DOI:
10.1039/D1MA00195G
(Paper)
Mater. Adv., 2021,
2, 4630-4633
Temperature dependent poly(L-lactide) crystallization investigated by Fourier transform terahertz spectroscopy†
Received
5th March 2021
, Accepted 30th May 2021
First published on 2nd June 2021
Abstract
Poly(L-lactide) (PLLA) was investigated by Fourier transform terahertz (THz) spectroscopy over the frequency range of 1.0–8.5 THz. THz absorption spectra were acquired for PLLA samples isothermally crystallized at the temperature of 80–140 °C after melt-quenching. Characteristic PLLA absorption peaks were identified at 1.8, 4.0, 4.7, and 7.1 THz for the 80 °C sample; higher crystallization temperature resulted in a blue shift of the absorption peak frequency. The higher two peaks (4.7 and 7.1 THz), which are present not only in α-form but also in δ-form, were attributed to the intermolecular vibration of the PLLA chain, depending on the lattice spacing and crystallinity. In contrast, the 4.0 THz peak was intrinsically in α-form, thus enabling its use as an indicator in the optimization of PLLA polymorphism. Broadband THz spectroscopy is a nondestructive and noninvasive evaluation technique for biodegradable polymers.
Introduction
Recent advances in the mass-production of polylactide (PLA) from a biomass feedstock have opened the possibility for its role as a renewable resource, specifically as an alternative to petroleum derived polymers.1–4 PLAs have fascinating characteristics such as piezoelectric properties and biodegradability for applications in flexible electronics.5 The piezoelectric constant d of PLA is approximately 7 to 12 pC N−1, which is low compared to that of lead zirconium titanate (PZT). However, PLA exhibits a very low relative dielectric constant of εr (ca. 2.5) compared to that of PZT and polyvinylidene fluoride (PVDF).6,7 Therefore, the piezoelectric output constant g (g = d/εr) is large when compared with that of PZT and PVDF; this reasonable sensitivity makes it a promising piezoelectric polymer material. Since the piezoelectric properties of PLA are governed by its higher-order structure, it is important to clarify the higher-order structure for the development of materials with new functions.
Recently, terahertz (THz) spectroscopy has been recognized as an attractive technique for identifying and characterizing the electrical properties and higher-order structure in polymer science. In particular, bulk properties such as intermolecular vibration and electromagnetic interactions of photons and phonons have been investigated using boson peak analysis in the THz range that are caused by low energy excitation.8 In addition, THz band peak analysis is a powerful method for studying collective excited vibrations along the long polymer chain structure and entanglement.9–12 For instance, H. Li et al. reported the crystal transition of PLA. Their results indicated that the α to α′ form (δ form) transformation is strongly related to a lattice vibration peak located around 2.0 THz, and the peak frequency was related to the lattice spacing derived from the X-ray diffraction (XRD) spectra.13 Most of their measurements used THz time-domain spectroscopy (THz-TDS) to coherently detect the THz electric field. However, this technique has a high dynamic range at low frequencies below ca. 3 THz,14 and the systematic analysis in the region beyond 3 THz is still limited. Fourier transform THz spectroscopy (FT-TS) is an excellent alternative for acquiring data beyond the upper frequency limit. To develop guidelines for the functional development and optimization of the piezoelectric properties of PLA materials, broadband THz analysis will also be crucial, as it reveals the relationship between higher-order structures and functional properties.
In this paper, we first explain the fabrication of poly(L-lactide) (PLLA) samples with various thermal annealing conditions for isothermal crystallization. Next, we introduce the use of broadband THz spectroscopy, which unveils the correlation between PLLA crystalline phase constitution and spectral changes. Finally, we discuss the origin of characteristic THz peaks and the usefulness of broadband THz spectroscopy.
Experimental
Sample preparation
PLA is known to form the PLLA, poly(D-lactide), and their blend (or stereocomplex formation). In this experiment, PLLA samples were fabricated from pellets kindly supplied by commercial companies (UNITIKA Ltd, Japan). The pellets (product ID: 4031DK, number average molecular weight = 1.10 × 105, and polydispersity index = 1.55, and L-lactic acid unit content = 95.2%) were first heated above the melting temperature (ca. 170 °C) and then pressed into a discoid shape with 0.1 mm thickness and ca. 20 mm diameter. After the pellets were completely melted for 90 min (200 °C), the samples were then abruptly immersed in liquid nitrogen (−196 °C) to initialize their thermal history and ensure amorphous structure. The samples were then transferred onto a hot stage pre-set at the required crystallization temperatures (Tc = 80–140 °C) with a 10 °C step. The temperature was maintained for 240 min to complete the isothermal crystallization process, and the samples were again immersed in liquid nitrogen to stop further crystallization.
X-Ray diffraction spectroscopy
XRD profiles were obtained using the conventional 2θ–θ scan method with an X-ray source (Cu-Kα = 0.154 nm) using a RINT-2500 diffractometer (Rigaku Corporation, Japan) in a range of 14–22° with a 0.02° step, scan speed of 4° min−1, and a 40 kV, 200 mA source power.
Terahertz spectroscopy
Broadband (1.0–8.5 THz) THz spectra of the samples were measured using an FT-TS with a frequency resolution of 0.015 THz (0.5 cm−1). The measurements were conducted at room temperature using a FARIS-S spectrometer (JASCO Corporation, Japan) equipped with a deuterated triglycine sulfate detector and a Martin–Puplett interferometer system including two pieces of half-mirrors, which enables a signal that is twice as strong as that obtained using conventional systems with only one half-mirror, see ESI† for further details. The samples were placed horizontally and perpendicular to the THz-wave propagation during the measurements, and the sample and interferometer spaces were evacuated to a pressure of less than 100 Pa to minimize absorption of the THz waves by oxygen gas or water vapor in the atmosphere.
Results and discussion
Absorption spectra
To investigate the relationship between the crystalline process and THz spectral features, spectral measurements were conducted on PLLA samples with different Tc from 80 °C to 140 °C. As a reference, the 80 °C sample was identified with peaks A (1.8 THz), B (4.0 THz), C (4.7 THz), and D (7.1 THz) in the relatively broad frequency range of 1.0–8.5 THz (Fig. 1(a)). A weak and broad peak appeared around 3.2 THz was not analyzed due to the mixture of multiple components. Although peaks B and C were close together, the higher Tc samples seemed to be blue-shifted, as shown in Fig. 1(b). The denser chain packing causes the higher intermolecular vibration frequency. The main origin of the blue-shift from 80 °C to 140 °C is thought to be attributed to the transition of the PLLA crystalline structure (lattice spacing) from δ-form (0.540 nm) to α-form (0.532 nm).15,16 A similar absorption peak around 1.8 THz only was also identified by previous researchers using THz-TDS.13 Our FT-TS measurements expanded the observable range and found new three peaks until 8.5 THz. The frequency shifts of peaks A and B were saturated at higher Tc, while those of peaks C and D gradually increased and did not appear to be saturated even at 140 °C. Especially, peak B seemed to be saturated at lower Tc than the other three peaks. However, no obvious difference was confirmed in peak shift behavior; therefore, further studies were required.
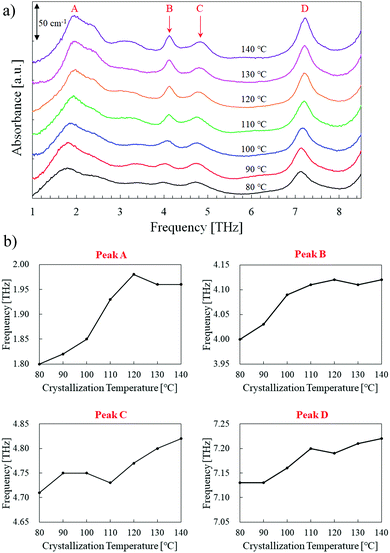 |
| Fig. 1 (a) THz absorption spectra of PLLA for different crystallization temperature. (b) Frequency shifts of the four peaks labeled in (a). | |
Spectral analysis
To quantitatively analyze the spectral change, the peak height and width (full width at half-maximum, FWHM) calculations were conducted on peaks B, C, and D. As peak A was complex by the mixture of multiple components, it was not analyzed. However, the three higher-frequency peaks had relatively simple profiles throughout the isothermal crystallization process. As an example, the peak analysis of the 80 °C sample is shown in Fig. 2. The close peaks B and C were separated by using two Gaussian fitting functions. Before this calculation, a linear baseline was adopted from 3.8 THz (the left edge of the peak B) and 5.5 THz (the valley between peaks C and D). For the single peak D, another linear baseline was employed from 6 THz (the valley between peaks C and D) and 8 THz (the right edge of peak D).
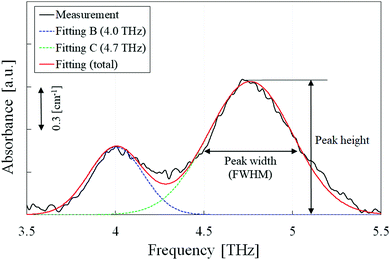 |
| Fig. 2 Peak separation using Gaussian approximation; peaks B and C are separated to obtain the height and width for each spectrum. | |
Fig. 3 shows the relative peak height and width, normalized at 80 °C, as a function of Tc. This provided a clearer and detailed description of the spectral changes in the three peaks (B, C and D). Peak C (4.7 THz) had a similar tendency as that of peak D (7.1 THz), and their relative heights and widths gradually increased and decreased with increasing Tc respectively, indicating that higher Tc samples have higher crystallinity. On the other hand, the relative height of peak B (4.0 THz) increased remarkably as the peak width slightly decreased. This tendency is consistent with XRD profiles, indicating that the sharp phase transition from δ-form to α-form was occurred around 110 °C (Fig. 4). Therefore, the relative peak intensity in THz range is an effective parameter to quantitatively evaluate the ratio of δ-form to α-form in PLLA samples. Peak B is an indicator for the optimization of polymorphism in PLLA, which would eventually influence the piezoelectric and/or biodegradable properties.
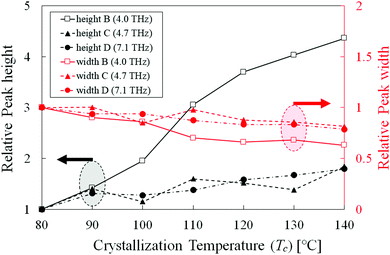 |
| Fig. 3 Relative height and width of peaks B, C, and D plotted against the crystallization temperature, normalized at 80 °C. Absolute height (width) of peak B, C, and D at 80 °C are 4.78 cm−1 (0.36 THz), 9.38 cm−1 (0.56 THz), and 25.13 cm−1 (0.46 THz), respectively. | |
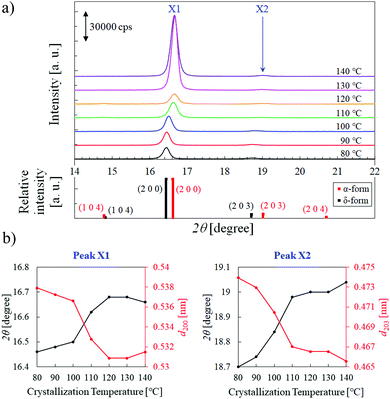 |
| Fig. 4 (a) XRD profiles of PLLA for different crystallization temperature, and standard XRD data of polylactide (JCPDS card no. 64-1623 for δ-form and 64-1624 for α-form). (b) Diffraction angle 2θ of the two peaks labeled in (a). The lattice spacing dhkl values with the Miller indices, calculated by the Bragg equation, are superimposed. | |
To further optimize the above properties of PLLA, it is important to elucidate the origin of peak B. The remarkable increase of the relative height suggests that probable origin is found not in δ-form but in α-form. According to the literature of PLLA crystalline phases reported by K. Wasanasuk and K. Tashiro's group,17 the δ-form is not simply the disordered state of the α-form taking the 103 helix with 21 screw symmetry (the periodic inclusion of 10 monomeric units and the asymmetric inclusion of 5 monomeric units) along the C(CH3)–C(
O)–O chain axis. Such 21 screw symmetry disappears in the δ-form. Therefore, it is natural to consider that the origin of peak B is not due to simply the intermolecular vibration of the PLLA chain but due to a novel intramolecular vibration caused by the 21 screw symmetry inside of the helical chain. In contrast, the higher two peaks (C and D), which are existent not only in α-form but also in δ-form, are thought to be the intermolecular vibration of the PLLA chain depending on the lattice spacing and crystallinity. In our future work, the expansion of the observable THz range and detailed structural analysis will deepen our understanding of the PLLA structures and its functions.
Conclusions
Elucidating the optical properties of PLLA is an important issue for predicting its crystal structure and the degree of disorder nondestructively. We prepared, measured, and analyzed PLLA samples with different crystallization temperatures (Tc = 80–140 °C). We introduced the Fourier transform THz spectroscopy to identify differences in the crystal structure among the samples. For the 80 °C samples, four absorption peaks (A, B, C, and D = 1.8, 4.0, 4.7, and 7.1 THz, respectively) were identified in the frequency range of 1.0–8.5 THz, with the peaks of higher Tc samples undergoing blue-shifts. The peak height and width calculations revealed similar temperature dependence for peaks C and D, whereas the peak B height grew remarkably with increasing Tc and could be used as an indicator for PLLA polymorphism optimization. Broadband THz spectroscopy is a powerful tool for various biodegradable polymer materials.
Author contributions
Seiichiro Ariyoshi: conceptualization, methodology, investigation, formal analysis, project administration, writing – original draft. Satoshi Ohnishi: investigation, data curation, formal analysis, writing – review & editing. Hikaru Mikami: investigation, writing – review & editing. Hideto Tsuji: resources, writing – review & editing. Yuki Arakawa: resources, writing – review & editing. Saburo Tanaka: supervision, writing – review & editing. Nobuya Hiroshiba: conceptualization, methodology, investigation, writing – original draft.
Conflicts of interest
There are no conflicts of interest to declare.
Acknowledgements
This work was partly supported by a Grant-in-Aid for Scientific Research (B) [grant number 17H02809] from the Ministry of Education, Culture, Sports, Science and Technology of Japan. The authors would like to thank Mr Kazunobu Yamada from Research and Development Center, Unitika Ltd, for supplying PLLA used in the present study.
References
- B. Žunar, A. Trontel, M. S. Miklenić, J. L. Prah, A. Štafa, N. Marđetko, M. Novak, B. Šantek and I. K. Svetec, World J. Microbiol. Biotechnol., 2020, 36, 8 Search PubMed.
- S. Hama, S. Mizuno, M. Kihara, T. Tanaka, C. Ogino, H. Noda and A. Kondo, Bioresour. Technol., 2015, 187, 167 Search PubMed.
- K. Okano, S. Hama, M. Kihara, H. Noda, T. Tanaka and A. Kondo, Appl. Microbiol. Biotechnol., 2017, 101, 1869 Search PubMed.
-
P. Emmanuel Vijay Paul and B. Viswanath, Recent Developments in Applied Microbiology and Biochemistry, Academic Press, 2020, ch. 22, 241 Search PubMed.
- Y. Tajitsu, IEEE Trans. Ultrason. Ferroelectr. Freq. Control, 2008, 55, 1000 Search PubMed.
- E. Fukada, Rept. Prog. Polym. Phys. Jpn., 1991, 34, 269 Search PubMed.
- K. Imoto, M. Date, E. Fukada, K. Tahara, Y. Kamaiyama, T. Yamakita and Y. Tajitsu, Jpn. J. Appl. Phys., 2009, 48, 09KE06 Search PubMed.
- N. Tomoshige, H. Mizuno, T. Mori, K. Kim and N. Matubayasi, Sci. Rep., 2019, 9, 19514 Search PubMed.
- L. Wang, K. Okada, Y. Hikima, M. Ohshima, T. Sekiguchi and H. Yano, Polymers, 2019, 11, 249 Search PubMed.
- S. Yamamoto, E. Ohnishi, H. Sato, H. Hoshina, D. Ishikawa and Y. Ozaki, J. Phys. Chem. B, 2019, 123, 5368 Search PubMed.
- S. Ariyoshi, B. Setyawan, S. Hashimoto, S. Negishi, H. Mikami and N. Hiroshiba, RSC Adv., 2020, 10, 8800 Search PubMed.
- H. Hoshina, Y. Morisawa, H. Sato, H. Minamide, I. Noda, Y. Ozaki and C. Otani, Phys. Chem. Chem. Phys., 2011, 13, 9173 Search PubMed.
- H. Li, H.-M. Ye and Y. Yang, Polym. Test., 2017, 57, 52 Search PubMed.
- P. Y. Han, M. Tani, M. Usami, S. Kono, R. Kersting and X.-C. Zhang, J. Appl. Phys., 2001, 89, 2357 Search PubMed.
- P. Song, L. Sang, C. Jin and Z. Wei, Polymer, 2018, 134, 163 Search PubMed.
- J. Zhang, Y. Duan, H. Sato, H. Tsuji, I. Noda, S. Yan and Y. Ozaki, Macromolecules, 2005, 38, 8012 Search PubMed.
- K. Wasanasuk and K. Tashiro, Polymer, 2011, 52, 6097 Search PubMed.
Footnote |
† Electronic supplementary information (ESI) available. See DOI: 10.1039/d1ma00195g |
|
This journal is © The Royal Society of Chemistry 2021 |