— |
On research |
• Charging |
• Discharging |
Fig. 11 is based on Table 7 and describes the characteristics of each method using a spider plot. The image shows the relative magnitudes of the different metrics involved in lithium extraction.
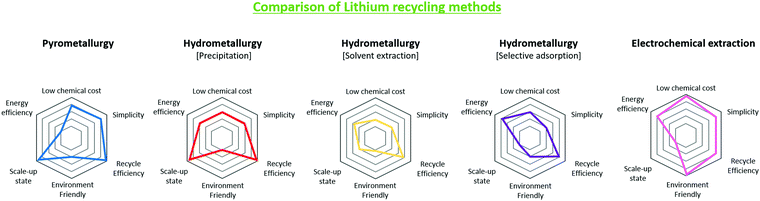 |
| Fig. 11 Performance and industrial comparisons of lithium recycling technologies from waste LIBs, pyrometallurgy, hydrometallurgy and electrochemical extraction. | |
5. Current state of lithium recycling
With the dawn of the era of Battery of Things (BoT), emerging applications other than EVs and ESSs are causing a significant hike in the demand for lithium-ion batteries. The growth of the markets has also resulted in a steep increase in the quantity of discarded lithium-ion batteries. As lithium resources are limited, this trend is expected to cause a shortage of lithium in the near future. This has led nations to create policies to regulate lithium-ion battery disposal, and research institutes and companies, aligning with policy interests, are focusing on lithium recycling technology. Worldwide, companies such as Umicore, Sumitomo-sony, DK recycling und Roheisen GmbH, and SNAM, recycle batteries using the pyrometallurgy and hydrometallurgy methods explained above.110,111 However, these companies have focused on recycling Co and Ni, rather than lithium. Conversely, Accurec and Retriev (TOXCO) use pyrometallurgy and hydrometallurgy methods to produce lithium compounds from disposed lithium-ion batteries.
Accurec pre-treats the battery mechanically to separate the plastic, followed by vacuum thermal treatment to remove the electrolyte and solvent. The material is then crushed, sieved, and separated (magnetically and with air) to separate Al, Fe, Cu, and plastic. The pre-treated material then undergoes pyrometallurgy treatments, namely, reduction to separate Co and Mn, followed by hydrometallurgy and acid leaching to precipitate LiCl.112
Retriev's pre-treatment processes include the use of liquid nitrogen in the cryo-milling process (at −196 °C) followed by the use of a hammer mill to crush the material. Subsequently, Cu, Co, and Al were separated on a shaker table, and the remaining cathode and anode material were processed in a filter tank and carbon filter press to remove the metal oxide and carbon. Na2CO3 was added to the solution to precipitate Li2CO3.113,114
The last decade has seen a global rise in the interest in lithium recycling. However, issues remain regarding the means to commercialize and make the process more environmentally friendly. According to the UNEP report on recycling rates, the lithium-ion battery recycling rate in the EU is less than 5%, and less than 1% of lithium is recycled.115–118
6. Future directions for lithium recycling technologies
Given these circumstances, each method of lithium recycling must be developed for commercialization to create a better and more sustainable lithium cycle. Research and development in this field must take the directions indicated below for a more vibrant lithium recycling market (Fig. 12):
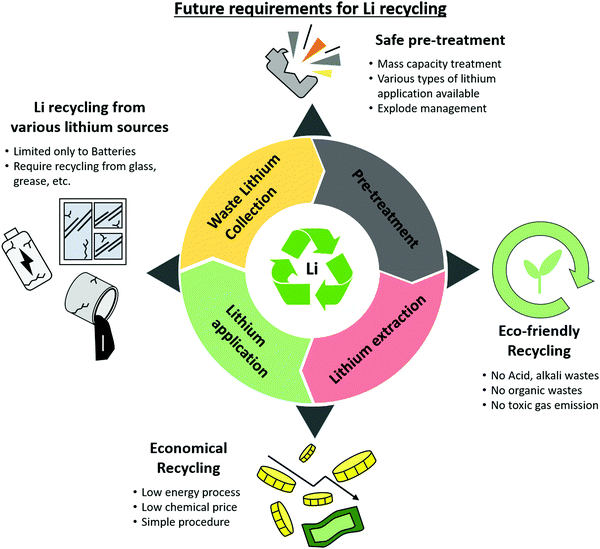 |
| Fig. 12 Future requirements for waste LIB recycling: recycling from various lithium sources, safe treatment, eco-friendly recycling, economical recycling. | |
(1) Although the increase in the demand for lithium is due to lithium-ion batteries, technological development to extract lithium from other products is needed. There is almost no research on the extraction of lithium from non-batteries. Because 35% of the lithium is used for glass, grease, and casting (as of 2019), more attention should be paid to the recycling of lithium from these products. The best option could be to leverage the technologies developed for recycling lithium-ion batteries to extract lithium from other waste lithium sources. Overall, this positively affects the entire lithium cycle.
(2) Even if lithium materials are collected for recycling in an organized manner, it is difficult to process them simultaneously. For batteries, the materials for the cathode, anode, and electrolytes, as well as the size of the batteries, vary widely. Processing them simultaneously holds the risk of problems such as explosions. A policy is needed to categorize or automatically process the disposed lithium material. In addition, it is important to pre-treat lithium-containing materials to increase the efficiency of lithium recycling. With a safe and environmentally friendly pre-treatment method, considerable quantities of lithium materials can be processed to significantly increase the speed of the lithium cycle.
(3) Currently, the techniques that are widely commercialized or researched are also environmentally harmful. Lithium recycling technology should not only mitigate lithium scarcity but also reduce waste to prevent environmental pollution. However, most currently developed recycling methods produce pollutants. This includes harmful gases created by the heat treatment of lithium waste and the acids, bases, and organic solvents used for leaching. This also leads to additional expenses. The extra costs and procedures can hinder the commercialization of the process. Thus, substantial consideration should be given to streamlining the process to minimize waste products or finding ways to reuse the waste products from these processes.
(4) For such a process, economics cannot be ignored. If the purchasing cost is lower than the recycling cost, recycling would not be economically appropriate or viable. Current methods that use acids or bases or operate at high temperatures are usually costly and energy-intensive. The involvement of more chemicals in the process will increase the cost of the process. The quantities of acids and bases required for lithium recycling are substantially greater than that of the recycled product, which is economically disadvantageous. Thus, methods to reuse the acids and bases, or to lower the operating temperature, must be developed to minimize the cost and energy required to recycle lithium. If an economically viable method emerges, this will accelerate interest in the development of lithium recycling technologies.
7. Conclusions
These future efforts will allow lithium to be an eco-friendlier and economical driving force for future technologies by creating a more natural recycling cycle, similar to those of other elements. In addition to the development of lithium recycling technologies, global awareness regarding lithium scarcity is important, and perspectives of sellers, developers, and recycling agents in this matter should be improved. Moreover, government-level policy interests and support are crucial for a more sustainable lithium economy.
Conflicts of interest
There are no conflicts to declare.
Acknowledgements
This work was supported by the Human Resources Program in Energy Technology of the Korea Institute of Energy Technology Evaluation and Planning (KETEP), which was granted financial resources from the Ministry of Trade, Industry & Energy, Republic of Korea (20194010201890). Also, this material is based upon work supported by the Ministry of Trade, Industry & Energy (MOTIE, Korea) under Industrial Technology Innovation Program. No. 20003877, ‘Development of eco-friendly electrochemical recycling system for production of high purity (>99.5%) lithium and lithium compounds.’
Notes and references
- H. Tong, Q. Zhou, B. Zhang, X. Wang, Y. Yao, Z. Ding, H. Chen, J. Zheng and W. Yu, Eng. Sci., 2019, 8, 25 Search PubMed.
- R. Li, C. Lin, N. Wang, L. Luo, Y. Chen, J. Li and Z. Guo, Adv. Compos. Hybrid Mater., 2018, 1, 440 CrossRef CAS.
- C. Hou, B. Wang, V. Murugadoss, S. Vupputuri, Y. Chao, Z. Guo, C. Wang and W. Du, Eng. Sci., 2020, 11, 19 CAS.
- M. Idrees, L. Liu, S. Batool, H. Luo, J. Liang, B. Xu, S. Wang and J. Kong, Eng. Sci., 2019, 6, 64 Search PubMed.
- Q. Zhang, J. Dai, M. Liao, T. Duan and W. Yao, Eng. Sci., 2019, 7, 43 Search PubMed.
- C. Hou, J. Hou, H. Zhang, Y. Ma, X. He, W. Geng and Q. Zhang, Eng. Sci., 2020, 11, 36 CAS.
- C. Hou, W. Yang, X. Xie, X. Sun, J. Wang, N. Naik, D. Pan, X. Mai, Z. Guo, F. Dang and W. Du, J. Colloid Interface Sci., 2021, 596, 396 CrossRef CAS PubMed.
- Sociedad Química y Minera de Chile S.A., Annual Report 2019, 2020.
-
M. Garside, Total global consumption of lithium from 2008 to 2016, 2019 Search PubMed.
- Lithium resources, http://chem230.wikia.com/wiki/Lithium_Resources (accessed on 05 April 2021).
- H. Vikström, S. Davidsson and M. Höök, Appl. Energy, 2013, 110, 252 CrossRef.
-
M. Garside, Distribution of lithium end-usage worldwide in 2019 by area of application, 2020, https://www.statista.com/statistics/268787/lithium-usage-in-the-world-market/ (accessed on 05 April 2021) Search PubMed.
- P. W. Gruber, P. A. Medina, G. A. Keoleian, S. E. Kesler, M. P. Everson and T. J. Wallington, J. Ind. Ecol., 2011, 15(5), 760 CrossRef.
- TALISON LITHIUM, Lithium, https://www.talisonlithium.com/lithium (accessed on 05 April 2021.
-
G. E. Totten, S. R. Westbrook and R. J. Shah, Fuels and lubricants handbook: technology, properties, performance, and testing, ASTM Int., 2003, ch. 22, p. 559 Search PubMed.
- Y. Q. Lu, G. D. Zhang, M. F. Jiang, H. X. Liu and T. Li, Mater. Sci. Forum, 2011, 675, 877 Search PubMed.
-
R. K. Bansal, 1996, 192, ISBN 978-0-7637-0665-4.
- P. Meshram, B. D. Pandey and T. R. Mankhand, Hydrometallurgy, 2014, 150, 192 CrossRef CAS.
- G. Liu, Z. Zhao and A. Ghahreman, Hydrometallurgy, 2019, 187, 81 CrossRef CAS.
-
J. F. Labbé and G. Daw, Panorama 2011 du marché du lithium; Rapport public 2012, BRGM/RP-61340-FR, BRGM, Orléans, France, 2012 Search PubMed.
- S. H. Mohr, G. M. Mudd and D. Giurco, Minerals, 2012, 2, 65 CrossRef.
-
D. E. Garrett, Handbook of Lithium and Natural Calcium Chloride: Their Deposits, Processing, Uses and Properties, 1st edn, Elsevier, 2004 Search PubMed.
- A. Yaksic and J. E. Tilton, Resour. Policy, 2009, 34, 185 CrossRef.
- P. Chen, S. Tang, H. Yue, C. Liu, C. Li and B. Liang, Ind. Eng. Chem. Res., 2017, 56, 5668 CrossRef CAS.
-
W. C. Bauman and J. L. Burba, Google Patents, US6280693B1, 2001 Search PubMed.
- X. Liu, M. Zhong, X. Chen and Z. Zhao, Hydrometallurgy, 2018, 176, 73 CrossRef CAS.
- Saltworks, Lithium Brine Extraction Technologies & Approaches, June 8th, 2018, https://www.saltworkstech.com/articles/lithium-brine-extraction-technologies-and-approaches/ (accessed on 05 April 2021).
- Roskill Information Services Ltd., “The Economics of Lithium”, Eleventh Edition, 2009.
- Y. Zhang, Y. Hu, L. Wang and W. Sun, Miner. Eng., 2019, 139, 105868 CrossRef CAS.
-
K. Pennell, How Does Lithium Mining Work? 2018, https://blog.grabcad.com/blog/2018/02/20/how-does-lithium-mining-work/ (accessed on 05 April 2021).
- V. Flexer, C. F. Baspineiro and C. I. Galli, Sci. Total Environ., 2018, 639, 1188 CrossRef CAS PubMed.
- R. F. Service, Seawater could provide nearly unlimited amounts of critical battery material, Science, 2020 Search PubMed , https://www.sciencemag.org/news/2020/07/seawater-could-provide-nearly-unlimited-amounts-critical-battery-material/ (accessed on 30 April 2021).
-
M. Garside, Average lithium carbonate price from 2010 to 2019 (in U.S. dollars per metric ton), 2020, https://www.statista.com/statistics/606350/battery-grade-lithium-carbonate-price/ (accessed on 05 April 2021) Search PubMed.
- Lithium-Ion Battery Market by Type, Power Capacity, Industry, Voltage, Region-Global Forecast to 2025, 2020.
- Y. Ding, Z. P. Cano, A. Yu, J. Lu and Z. Chen, Electrochem. Energy Rev., 2019, 2, 1 CrossRef CAS.
- How much Lithium is in a Li-Ion Vehicle Battery, 2017, https://www.linkedin.com/pulse/how-much-lithium-li-ion-vehicle-battery-paul-martin/ (accessed on 05 April 2021).
- U.S. Geological Survey, Mineral Commodity Summaries, January 2020.
- Altura Lithium, New Significant Supply to the Battery market in 2018, 2018, 32, https://alturamining.com/wp-content/uploads/2018/07/2018-06-27-Investor-Pres-June-2018-updated.pdf/, (accessed on 30 April 2021) Search PubMed.
- Projection of total worldwide lithium demand from 2018 to 2025 (in 1000 metric tons of lithium carbonate equivalent), 2019.
-
C. Admin, How Often Should I Charge My Phone To Prolong The Battery Life? 2020, https://charbycharge.com/how-often-should-i-charge-my-phone-to-prolong-the-battery-life/ (accessed on 05 April 2021).
- P. Haidl, A. Buchroithner, B. Schweighofer, M. Bader and H. Wegleiter, Sustainability, 2019, 11, 6731 CrossRef CAS.
-
J. Svarc, Lead-Acid Vs Lithium-Ion Batteries, 2019, https://www.cleanenergyreviews.info/blog/simpliphi-pylontech-narada-bae-lead-acid-battery (accessed on 05 April 2021) Search PubMed.
- Lead Acid Battery working – Lifetime Study, http://www.power-thru.com/documents/The%20Truth%20About%20Batteries%20-%20POWERTHRU%20White%20Paper.pdf (accessed on 05 April 2021).
- Features of sealed lead acid batteries, https://www.power-sonic.com/blog/features-of-sealed-lead-acid-batteries/ (accessed on 04.05.2021).
- D. L. Thompson, J. M. Hartley, S. M. Lambert, M. Shiref, G. D. J. Harper, E. Kendrick, P. Anderson, K. S. Ryder, L. Gaines and A. P. Abbott, Green Chem., 2020, 22, 7585 RSC.
-
L. An, Recycling of Spent Lithium-Ion Batteries, Processing Methods and Environmental Impacts, 2019 Search PubMed.
- M. Jacoby, Chem. Eng., 2019, 97(28) Search PubMed , https://cen.acs.org/materials/energy-storage/time-serious-recycling-lithium/97/i28 (accessed on 05 April 2021).
-
C. Pillot, Presented at the 31st International Battery Seminar & Exhibit, Fort Lauderdale, FL, USA, 2017.
-
D. Hauck and M. Kurrat, Overdischarging Lithium-Ion Batteries, Springer, 2018 Search PubMed.
-
A. Kwade and J. Diekmann, Recycling of Lithium-Ion Batteries; The LithoRec Way, Sustainable Production, Life Cycle Engineering and Management, 2018, ch. 4, p. 53 Search PubMed.
- J. S. Stewart, A. A. Reguera, A. Greszta, J. Marco, M. Masood, R. Sommerville and E. Kendrick, Sustainable Mater. Technol., 2019, 22, e00110 CrossRef.
- T. Zhang, Y. He, F. Wang, L. Ge, X. Zhu and H. Li, Waste Manage., 2014, 34, 1051 CrossRef CAS PubMed.
- D. A. Bertuol, C. Toniasso, B. M. Jimenez, L. Meili, G. L. Dotto, E. H. Tanabe and M. L. Aguiar, J. Power Sources, 2015, 275, 62 CrossRef.
- F. Pagnanelli, E. Moscardini, P. Altimari, T. A. Atia and L. Toro, Waste Manage., 2016, 51, 214 CrossRef CAS PubMed.
- X. Wang, G. Gaustad and C. W. Babbitt, Waste Manage., 2016, 51, 204 CrossRef CAS PubMed.
- F. Pagnanelli, E. Moscardini, P. Altimari, T. A. Atia and L. Toro, Waste Manage., 2017, 60, 706 CrossRef CAS PubMed.
- Y. Zhang, Y. He, T. Zhang, X. Zhu, Y. Feng, G. Zhang and X. Bai, J. Cleaner Prod., 2018, 202, 736 CrossRef CAS.
- L. Li, J. Ge, R. Chena, F. Wua, S. Chen and X. Zhang, Waste Manage., 2010, 30, 2615 CrossRef CAS PubMed.
- X. Chen and T. Zhou, Waste Manage. Res., 2014, 32(11), 1083 CrossRef PubMed.
- L. P. He, S. Y. Sun, X. F. Song and J. G. Yu, Waste Manage., 2015, 46, 523 CrossRef CAS PubMed.
- X. Chen, C. Luo, J. Zhang, J. Kong and T. Zhou, ACS Sustainable Chem. Eng., 2015, 3, 3104 CrossRef CAS.
- L. Sun and K. Qiu, Waste Manage., 2012, 32, 1575 CrossRef CAS PubMed.
- P. Meshram, B. D. Pandey and T. R. Mankhand, Chem. Eng. J., 2015, 281, 418 CrossRef CAS.
- C. Hanisch, T. Loellhoeffel, J. Diekmann, K. J. Markley, W. Haselrieder and A. Kwade, J. Cleaner Prod., 2015, 108, 301 CrossRef CAS.
- Y. Yang, G. Huang, S. Xu, Y. He and X. Liu, Hydrometallurgy, 2016, 165, 390 CrossRef CAS.
- T. G. Maschler, B. Friedricha, R. Weyhe, H. Heegn and M. Rutz, J. Power Sources, 2012, 207, 173 CrossRef.
- J. Li, G. Wang and Z. Xu, J. Hazard. Mater., 2016, 302, 97 CrossRef CAS PubMed.
- J. Xiao, J. Li and Z. Xu, Environ. Sci. Technol., 2017, 51, 11960 CrossRef CAS PubMed.
- J. Xiao, J. Li and Z. Xu, J. Hazard. Mater., 2017, 338, 124 CrossRef CAS PubMed.
- J. Hu, J. Zhang, H. Li, Y. Chen and C. Wang, J. Power Sources, 2017, 351, 192 CrossRef CAS.
- H. Dang, B. Wang, Z. Chang, X. Wu, J. Feng, H. Zhou, W. Li and C. Sun, ACS Sustainable Chem. Eng., 2018, 6, 13160 CrossRef CAS.
- S. Pindar and N. Dhawan, J. Oper. Manage., 2019, 71, 4483 CAS.
- C. Pengb, F. Liu, Z. Wang, B. P. Wilson and M. Lundström, J. Power Sources, 2019, 415, 179 CrossRef.
- S. Maroufi, M. Assefi, R. Khayyam and V. Sahajwalla, Sustainable Mater. Technol., 2020, 23, e00139 CrossRef CAS.
- S. Pindar and N. Dhawan, J. Therm. Anal. Calorim., 2020 DOI:10.1007/s10973-020-10139-6.
- J. Lin, L. Li, E. Fan, C. Liu, X. Zhang, H. Cao, Z. Sun and R. Chen, ACS Appl. Mater. Interfaces, 2020, 12, 18482 CrossRef CAS PubMed.
- S. Aktas, D. J. Fray, O. Burheim, J. Fenstad and E. Açma, Metallurgy, 2006, 115(2), 95 CAS.
- J. F. Paulino, N. G. Busnardo and J. C. Afonso, J. Hazard. Mater., 2008, 150, 843 CrossRef CAS PubMed.
- R. C. Wang, Y. C. Lin and S. H. Wu, Hydrometallurgy, 2009, 99, 194 CrossRef CAS.
- S. Zhu, W. He, G. Li, X. Zhou, X. Zhang and J. Huang, Trans. Nonferrous Met. Soc. China, 2012, 22, 2274 CrossRef CAS.
- H. Zou, E. Gratz, D. Apelian and Y. Wang, Green Chem., 2013, 15, 1183 RSC.
- X. Zhang, H. Cao, Y. Xie, P. Ning, H. An, H. You and F. Nawaz, Sep. Purif. Technol., 2015, 150, 186 CrossRef CAS.
- X. Chen, T. Zhou, J. Kong, H. Fang and Y. Chen, Sep. Purif. Technol., 2015, 141, 76 CrossRef CAS.
- W. Gao, X. Zhang, X. Zheng, X. Lin, H. Cao, Y. Zhang and Z. Sun, Environ. Sci. Technol., 2017, 51, 1662 CrossRef CAS PubMed.
- Y. Yang, X. Zheng, H. Cao, C. Zhao, X. Lin, P. Ning, Y. Zhang, W. Jin and Z. Sun, ACS Sustainable Chem. Eng., 2017, 5, 9972 CrossRef CAS.
- S. Natarajan, A. B. Boricha and H. C. Bajaj, Waste Manage., 2018, 77, 455 CrossRef CAS PubMed.
- Y. Yang, X. Meng, H. Cao, X. Lin, C. Liu, Y. Sun, Y. Zhang and Z. Sun, Green Chem., 2018, 20, 3121 RSC.
- W. Gao, J. Song, H. Cao, X. Lin, X. Zhang, X. Zheng, Y. Zhang and Z. Sun, J. Cleaner Prod., 2018, 178, 833 CrossRef CAS.
- S. Tao, J. Li, L. Wang, L. Hu and H. Zhou, Ionics, 2019, 25, 5643 CrossRef CAS.
- R. Sattar, S. Ilyas, H. N. Bhatti and A. Ghaffar, Sep. Purif. Technol., 2019, 209, 725 CrossRef CAS.
- Y. Yang, S. Song, S. Lei, W. Sun, H. Hou, F. Jiang, X. Ji, W. Zhao and Y. Hu, Waste Manage., 2019, 85, 529 CrossRef CAS PubMed.
- Q. Li, K. Y. Fung, L. Xu, C. Wibowo and K. M. Ng, Ind. Eng. Chem. Res., 2019, 58, 3118 CrossRef CAS.
- L. Li, Y. Bian, X. Zhang, Y. Yao, Q. Xue, E. Fan, F. Wu and R. Chen, Waste Manage., 2019, 85, 437 CrossRef CAS PubMed.
- C. H. Jo and S. T. Myung, J. Power Sources, 2019, 426, 259 CrossRef CAS.
- P. Yadav, C. J. Jie, S. Tan and M. Srinivasan, J. Hazard. Mater., 2020, 399, 123068 CrossRef CAS PubMed.
- W. Lv, Z. Wang, X. Zheng, H. Cao, M. He, Y. Zhang, H. Yu and Z. Sun, ACS Sustainable Chem. Eng., 2020, 8(13), 5165 CrossRef CAS.
- Y. Zhang, W. Wang, J. Hu, T. Zhang and S. Xu, ACS Sustainable Chem. Eng., 2020, 8, 15496 CrossRef CAS.
- P. Zhang, T. Yokoyama, O. Itabashi, T. M. Suzuki and K. Inoue, Hydrometallurgy, 1998, 47, 259 CrossRef CAS.
- G. Granata, E. Moscardini, F. Pagnanelli, F. Trabucco and L. Toro, J. Power Sources, 2012, 206, 393 CrossRef CAS.
- W. S. Chen and H. J. Ho, Metals, 2018, 8, 321 CrossRef.
- T. A. Atia, G. Elia, R. Hahn, P. Altimari and F. Pagnanelli, J. Energy Chem., 2019, 35, 220 CrossRef.
- L. Xu, C. Chen and M. L. Fu, Hydrometallurgy, 2020, 197, 105439 CrossRef CAS.
- H. Wang, K. Huang, Y. Zhang, X. Chen, W. Jin, S. Zheng, Y. Zhang and P. Li, ACS Sustainable Chem. Eng., 2017, 5, 11489 CrossRef CAS.
- A. G. Rajan and E. A. Carter, Energy Environ. Sci., 2020, 13, 4962 RSC.
- N. M. Asl, S. S. Cheah, J. Salim and Y. Kim, RSC Adv., 2012, 2, 6094 RSC.
- H. Bae, S. M. Hwang, I. Seo and Y. Kim, J. Electrochem. Soc., 2016, 163(7), E199 CrossRef CAS.
- CEIC, https://www.ceicdata.com/ (accessed on 05 April 2021).
- Chemical book, https://www.chemicalbook.com/ (accessed on 05 April 2021).
- ECHEMI.com, https://www.echemi.com/ (accessed on 05 April 2021).
- C. Liu, J. Lin, H. Cao, Y. Zhang and Z. Sun, J. Cleaner Prod., 2019, 228, 801 CrossRef CAS.
-
I. Samarukha, Recycling strategies for End-of-Life Li-ion Batterie from Heavy Electric Vehicles KTH Industrial Engineering and Management, 2020 Search PubMed.
-
C. L. Gabriel, Environmental and Natural Resources Engineering, Luleå University of Technology, Finland, 2016 Search PubMed.
-
L. L. Gaines and J. B. Dunn, Lithium-Ion Batteries: Advances and Applications, Elsevier, 2014, ch. 21, p. 483 Search PubMed.
- A. Sonoc, J. Jeswiet and V. K. Soo, Procedia CIRP, 2015, 29, 752 CrossRef.
- T. E. Graedel, J. Allwood, J. P. Birat, B. K. Reck, S. F. Sibley, G. Sonnemann, M. Buchert and C. Hageluken, Nat. Energy, 2019, 4, 253 CrossRef.
- AReport of Teh Working Group on the Global Metal Flows to the International Resources Panel., United Nations Environment Programme, 2011.
-
D. P. Raphaël, The recycling of lithium-ion batteries A strategic pillar for the European Battery Alliance, Center for Energy, Raphael, 2020 Search PubMed.
- Critical Minerals Today and in 2030: An Analysis for OECD Countries, OECD Environment Working Papers, 2015, No. 91.
Footnote |
† Electronic supplementary information (ESI) available. See DOI: 10.1039/d1ma00216c |
|
This journal is © The Royal Society of Chemistry 2021 |