DOI:
10.1039/D1MA00193K
(Review Article)
Mater. Adv., 2021,
2, 4532-4573
Recent advances in the synthesis of smart hydrogels
Received
4th March 2021
, Accepted 4th May 2021
First published on 11th May 2021
Abstract
Understanding the surrounding atmosphere and reacting accordingly with a precise action are always fascinating features of a material. Materials that pose such responsiveness are called smart materials. Currently, research studies on smart materials are being accelerated exponentially around the world; this is also true for smart hydrogels. Smart hydrogels with various chemically and structurally responsive moieties exhibit excellent characteristics of reacting under different environmental conditions such as pH, temperature, light, electric field, and magnetic field as well as biological and chemical stimuli. These smart hydrogels are drawing the attention of researchers for a wide range of applications, for instance, in designing biomedical, industrial, agricultural, electrical, healthcare, and hygienic products. This review encompasses the latest developments in the field of smart hydrogel synthesis based on their unique features and different aspects of their responsive behaviors. Additionally, this paper covers some of the recent strategies for tuning special functional properties of smart hydrogels for targeted applications.
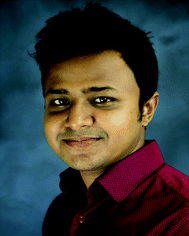 Partha Sikdar | Partha Sikdar is currently a PhD student in the Department of Textiles, Merchandising and Interiors at The University of Georgia (UGA) with his research focusing on Fiber, Polymer and Textile Science. He has been working as a research fellow at the Nonwoven Functional Materials Lab of UGA. He did his master's degree in the same department at UGA. Partha graduated with a Bachelor's degree in Textile Engineering from the University of Chittagong, Bangladesh in 2015. His research focuses on the synthesis of cotton-based nonwovens and composites, stretchable cotton nonwovens, functional biopolymers, and hydrogels. |
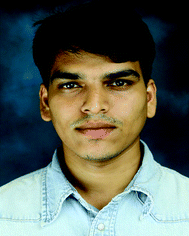 Md. Mazbah Uddin | Md Mazbah Uddin has graduated with a bachelor's degree in Textile Engineering from Bangladesh University of Textiles, Bangladesh. He is currently pursuing his master's degree at the University of Georgia, USA, in polymer, fiber, & textile sciences (PFTS). He is a part of the Innovative Materials Research Team and Nanostructured Materials Lab of the University of Georgia. His current research work involves the fabrication of nanogenerators that are able to harness the mechanical energy generated by the human body. He is interested in biomaterials, nanotechnology, smart textiles, and biomedical implants. |
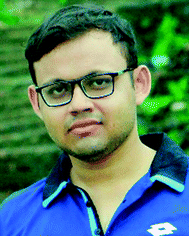 Tanvir Mahady Dip | Tanvir Mahady Dip graduated with a bachelor's degree in Textile Engineering with specialization in Yarn Manufacturing from the Bangladesh University of Textiles. He is working as a lecturer in the Department of Yarn Engineering, Faculty of Textile Engineering, Bangladesh University of Textiles, Bangladesh, with his research focusing on functional polymer coatings, life cycle analysis of textiles, and technical textiles. |
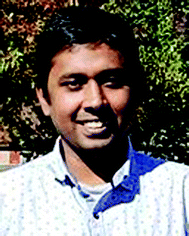 Shafiqul Islam | Shafiqul Islam is a PhD student and researcher at the department of TMI of the University of Georgia. He pursued his undergraduate and master's degrees in textile engineering from the Bangladesh University of Textiles. After completing the undergraduate degree, Shafiqul worked in research and developed sections of an export oriented industry. Later he joined at Dhaka University of Engineering and Technology as a faculty member. Currently, he is on study leave and pursuing his PhD degree at UGA. His main research focus is on the design and development of composite materials and production of environmentally friendly, sustainable insulation materials. He is also working on lifecycle assessment (LCA) to check the true sustainability of produced composite materials and comparing them with those of commercially available products. Another desirable property of such composites is that of self-repairing. At present, Shafiqul is investigating the possibility of incorporating the right ingredients to make self-healing composite materials. |
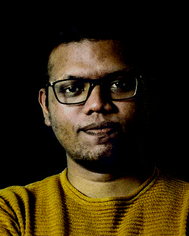 Md. Saiful Hoque | Md. Saiful Hoque, with a solid background in fibers and polymers, textiles, and apparel, is currently pursuing his PhD in Textile and Apparel Science at the University of Alberta, Canada. Mr. Hoque is pursuing his research in protective clothing, which includes the performance of protective clothing, smart textiles for protective clothing, and clothing comfort. |
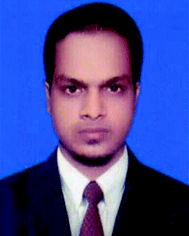 Avik K. Dhar | Avik Kumar Dhar graduated with a master's degree in Textile Engineering from the Bangladesh University of Textiles. In 2016, he joined the Department of Textile Engineering, Shyamoli Textile Engineering College, University of Dhaka as a faculty member, with his research currently focusing on functional fibers and polymers, non-woven, protective clothing, and technical textiles. |
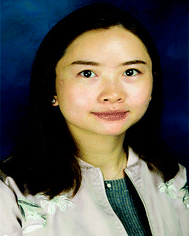 Shuangyan Wu | Shuangyan Wu is a PhD student and a researcher at the department of TMI of the University of Georgia (UGA). She obtained her bachelor's and master's degrees in textile engineering from Jiangnan University, China. Her research focuses on investigating the effect of the incorporation of PUs and nanomaterials such as graphene, fullerenes and carbon nanotubes (CNTs) into fiber reinforced composite structures. |
1. Introduction
Nature has always inspired and intrigued researchers towards the fascinating smart behavior of materials through numerous examples, such as the touch sensitivity of the leaves of Mimosa pudica and Venus flytraps, the photosensitivity of sunflowers and Codariocalyx motorius, and the color sensitivity of chameleons.1 Due to continuous scientific developments and innovations, an insurgence of artificially smart and intelligent materials in the form of smart hydrogels has added a new paradigm in the field of polymer-based biomaterials. The biomimetic nature of hydrogels has envisioned widespread applications in biomedical engineering for cell culture, drug delivery, and therapeutics. Hydrogels are water-absorbing polymeric networks and are developed to mimic many of the inherent properties of soft tissue.2,3 For instance, the properties of hydrogels can be tuned to mimic the biochemical, mechanical, and rheological properties and stimulus responsiveness of soft tissue.4,5 Smart hydrogels can undergo structural and volume phase transitions in response to external stimuli, providing enormous potential for scientific observations and various advanced multidimensional technological applications.6 The development of smart hydrogels as functional materials has revolutionized the field of study concerning responsive materials known as stimuli-responsive hydrogels (SRHs).7 Generally, SRHs are absorbent to superabsorbent8 materials that can react to any subtle environmental changes such as pH,9 temperature,10 chemical species,11 ionic strength,12 electric field,13 and biological conditions.14–16Fig. 1 shows a classification of external factors that can instigate smart hydrogels. These wonderful characteristics have the potential to trigger many applications, for example, biomedical (i.e., tissue engineering, drug delivery, and drug release),17 personal healthcare and hygienic products,18 agriculture (i.e., soil moisturizing, conditioning, nutrient carrier, and erosion control),19 wastewater treatment,20 sensors and actuators,21,22 textiles,23 construction,24 electrical,25 membranes,26 and flocculation.27 Based on their desired application trigger, hydrogels can be synthesized by physical interactions and chemical reactions. Physical hydrogels can be developed either by the interaction between oppositely charged polyelectrolytes or oppositely charged multivalent ion/surfactants and polyelectrolytes.28,29 In contrast, chemical hydrogels are typically formed through a covalently crosslinked polymeric network.30 These smart hydrogels are capable of swelling and shrinkage reversibly in response to changes in external environmental stimuli.7 These hydrogels are composed of homo-polymeric, co-polymeric, or multi-polymeric networks that are synthesized from one, two, or multiple polymers, respectively.29,31 Consequently, these hydrogels can display many functionalities. Such functional hydrogels can be engineered in multiple desired dimensions. For example, they can be subjected to biomedical applications by modifying their chemical structure, composition, biological functions, biodegradability, and various physicochemical properties such as mechanical and rheological, spectral, pH stability, release, and loading properties.32–34
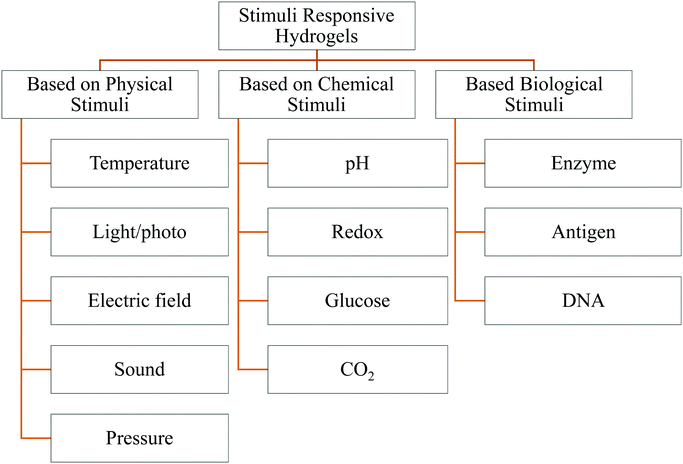 |
| Fig. 1 Major classification of SRHs (based on ref. 48 and 58–61) [reproduced with permission from ref. 58, ©The Royal Society of Chemistry, 2014]. | |
SRHs can be engineered based upon the application of external stimuli that trigger them to show a switchable sol–gel transition. External stimuli, including temperature, light, magnetic and electrical fields, and ultrasonic waves, are considered as physical triggers, while pH and redox reactions are considered as chemical triggers.35 In addition, biological triggers include enzymes,36–40 antigens,14,41 and DNA.42–45
During the past few decades, a plethora of research studies have been conducted to fabricate hydrogels with stimuli-responsive properties that correspond to regular hydrogel attributes such as swelling, porosity, physical structure, and modulus.46–48 Furthermore, the diversified properties and applications of hydrogels have resulted in thousands of research articles focusing on various aspects. In addition, numerous review articles have been found based on hydrogel applications49–52 and their types.53–57 However, a critical review addressing the techniques to tailor different functional properties and governing mechanisms of responsive behavior is yet to be amassed into a single article. This review article is therefore devoted to designing this. In this review article, we explicitly concentrated on the recent and advanced hydrogel synthesis technologies, together with process design implications, and optimized conditions of the preparation process.
2. Typical polymerization techniques for hydrogel synthesis
Hydrogels are three-dimensional polymeric networks that can absorb and contain water due to the presence of hydrophilic groups in the constituent polymeric networks. The term “network” implies the presence of crosslinks within hydrogels which provide pure elastic to viscoelastic properties. Hydrophilic monomers are typically used to prepare hydrogels, but hydrophobic monomers are also included to tune the physical, mechanical, and chemical properties towards specific applications. Both synthetic and natural polymeric networks can be regarded as hydrogels. Typically, synthetic polymers are hydrophobic and more chemically stronger than natural polymers. They provide a slow degradation rate and a mechanically robust structure when incorporated with natural polymers.62–64
The most practical approach to producing SRHs is to combine monomers that exhibit different behaviors in response to various environmental stimuli. Any technique that is appropriate for synthesizing a cross-linked polymeric network can be applied to produce hydrogels. Free radical polymerization techniques are commonly applied to natural and/or synthetic hydrophilic monomers with multifunctional cross-linkers to produce hydrogels.31 The choice of the polymerization process/technique influences the properties of the resultant hydrogels greatly.65 Hydrogels can be synthesized either through a one-step process in the form of simultaneous polymerization and crosslinking of multifunctional monomers or by a stepwise process of producing polymers with reactive groups that can crosslink themselves or can react with appropriate crosslinkers.31,66,67
Composite hydrogels are developed by combining two or more types of organic, inorganic, and polymeric materials in combination to bring about the best synergistic effect of those materials for specific applications. For example, achieving desired mechanical strength and conforming to a particular shape could be achieved by incorporating hydrophobic polymer segments in the presence of crosslinkers.67,68
2.1. Chain growth polymerization
Chain growth polymerization with a free radical mechanism is popular to synthesize chemically crosslinked hydrogels. The polymerization process involves three steps: initiation, propagation, and termination. Mostly hydrophilic –C
C– containing monomers participate in free radical polymerization.69 Various free radical polymerization techniques for hydrogel synthesis are described below.
Bulk polymerization (Fig. 2a) is widely applied to produce hydrogels because of its simple mechanism. This technique involves the polymerization of liquid monomers and monomer-soluble initiators with a small number of crosslinkers. The polymerization process is typically initiated using ultraviolet light, radiation, and/or chemical catalysts.72 Shin et al. synthesized pH-responsive bulk polymerized hydrogels from sodium functionalized acrylic acid (NaAAc) and hydroxyethyl methacrylate (HEMA). At first, AAc was neutralized by NaOH to get NaAAc. Afterward, the initiator (i.e. α,α′-azobisisobutyronitrile: AIBN) and crosslinker (N,N′-methylenebisacrylamide: MBAAm) along with HEMA were added to the reaction mixture. The polymerization was continued on a Petri dish for 30 minutes at 75 °C in an oven. The unreacted reaction ingredients were discarded by repeatedly washing with deionized (DI) water.73 Bulk polymerization has a higher polymerization rate and inefficient heat control, and the viscosity of the reaction increases very rapidly. Therefore, it is crucial to control the conversion rate to tune the hydrogel properties. The resultant hydrogels exhibit a glassy and transparent polymer matrix, which swells and becomes flexible upon immersion into water.31 With the increase in reaction temperature and the initial concentration of the initiator, the rate of polymerization and conversion increases. By controlling temperature and the concentration of the initiator, it is possible to control the conversion rate of bulk polymerization.74 Besides, terminating the reaction at low conversion could be another approach, but in large-scale processes it is considered as uneconomical. Hence, other polymerization techniques such as solution, suspension, and emulsion polymerization are widely adopted for hydrogel synthesis.75
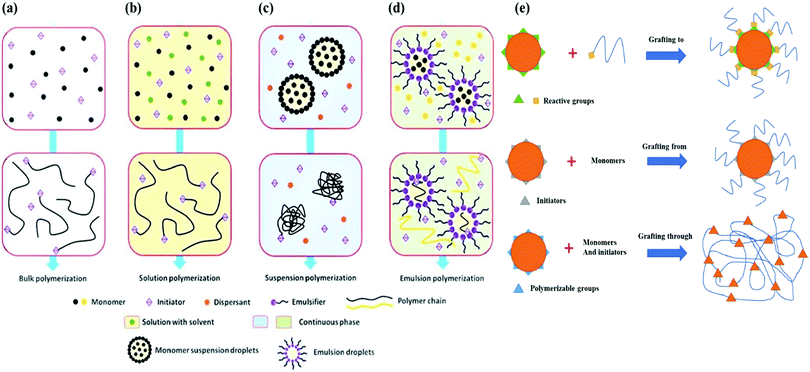 |
| Fig. 2 Schematic diagram of the common free-radical polymerization techniques. (a) Bulk polymerization. (b) Solution polymerization. (c) Suspension polymerization. (d) Emulsion polymerization [reprinted with permission from ref. 70, ©2021 by the authors, open access under attribution 4.0 international (CC BY 4.0)]. (e) Graft polymerization techniques [reprinted with permission from ref. 71, ©2018 by the authors, open access under attribution 4.0 international (CC BY 4.0)]. | |
2.1.1. Solution polymerization. In solution polymerization, ionic or neutral monomers with a solvent (e.g., benzyl alcohol, water, ethanol, or water–ethanol mixtures) and multifunctional cross-linkers undergo polymerization by ultraviolet (UV) or redox initiation to form hydrogels. The hydrogel is separated, and the residual monomers, cross-linkers, initiators, and other impurities are extracted by washing with distilled water (Fig. 2b).76 Solution polymerization is well-known for ease of synthesis and low-cost processing with better heat transfer control during polymerization. Since polymerization occurs in the aqueous medium, it is safe and harmless.72,77 Solution polymerization is greatly used for synthesizing cellulose-based superabsorbent hydrogels. The polymerization rate is high, and the reaction can be conducted at room temperature. Since the solution has low viscosity, stirring of the reaction mixture becomes easier; therefore, better heat transfer and dissipation are achieved in solution polymerization than in bulk polymerization.77,78 Generally, bulk and solution polymerization processes are homogenous with the potential to become heterogeneous when the formed polymer is insoluble in the monomer and solvent in the respective processes.78
2.1.2. Suspension polymerization (including inverse-suspension polymerization). Suspension polymerization (Fig. 2c) involves generally insoluble monomers and initiators with a low hydrophilic–lipophilic balance suspending agent in aqueous solution. They are constantly agitated to create droplets of monomers (0.1–5 mm in diameter). As polymerization proceeds, the polymer hydrogel beads are formed, which can be filtered to separate from the reaction mixture.57 Herein, individual monomers undergo small-scale bulk polymerization. Since water is the usual medium, it functions as an excellent heat transfer medium. However, a protective colloidal agent [e.g., carboxymethyl cellulose (CMC) or methylcellulose (MC) and polyvinyl alcohol (PVA)] is often used to obstruct the coalescence of the droplets.78 Inverse suspension polymerization is also widely used for hydrogel synthesis.79
2.1.3. Emulsion polymerization (including micellar polymerization). Synthesis of hydrogels can also be done by emulsion polymerization. A typical emulsion polymerization process involves a water-soluble initiator, a surfactant, crosslinkers, and real but small water-soluble monomers (i.e. slightly water-soluble or completely hydrophobic monomers).80,81 In inverse emulsion polymerization, a hydrophilic monomer from an organic liquid is used.76Fig. 2d shows a typical emulsion polymerization technique. It produces polymer particles much smaller than those from suspension polymerization (0.1–3 μm). The suspension and emulsion polymerization processes can be easily controlled and heat transfer can be performed effectively over bulk polymerization.78 In contrast to suspension polymerization, emulsion polymerization uses a water insoluble initiator.
2.1.4. Graft polymerization mechanism. Poor mechanical properties of a bulk polymerized hydrogel can be induced when using a grafting technique, especially when grafted onto more robust support frames. Usually, free radical sites are generated on the surface of the support where monomers can be directly polymerized to form stronger covalent bonds with the support structure. For example, grafting vinyl monomers onto polysaccharides is common.31 Besides, grafted PAAc from hybridized chitosan (CHT) with cellulose via thiourea formaldehyde resin produces pH-responsive hydrogels which are mechanically more robust than grafted AAc from CHT hydrogels.82Fig. 2e demonstrates a different grafting approach to synthesize hydrogels.
2.2. Step-growth polymerization
Step-growth polymerization utilizes distinct functional groups containing monomers to synthesize hydrogels. These complementary functional groups react and form covalent bonds to propagate a one-step polymerization process.69 Tibbitt et al. studied the mechanical properties of photodegradable hydrogels synthesized via chain and step-growth polymerization processes (Fig. 3). It was demonstrated that mechanical integrity, tensile toughness, ductility, and shear strain to yield of step-growth hydrogels were better than those of chain growth hydrogels because of network homogeneity and cooperativity. However, the rate of erosion due to light exposure was less for chain-growth hydrogels due to higher network connectivity in chain-grown hydrogels.83 With the understanding of hydrogel networking, desirable properties of hydrogels can be achieved.
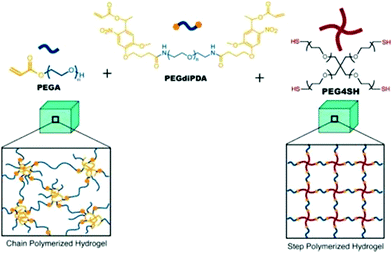 |
| Fig. 3 Photo-degradable hydrogel based-on chain and step polymerization of a photolabile monomer (PEGdiDPA). (a) Chain polymerization of PEGdiPDA and PEGA via free radical mechanism (e.g. heterogeneous network structure). (b) Step-polymerization of PEGdiDPA with PEG4SH via Michael-addition polymerization [reprinted with permission from ref. 83, ©American Chemical Society, 2013]. *PEGA (monoarylated poly(ethylene glycol)); poly(ethylene glycol) di-photodegradable acrylate (PEGdiPDA); four-arm poly(ethylene glycol) functionalized with thiol end groups (PEG4SH). | |
3. Crosslinking methods of hydrogel fabrication
Since hydrogels contain hydrophilic segments, cross-linking is essential to avoid the dissolution of hydrophilic polymer segments in the aqueous medium. Without substantial cross-links, the solutions of hydrophilic polymers behave as Newtonian fluids. On the other hand, elastic to visco-elastic behavior is achieved by increasing the number of cross-links.66 The degree of crosslinking is correlated with overall hydrogel properties and characteristics.59,60
Typically, hydrogels are classified into two categories and their 3D networks can be formed in numerous ways, as shown in Fig. 5.84 The first category is known as physically crosslinked hydrogels that form networks in polymers via physical interactions, such as ionic interactions between polycations/multivalent cations and polyanions, or hydrophobic interactions in polymer chains, and so on (Fig. 4a). The other category is called chemically crosslinked hydrogels that form networks in polymers via chemical interactions (i.e. covalent bonds). Herein, crosslinking can be instigated by heating, or ultraviolet radiation, and/or by chemical crosslinking achieved by different reactions, such as Michaelis–Arbuzov reaction, nucleophilic reaction, and Michael's reaction, to name a few (Fig. 4b).85,86 Theoretically, different applications of hydrogels are possible through the optimization of their properties which is controlled by the degree of crosslinking.87,88
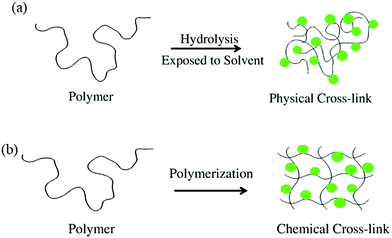 |
| Fig. 4 Schematic illustration of hydrogel fabrication. (a) Physical cross-linking. (b) Chemical Crosslinking [reprinted with permission from ref. 77, ©Springer Nature, 2018]. | |
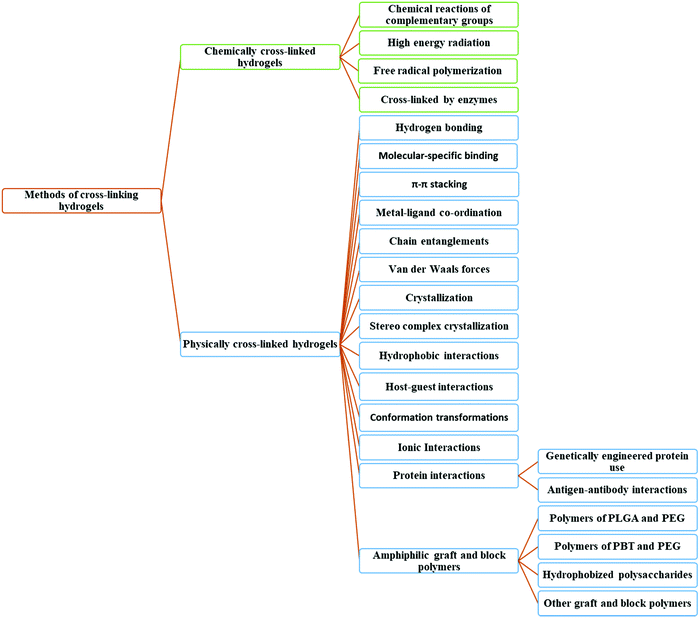 |
| Fig. 5 Methods of cross-linking hydrogels (based on ref. 63). *Polylactic-co-glycolic acid (PLGA); polybutylene terephthalate (PBT). | |
4. Synthesis of stimulus-responsive hydrogels (SRHs)
4.1. Synthesis of thermo-responsive hydrogels (TRHs)
Hydrogels responsive to temperature are considered as one of the smartest types of hydrogels that can change their shapes and formation in response to temperature changes.89 They are mainly characterized by the presence of hydrophobic groups, such as methyl, ethyl, and propyl groups.90 Usually, TRHs can be divided into positively thermo-sensitive, negatively thermo-sensitive, and thermally reversible gels.91 Positively thermo-sensitive hydrogels swell at high temperatures and shrink at low temperatures, for instance, interpenetrating polymeric networks (IPNs) based on poly(acrylamide-co-butyl methacrylate) [P(NIPAAm-co-BMA)] and PAAc.92 Conversely, negatively thermo-sensitive hydrogels swell with decreasing temperature and vice versa. TRHs can be synthesized from both natural polymers (e.g., chitosan, cellulose, and gelatin) and synthetic polymers (e.g. poly(N-isopropylacrylamide) (PNIPAAm) and polyfluorene 127).93 Here, the choice of a monomer is important because the behavior of a hydrogel is largely regulated by the type of monomer and crosslinker used for the synthesis. The most common monomer, NIPAAm, is widely used to design drug release systems. Research reveals that the presence of both hydrophilic amide groups and hydrophobic isopropyl groups in side chains allows PNIPAAm to show temperature-controlled phase transition behavior in aqueous solution at a lower critical solution temperature (LCST) of around 32 °C.94–96 PNIPAAm is one of the most intensely studied hydrogels, especially in biomedical applications, because its volume phase transition temperature (VPTT) at LCST is close to human body temperature and due to its fast on-off switching.89,97 Some typical parameters and ingredients considered in the synthesis of PNIPAAm are shown in Table 1.
Table 1 Conditions and parameters of PNIPAAm synthesis
Synthesis method |
Synthesis temperature |
Fluid |
Crosslinkers |
Reagents |
Effect on PNIPAAm properties |
Ref. |
Abbreviations: ammonium persulfate (APS); N,N,N′,N′-tetramethyl ethylenediamine (TEMED); potassium persulfate (KPS); sodium dodecyl sulfate (SDS); and N,N′-methylenebisacrylamide (MBAAm). |
Free radical polymerization |
22 °C |
DI water |
MBAAm |
APS and TEMED |
Increased sensitivity to temperature triggered controllable drug release and improved mechanical properties |
98 |
Surfactant-free emulsion polymerization |
70 °C |
DI water |
MBAAm |
KPS |
Improved crosslinks, charge distribution impact and temperature-responsive deswelling behavior |
99 |
Free radical polymerization |
10, 15, 20, or 25 ± 0.2 °C |
DI water |
MBAAm |
APS and TEMED |
The transition towards a turbid substrate became more gradual (at 33.5–34.5 °C) which consequently had an influence on the light scattering behavior in response to temperature |
100 |
Free-radical precipitation copolymerization |
70 °C |
DI water |
MBAAm |
SDS, APS |
The particle size can be influenced by controlling the concentration of the free radical initiator, which is a crucial factor for drug delivery |
101 |
Sedimentation polymerization |
80 °C |
Silicone oil |
MBAAm |
KPS, TEMED |
The swelling rate of the resulting hydrogel beads was directly influenced by the morphology (especially porosity in the structure) of the gel delivered by the applied polymerization |
102 |
Another source of novel thermo-responsive hydrogels can be in the form of renewable resource-based hydrogels. It is possible to synthesize them using bacterial cellulose (BC) and castor oil, in combination with NIPAAm.103 Most of the thermo-responsive polymers undergo phase transitions with the change of temperature.104 The critical solution temperature is responsible for controlling the solubility of such hydrogels.47,105 The synthesis of thermo-responsive hydrogels from polymers using temperature changes as a trigger is shown in Fig. 6.
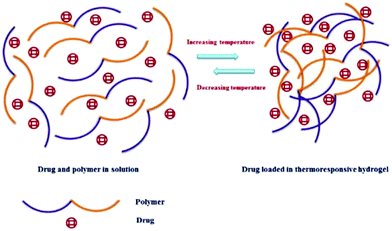 |
| Fig. 6 Formation of TRHs using temperature as a trigger [reprinted with permission from ref. 93, ©2018 by the authors, open access under attribution 4.0 international (CC BY 4.0)]. | |
Some cellulose-based functionalized MCs with the protein laminin can be used to develop a bioactive scaffold for neural tissue engineering.106 Similarly, composite thermo-sensitive hydrogels synthesized from CHT/CMC, CHT/collagen, and CHT/PEG can be alternatives to invasive surgeries.107–109 They can also be used as potential targeted drug carriers that are injected into the body as a liquid and form a gel if the body temperature rises above their LCST.84
TRHs have become an optimum material for advanced bioprinting applications because of their easy tunability from the sol–gel state by changing temperature (rapid gelation), printability with good shape fidelity, high resolution,110 cell compatibility111,112 with the ability to mimic the micro-environment, natural shape, and vascularization.113,114 TRHs that are used for bioprinting can be synthesized from gelatin and its derivatives, MC, agarose, collagen, pluronic and its derivatives, PEG/polyethylene oxide (PEO) based block polymers, and poly(N-PNIPAAm) and its derivatives.115 Josergio et al. developed a composite PAAm hydrogel containing silica nanoparticles (NPs) with an enhancement in the thermal diffusivity of PAAm hydrogel nanocomposites.116 Chu et al. summarized the most used techniques to fabricate and engineer different thermo-responsive smart hydrogel materials in different forms of functional compounds (shown in Fig. 7).117
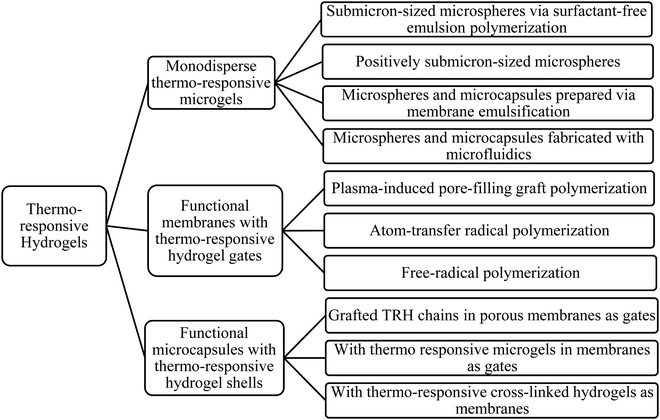 |
| Fig. 7 Synthesis and engineering techniques for different thermo-responsive smart hydrogels (based on ref. 117). | |
4.2. Synthesis of light-responsive hydrogels (LRHs)
Hydrogels responsive to light show reversible deformations from a flowable state to a non-flowable state when exposed to light sources such as UV light, visible light, and near IR light.54,118 LRHs are generally classified into two categories: one contains photolabile moieties (e.g. azobenzene and o-nitro benzyl) and the other contains near IR absorbing nanostructures such as nanorods, nano-shells, and carbon nanotubes embedded in thermo-responsive hydrogels.119 Such hydrogels are functionalized as light-sensitive through photochromism by incorporating photochromic molecules into the hydrogel matrix. This can be performed by chemical coupling or mechanical processes and controlled by the right selection of chromophores, wavelength, light intensity, and chromophore–polymer interactions.120 These hydrogels can undergo sol–gel phase transitions due to the cleavage of photo-responsive moieties linked to the hydrogel networks, contraction–expansion of volume occurring due to photothermal heating of NPs incorporated within TRHs,121 and/or chemical modifications (as demonstrated in Fig. 8).122
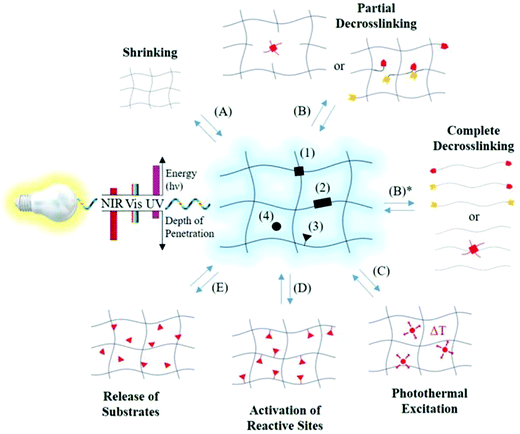 |
| Fig. 8 Molecular architecture and responses of a photo-responsive hydrogel (center). The photo-responsive entities (black geometries) may be present at (1) crosslinking points, (2) polymer or supramolecular backbone, (3) side chains, or (4) aqueous medium of dissolved hydrogels. Based on the placement and photo-responsive moieties, photoresponses may include (A) shrinkage and (B) partial de-crosslinking (results in water uptake and thus increasing hydrogel volume). Nevertheless, increasing photoinduced crosslinking leads to hydrogel shrinkage and full de-crosslinking leads to degradation to liquefication of hydrogels (B)*. Besides these, there can be (C) photothermal excitation due to the increase in temperature locally, (D) reactive site activation or deactivation, and (E) release or capture of substrates [reprinted with permission from ref. 122, ©John Wiley and Sons, 2019]. | |
Ter Schiphorst et al. developed a light-responsive hydrogel by incorporating chromophore (photochrome) spiropyrans via copolymerization with NIPAAm and AAc. This hydrogel contracts and swells due to the photoisomerization of the photochrome.123 In another study, the incorporation of metallic NPs such as Au, Pt, Ag, and Cu was reported to synthesize light-sensitive hydrogels due to their superior light adsorbing and/or scattering capability than molecular chromophores.124 The light responsiveness of hydrogels based on trans–cis photoisomerization and thereby sol–gel and gel–sol transitions were reported by Zhao and Fraser Stoddart. The photoinduced cis–trans isomerization of azobenzene found applications in many hydrogel systems. The host–guest119 chemistry of some materials such as cyclodextrins (CDs) and their derivatives was also used in the synthesis of light-responsive hydrogels.125 Another study suggests that the composite hydrogels of PNIPAAm synthesized with the nanofillers of glycidyl methacrylate (GMA) functionalized graphene oxide (GO) exhibited light responsiveness due to the infrared light sensitivity of GO.126 A light-responsive hydrogel of polymeric NPs (light-sensitive) embedded in a thermo-responsive and mechanically stable double networked hydrogel was recently developed.121 Hydrogels based on photo-responsive agents (e.g., polydopamine)127 and semi-conductive polymers128 were also synthesized and studied to fabricate light-responsive hydrogels.
4.3. Synthesis of electrically conductive hydrogels (ECHs)
Hydrogels responsive to electrical stimuli are typically polymeric blends or co-networks of electroactive polymers and highly hydrated hydrogels. The electroactive constituent contributes to electrical conductivity and on–off electrical and optical switching, whereas the hydrated constituent endows the swelling ability, biocompatibility, and small molecular diffusion.129 When a water-swollen crosslinked polyelectrolyte gel (i.e. electroconductive hydrogels) is sandwiched between two electrodes and subject to a DC voltage, a potential gradient is generated, and based on the charges on the hydrogel it contracts anisotropically, thereby discharging the water content.13,48,130 Hydrogels containing ionic moieties exhibit such a contraction mechanism, whereas hydrogels with charge neutrality show zero contraction. The presence of polyanions makes hydrogels contract extensively near the anode and swell to a lesser extent near the cathode. On the contrary, hydrogels with polycations show an opposite contraction–swelling mechanism, meaning that they contract extensively near the cathode and show little swelling near the anode. Moreover, the contraction rate increases with the applied electric field.130–132
The use of free radical polymerization and the chemical crosslinking approach was reported for the fabrication of electric field responsive hydrogels, for example, poly(2-(acrylamido)-2-methylpropanesulfonic acid) (PAMPS) hydrogels.130,133
ECHs are synthesized by the incorporation of conductive materials (Fig. 9) such as metallic NPs (e.g. Au NPs), conductive polymers (e.g., polypyrrole (PPy), polyaniline (PANI), and poly-3,4-ethylene dioxythiophene: PEDOT, etc.), and carbon-based materials (e.g., graphene and carbon nanotubes) as fillers into the hydrophilic hydrogel matrix.13,134
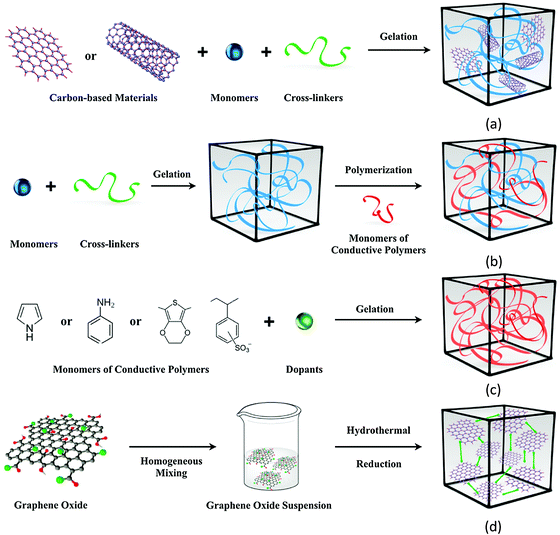 |
| Fig. 9 Four main approaches were used to obtain ECAs. (a) Hydrogel formation from a conductive filler suspension. (b) Polymerization within a preformed hydrogel matrix. (c) Crosslinking conductive polymers by dopant molecules. (d) Self-assembly of a graphene hydrogel via supramolecular interactions [reprinted with permission from ref. 134, ©Elsevier, 2019]. | |
The application of ECHs in soft electronics is drawing attention due to their enhanced conductivity, stretchability, and flexibility.135,136 These properties are favorable for flexible strain sensors and highly applicable in soft robotics, human–machine interfaces, and micromachines.131 Biosensors and bioelectronics-based conductive hydrogels for the detection of pathogens,137 glucose concentration,138 and viruses,139 delivering drugs,140 and environmental monitoring140 are being developed rapidly.141
4.4. Synthesis of magnetically responsive hydrogels (MRHs)
Usually, magnetic responsive behavior can be imparted by incorporating magnetic NPs (MNPs) as a dispersion in a cross-linked polymeric matrix rendering responsive behavior against magnetic stimuli.142 With the change of magnetic state, the mechanical, thermal, and acoustic behaviors are also affected simultaneously as the magnetic part behaves as a composite along with the hydrogel matrix.143 Due to the inclusion of magnetic NPs, the hydrogel application expands through many areas. Moreover, the optimum effect can be achieved by ensuring the homogeneous distribution of magnetic NPs to create a hybridized hydrogel composite.144,145 Therefore, in the case of magnetic NPs embedded into hydrogels, it is necessary to control the distribution of NPs within the hydrogel network which can be manipulated by creating a magnetic field outside. Furthermore, the properties of such a hydrogel can also be affected by factors like the concentration and size of both the hydrogel and magnetic particles. These magnetic gel types can be synthesized by incorporating NPs that are responsive to a magnetic field into a cross-linked hydrogel network. Mainly, three major synthesis processes are followed to develop a magnetic nanoparticle hybridized hydrogel: the blending method, the encapsulation or co-precipitation method, and the grafting approach (Fig. 10).143,146–149
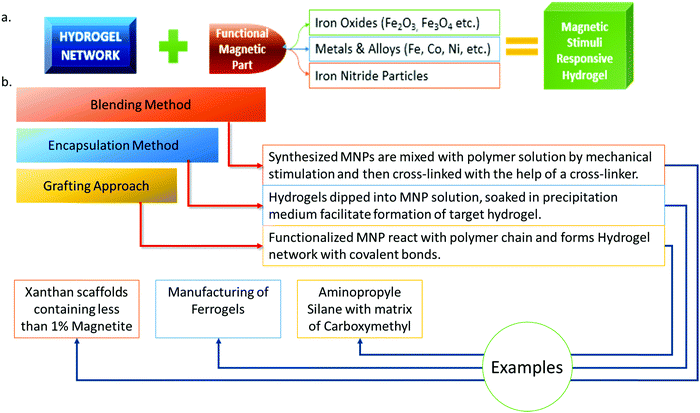 |
| Fig. 10 (a) Principle of magnetically responsive hydrogel synthesis (based on ref. 143). (b) Major synthesis principles of MNP hydrogels with examples (based on ref. 142, 143 and 150–157). | |
By comparing hydrogels with randomly distributed MNPs with those incorporating homogeneously distributed MNPs, it is found that the distribution arrangement greatly influences the magnetothermal behavior of hydrogels. Hence, magnetothermal properties can be regulated by controlling the arrangement of MNPs inside the hydrogel via the regulation of the magnetic field.151–154 To improve the collective magnetic properties of MNPs as well as their monodispersity and homogeneity, they can be assembled into a one-dimensional structure (Fig. 11).144,158
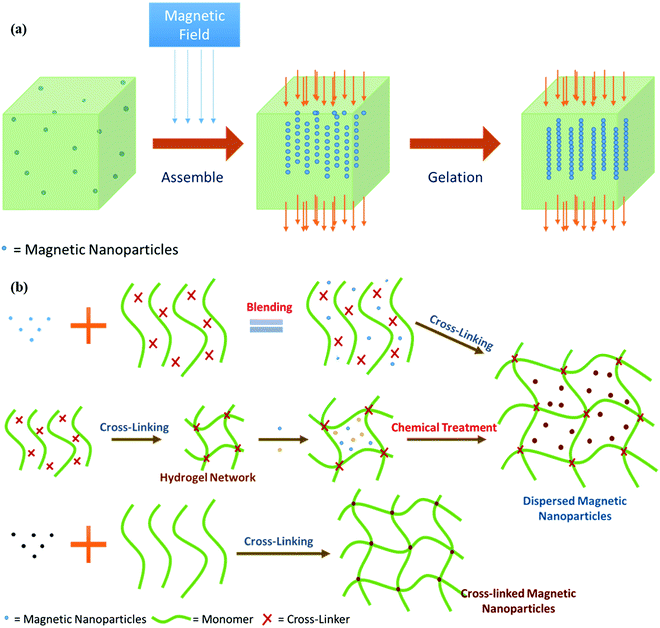 |
| Fig. 11 (a) Effect of magnetic field on MNPs incorporated in the hydrogel [reproduced from ref. 3]. (b) Major preparation methods for synthesizing a magnetic nanoparticle-induced hydrogel and a regenerated nanoparticle-induced hydrogel [reproduced with permission from ref. 146, ©2015 by the authors, open access under attribution 4.0 international (CC BY 4.0)]. | |
Besides, when MNPs are exposed to an alternating electromagnetic field, the magnetization flip dissipates thermal energy to the surroundings and causes magnetic hyperthermia.155,156,162 This phenomenon has been largely used in chemotherapy to kill malignant cells.159,160,163,164 There are four major methods for the synthesis of magnetic stimulus hydrogels with suspension polymerization (Fig. 12).
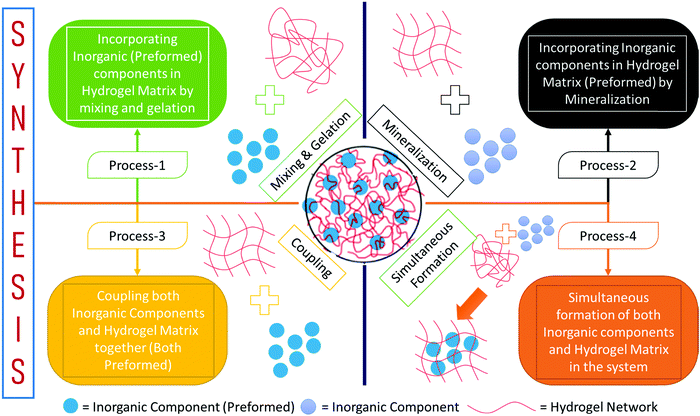 |
| Fig. 12 Major synthesis processes of magnetic stimulus hydrogels with suspension polymerization (based on ref. 147 and 161–165). | |
4.5. Synthesis of sound responsive hydrogels (SORHs)
Among different environmental stimuli, smart hydrogels are also responsive to sound or ultrasound.166,167 Ultrasound refers to the sound beyond the limit of human hearing and has applications in many fields such as cleaning, mixing, and imaging.168,169 Particularly in the biomedical field (e.g., drug release, cancer therapy), its applications with hydrogels are prevalent.167,170–175 Polymers or hydrogels can be activated and allowed to burst due to their interaction with ultrasound waves, thereby releasing drug loads into specific tissues (Fig. 13a).
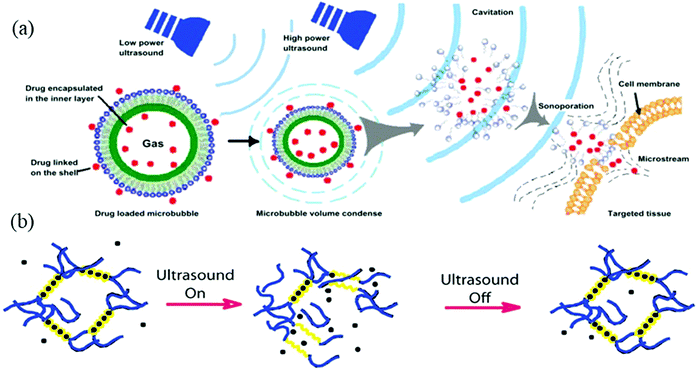 |
| Fig. 13 (a) Schematic diagram of the working principle of low and high ultrasound on polymers for drug release [reprinted with permission from ref. 176, ©Dovepress, 2013]. (b) Disruption and self-healing of an ultrasound responsive ionic cross-linked hydrogel [reprinted with permission from ref. 170, ©PNAS, 2014]. | |
SORHs exhibit a reversible de-crosslinking–crosslinking behavior when interacting with sound waves. In the presence of ultrasound, crosslinks break and, conversely, regenerate upon the withdrawal, thus rendering the self-healing ability to SORHs (Fig. 13b). This mechanism can be incorporated to release drugs in a controlled manner.170
The synthesis of SORHs varies with their application. For example, Zhang et al.177 developed a hydrogel composite with an MXene (Ti3C2Tx), which was found to be successful in monitoring the phonatory process or sound of the human voice. MXene (Ti3C2Tx) nanosheets with PVA, water, and anti-dehydration agents were combined to fabricate this hydrogel.
Kwok et al.178 synthesized a SORH to facilitate on-demand drug release. They developed a methylene chain within the drug insulin polymer to produce the final ultrasound responsive hydrogel. Crosslinked hydrogel slabs were made via copolymerization of the monomer HEMA, 2-hydroxyethyl acrylate (HEA), poly(ethylene glycol) dimethacrylate (PEGDMA), and insulin. PEG was incorporated to form large pores so that the hydrogel can retain large protein molecules like insulin. Then the methylene chains were developed over the PHEMA surface by processing it with C-12 isocyanate, dibutyltin dilaurate (catalyst), and anhydrous tetrahydrofuran.178,179 Exposure of ultrasound to this insulin drug-containing hydrogel showed successful sound controlled drug release.178
4.6. Synthesis of pressure responsive hydrogels (PRHs)
The ability of hydrogels to detect the pressure exerted on them and exhibit various responses under various pressures is a novel characteristic. This behavior is apparent in super-elastic, cellular structured, and nanofibrous hydrogels, for example, hydrogels produced by the combination of alginate and flexible SiO2 nanofibers. Hydrogels with an ultrahigh water content (above 97%) do not exhibit robust mechanical strength and significant recoverable deformation because of containing a high amount of water. These hydrogels are formed by assembling different polymer units, such as proteins, NPs, polysaccharides, polyelectrolytes, and so on, into 3D networks. Although they have a homogeneous distribution of networks, they tend to lose mechanical properties in a high water-containing environment. In such a case, hydrogels of cellular fibrous networks can readily improve mechanical properties and exhibit a significant response when pressure is applied.180–184
Respective swelling/shrinkage and expansion/contraction are apparent upon the application of external pressure on hydrogels containing large mono-domains. A study reports the incorporation of a mechanical shearing technique for synthesizing half ester nanocrystallized cellulose (CNC). This includes UV-triggered polymerization of a monodomain anisotropic gel converted by shearing. When subjected to pressure between cross-polarizers, this hydrogel displays rapid and reversible color changes, accompanied by the degree of alignment observed as swelling.185,186
In another study, a SiO2 nanofibrous hydrogel was synthesized by incorporating four elements, namely, water, alginate, SiO2 nanofibers, and a metallic cation (Al3+), by applying the sol–gel electrospinning technique.187 The pressure-responsive characteristics can be readily used for different advancements in the field of hydrogels such as flexible pressure sensors, tissue-engineered scaffolds within the range of pressure stimulus, drug delivery vehicles, artificial skin, and bio-actuators, to name a few.180
4.7. Synthesis of pH-responsive hydrogels (pHRHs)
pH-Responsive hydrogels are generally synthesized by utilizing polymers constructed using monomers containing ionizable pendant/functional groups of –OH, –COOH, –NH2, –CONH2, –N<, and –SO3H.12,48,188,189 When such a hydrogel is exposed to a solvent (e.g., aqueous solution) of certain pH and ionic strength, its pendant/functional groups will get ionized with fixed charges and these charges will exhibit electrostatic repulsion, resulting in a reversible swelling–deswelling mechanism depending on the acidic or basic nature of the medium.48 The presence of anionic and cationic pendant groups in polymers influences pH depending on the swelling behavior of the hydrogels. Hydrogels with cationic pendant groups [e.g. CHT, poly(N,N-dimethylaminoethyl methacrylate) (PDMAEMA), and poly(N,N-diethylaminoethyl methacrylate) (PDEAEMA)] swell at pH < pKa and deswell at pH > pKa (Fig. 14a), whereas hydrogels with anionic pendant groups (e.g. albumin and PAAc) swell at pH > pKa and deswell at pH < pKa (Fig. 14b).48,190,191 Both synthetic and natural polymers with ionizable functional groups can be utilized to synthesize hydrogels with pH-responsive behaviors.48 This pH-stimulated reversible shrinkage-expansion mechanism of hydrogels is utilized to deliver therapeutic drugs protectively.192
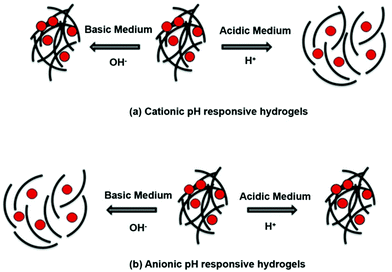 |
| Fig. 14 The schematic representation of pH-responsive hydrogels. (a) Cationic hydrogels swell at low pH and deswell at high pH. (b) Anionic hydrogels swell at high pH and deswell at low pH [reprinted with permission from ref. 190, ©2019 by the authors, open access under attribution 4.0 international (CC BY 4.0)]. | |
Numerous approaches can be adopted to synthesize pH-responsive hydrogels such as free radical polymerized chemical crosslinking,188 free radical polymerized physical crosslinking,193 grafted polymerization,194 injection emulsion polymerization,9 radiation polymerization and crosslinking techniques,195 template polymerization,196 covalent linkages,197,198 click reactions,199etc. The major advantage of these hydrogels is their capability to administer drugs (especially protein and peptide-based drugs) orally while protecting them through the gastrointestinal tract (Fig. 15).193
Hibbins et al. developed a novel drug delivering pH-responsive hydro-porous hydrogel based on in situ free-radical copolymerization of the monomers AAm and methacrylic acid with MBAAm as the chemical crosslinking agent.188 Similar polymerization and crosslinking approaches were utilized by Shantha and Harding to fabricate pH-responsive hydrogels of N-vinylpyrrolidone (NVP), PEG diacrylate, and chitosan, where the –NH2 groups of chitosan were able to ionize in acidic medium (pH 1.2). A combined process of graft polymerization and free radical polymerized chemical crosslinking was applied to synthesize dual responsive hydrogels (i.e. pH and temperature) by grafting cellulose nanowhiskers onto AAm (CNWs/AAm); afterward, CNWs/AAm was polymerized with NIPAAm in the presence of MBAAm crosslinkers to obtain the final hydrogels.194 Hydrogels based on the physical interactions of AAc and 2-(dimethylamino) ethyl methacrylate (DMAEMA) copolymers developed via free radical polymerization were also studied by Suhag et al.193
Hydrogels obtained through γ-irradiation initiated copolymerization and the crosslinking approach were reported to be more advantageous than those obtained via chemically initiated polymerization and crosslinking due to the exclusion of additives (i.e. initiators and crosslinkers). This approach produces sterilized hydrogels of non-carcinogenesis. Hydrogels of AAc and DMAEMA obtained via the radiation technique were reported and simulations were carried out in a buffer solution to study ketoprofen drug delivery in the colon. Most importantly, template polymerization can be performed to overcome the disadvantages of reduced bioactivity and inhomogeneity of chemically and physically crosslinked hydrogels, respectively.196 pH-responsive hydrogels via template polymerization of PAAc and DMAEMA can facilitate the immobilization of an enzyme (i.e. β-galactosidase).196
4.8. Synthesis of redox responsive hydrogels (RRHs)
SRHs have been developed with the ability to sense reactive oxygen species (ROS), which facilitate cell protection against oxidative stress. These SRHs, herein designated as RRHs, can sense and eliminate the ROS produced from cellular metabolism. ROS are highly reactive reagents and abundantly present in the human body. They play a significant role in cell signaling pathways but cause strong oxidative degradation to liquids, proteins, nucleic acids, etc.201,202 As a result, excessive ROS generation disrupts body functions and is responsible for systemic diseases.203–208 When a redox-responsive hydrogel is mixed with an oxidant (e.g. NaClO), its viscosity reduces and a transition into a solution phase occurs; on the contrary, when a reductant (e.g. glutathione (GSH)) is added to this solution, its viscosity increases and gelation occurs. This concept is applied to mitigate the effect of ROS. A similar mechanism was also observed through electrochemically-induced redox reactions (Fig. 16).204,209
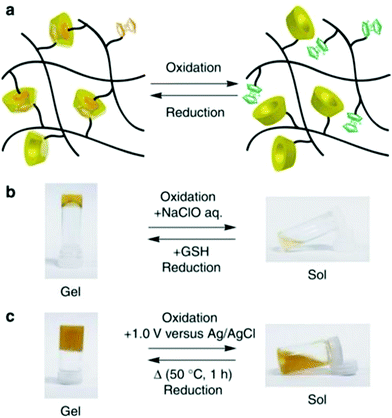 |
| Fig. 16 Redox-responsive sol–gel transition. (a) Schematic illustration of sol–gel transition. (b) Sol–gel transition against chemical reagents (NaClO and glutathione). (c) Electrochemical oxidation (+1.0 V versus Ag/AgCl) and reduction induced sol–gel transition [reprinted with permission from ref. 209, ©Springer Nature, 2011]. | |
A synthesis process of such a redox responsive hydrogel includes the incorporation of redox-sensitive entities into a temperature-responsive environment during fabrication. A study reports that the functionalization of a copolymer fabricated by ring-opening polymerization can be done via a thiol–yne reaction to incorporate the redox-sensitive characteristic in the hydrogel. Under the stimuli of chemicals like H2O2, the resulting copolymer can show redox-sensitivity.204 The inclusion of redox-sensitive organo-metallic compounds can also be applied to synthesize RRHs. For example, Nakahata et al. developed a hydrogel based on host (PAAc/β-CD)–guest (PAAc/FE) polymer chemistry by integrating redox-sensitive ferrocene (FE) with PAAc.209 According to another concept, disulphide linkage-based crosslinking is one of the popular approaches to fabricate redox-sensitive hydrogels based on thiol–disulphide exchange reactions.10
4.9. Synthesis of glucose-responsive hydrogels (GRHs)
Modern research into stimulus-responsive hydrogels has opened a new dimension towards advancements that were beyond imagination once. One of the most widespread and commercialized studies involves the synthesis of glucose-responsive hydrogels. These hydrogels can be very popularly used in advanced science such as self-monitoring of blood glucose (SMBG) to regulate the blood glucose level of diabetic patients,210,211 and as effective insulin and drug carriers. They can also be applied in the form of nanogels, microgels, micelles, vesicles, mesoporous NPs, etc.212 One of the popular synthetic methods is based on dynamic covalent linkages. A study shows that the PEO-b-PVP diblock polymer, α-cyclodextrin (α-CD), and phenylboronic acid (PBA)-terminated PEO crosslinker can simply be mixed to form a hydrogel solution. While PVA and PBA together form the crosslinker required, the inclusion occurs between PEO and α-CD. This reaction is responsible for both hydrogel formation and providing necessary stability. The hydrogel structure tends to dissolve in the presence of glucose and thus can be very effective to release loaded drug proteins in the intended environment (Fig. 17).213
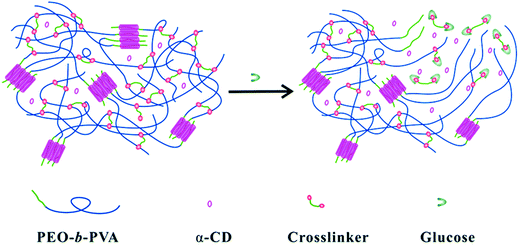 |
| Fig. 17 Schematic illustration of the glucose response mechanism of the hydrogel formed with PEO-b-PVA, α-CD, and a cross-linker [reprinted with permission from ref. 213, ©The Royal Society of Chemistry, 2014]. | |
In another study, free radical copolymerization has been employed for producing other GRHs. Preparing a stock solution of AAm and MBAAm in 4-(2-hydroxyethyl) piperazine-1-ethane sulfonic acid (HEPES) is the first step, followed by mixing N-(3-dimethyl aminopropyl acrylamide) (DMAPAAm) and N,N,N′,N′-tetramethylethylenediamine (TEMED) with the solution. Herein, 3-acrylamidophenylboronic acid (3-APB) acts as a free radical initiator. Free-radical crosslinking takes place in the solution and thus GRHs are produced. After confining the resulting hydrogel into a porous membrane, when a change in the concentration of glucose around it is brought, a transition is observed. The hydrogel senses the glucose stimulation through the porous structure.214,215
4.10. Synthesis of CO2 responsive hydrogels (CO2RHs)
The surface properties of CO2-responsive hydrogels are controllable with the stimulation of CO2. In most cases, the modification comes in the form of a reversible switching technique.216 In one-way CO2 stimuli-responsive hydrogels can be prepared by cross-linking the gels with discrete metal–organic macrocycles (MOM) or metal–organo cages (MOC). This technique has added a new magnitude of versatility in the field of supramolecular self-assembled hydrogels.
In one study, a new family of star block copolymers (SBCP) prepared with MOM as the core show CO2 responsive behavior including CO2 triggered morphology transition, CO2 promoted hydrogel formation, and even CO2-induced thermo-responsive behavior. Moreover, the bubbling of N2 results in a hydrogel with a reverse sol-to-gel transition.217–226 Such CO2 responsive hydrogels have found applications in different sectors such as CO2 capture and monitoring, separation, encapsulation, and CO2 switchable vesicles.227–232 The reversible swelling–deswelling property in the presence of a green stimulus like CO2 is a topic of interest where external stimulation can be used to exhibit responsiveness (Fig. 18).233–238
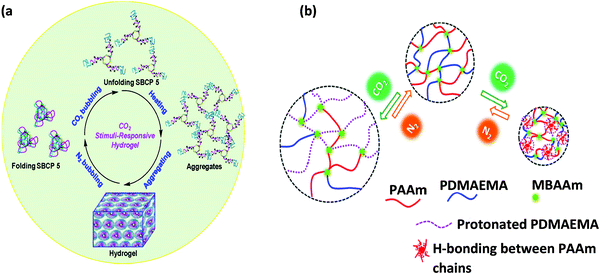 |
| Fig. 18 (a) Schematic illustration of a CO2 responsive hydrogel of a star supramolecular block copolymer (SBCP) [reprinted with permission from ref. 217, ©American Chemical Society, 2017]. (b) Schematic illustration of CO2 switchable swelling behaviors of P(AAm-co-DMAEMA) hydrogels [reprinted with permission from ref. 233, ©Springer Nature, 2018]. | |
In addition to CO2 responsive hydrogels, CO2-temperature dual stimuli-responsive hydrogels can be conveniently used in various circumstances and showed significant potential in targeted drug transport and controlled drug release. Lie et al. synthesized a CO2-temperature-responsive hydrogel from PDMAEMA with a β-CD via atom transfer radical polymerization (ATRP). This supramolecular triblock stimuli-responsive copolymer exhibits good biocompatibility with PNIPAAm and PDMAEMA hydrophilic segments and polycaprolactone (PCL) hydrophobic segments. The resultant triblock copolymer is responsive to carbon dioxide (CO2) gas and temperature reversibly through self-assembly into cell vesicles.239
4.11. Synthesis of enzyme responsive hydrogels (EZRHs)
Since all the major changes occurring in living cells are because of enzymes, in the field of artificial materials enzymes are being used as a trigger to create biomimetic responsive materials. These include hydrogels that not only undergo structural changes but also interact with the environmental components upon exposure to different enzymes.36–40 To synthesize novel responsive polymers, enzyme and enzyme-catalyzed reactions have turned out to be potential triggering elements.38,240,241 Enzymes are biological substances that act as a natural trigger. Since enzymes are biomolecules, it is possible to release as per requirement wherever necessary by controlling physical attributes and harmonizing the material response.242 Enzyme responsive hydrogels with low molecular weight gelators (LMWG) are recent developments in this field as the enzymatic process involves adaptation and reorganization of the gel surface.36,243
For the synthesis of EZRHs, one of the most popular techniques is the incorporation of an enzyme-catalyzed reaction. Here, an enzyme acts as a key biological catalyst capable of bringing about chemical or morphological changes within hydrogel cells. It can be used to obtain enzyme-mediated bio-responses, especially in the biomedical field. To synthesize such EZRHs, there are mainly three basic requirements. The hydrogel must contain embedded enzyme recognition elements like linkers. Next, the linkers must be accessible by the enzyme to ensure the enzyme-catalyzed reaction. Finally, the enzyme linker reaction imparts physical or chemical changes, i.e. degradation or morphological transitions.242,244,245
Another concept is that some hydrogels can release trapped biomaterials in the presence of an enzyme. This is possible due to the disintegration of hydrogel-forming polymer chains. Hydrogels with such characteristics can be synthesized in two ways: firstly, by a simple covalently cross-linked network of the normal polymer and secondly in the form of a supramolecular structure that takes place among multiple self-assembling molecules (hydrogelators). The hydrogelators interact to form nanofiber structures. The intermingling of these nanofiber structures is responsible for forming a hydrogel network in this way.243,246–248
The graphical illustration (Fig. 19) shows the action of the hydrogels in the presence of an enzyme. The hydrogel contains a linkage itself which links with the provided enzyme and brings about morphological changes in the hydrogel structure. This is applicable in both the intracellular cytoskeleton and extracellular matrices (ECM). This is an example of bio-simulation or bioactivation.243,249,250
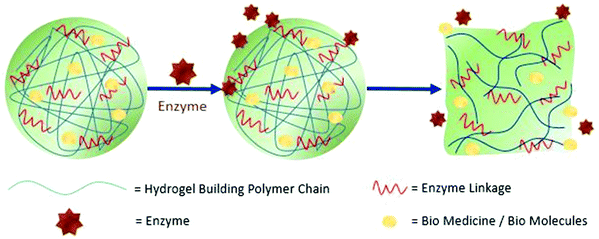 |
| Fig. 19 Hydrogel responding to the enzyme and mechanism to release biomolecules [reproduced with permission from ref. 242, ©SAGE Publications, 2016]. | |
Generally, the chemical synthesis process of a hydrogel includes covalent cross-linking among polymer chains. The formation of the hydrogel is done via two principal steps. Initially, the biodegradable component is dissolved into a liquid state.84,251,252 The physical hydrogel can be produced by ensuring physical interactions or cross-linking among biological components. As we know, some enzyme-responsive polymers typically contain enzyme reactive groups either in their main chain or as additional side groups. They exist in the form of labile linkages. These linkages cause modification and tuning of the transformation in the structure via different non-covalent interactions triggered by enzymes.84,241 Some of the physical networking processes are shown in Fig. 20.
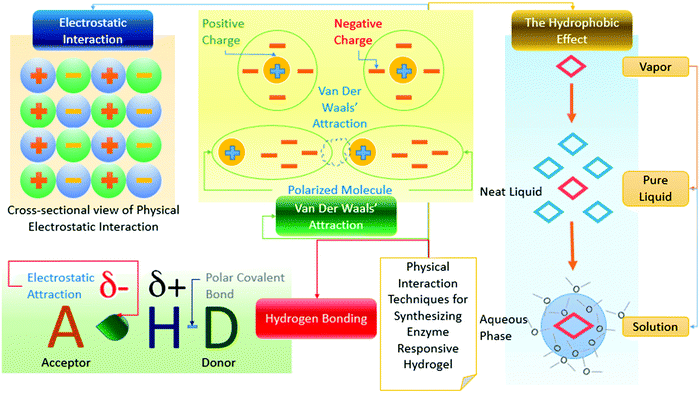 |
| Fig. 20 Physical interaction processes for producing enzyme responsive hydrogels (based on ref. 84, 241 and 253). | |
Enzymes are specific in their actions and activated by hydrogel degradation. They are absorbed as biocatalysts to regulate the controlled release of drugs and biomolecules at desired locations. For a polymer to be enzyme responsive, some conditions must be met; for instance, an enzyme responsive substrate, a compound having a high influence on the kinetics of the enzyme–catalyst interaction. Finally, the enzyme–substrate reaction requires altering the material properties.254 Enzymatic action on the substrate as a consequence of the molecular interaction in terms of forming different chemical bonds such as hydrogen bonds, electrostatic interactions, van der Waals interactions, hydrophobic interactions, π–π interactions, or any other combination of these bonds triggers the alteration of these surface properties (supramolecular architectures, self-assembly and swelling/shrinkage of hydrogels).255 A study shows the incorporation of urea and rhodamine 6G (RH6G) to formulate a urea-based hydrogel that demonstrates a typical sol-to-gel phase transition due to enzymatic hydrolysis in the presence of the enzyme β-galactosidase (β-Gal).256 Another study reports a hydrogel formed of covalent cross-linking between the β-(1 → 4) linked D-glucose unit and chitin which can be incorporated for targeted medication release in response to 2-acetamido-2-deoxy-β-D-glucose.84
4.12. Synthesis of antigen responsive hydrogels (ARHs)
Antigen responsive hydrogels exhibit a volume or mass transition in the presence of an antibody or its supplementary. Physical entrapment of the antigen in the hydrogel network, chemical conjugation of the antigen, and the utilization of the antibody–antigen pair as a reversible cross-linker are usually employed to fabricate them with great potential for application in biosensors.14,41
In the field of supramolecular hydrogels, it is possible to prepare an antigen-responsive structure. LMWG can be manipulated to exert self-assembly to construct supramolecular hydrogels. The stability of a supramolecular hydrogel capsule in a cell culture medium allows it to further upgrade to an enzyme and a cell responsive hydrogel, which possesses an appropriate mechanism to release fluorescent drugs stimulated by prostate specific antigen (PS)-catalyzed proteolysis. It has good potential for application in the diagnosis of prostate cancer.15,257–260
A hydrogel-based smart membrane can be produced by grafting the dextran backbone in a fluorescein isothiocyanate (FITC) antigen and a sheep anti-FITC IgG antibody where the bio-specific interactions between IgG and FITC ensure additional stabilization of the structure.261 A recent study demonstrates the synthesis of a hydrogel from the copolymerization of IgG2a and NIPAAm through redox initiation and chemical crosslinking via MBAAm.14 Using a similar mechanism of change in their crosslinking density in response to target molecules, a target responsive hydrogel can be engineered.16 This novel hydrogel shows great potential for quantitative point-of-care testing with higher sensitivity and accuracy in detecting cocaine, ochratoxin A, and lead ions. The hydrogel was constructed as described in earlier studies262–264 with DNA grafted linear PAAm and cross-linking DNA for targeted recognition. The mechanism of this action is presented in Fig. 21. The hydrogel was formed upon the hybridization of polymer strands and linker DNA (aptamer or DNAzyme). The hydrogel response to the target substance allows the release of the preloaded Pt NPs, which have good stability and excellent catalytic ability for decomposing H2O2 to O2. Then, the generated O2 in an enclosed environment leads to a significant pressure increase, which is rapidly traceable by a pressure meter (Table 2).265
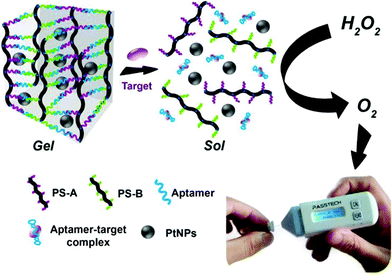 |
| Fig. 21 Working principle of the target-responsive hydrogel pressure-based assay [reprinted with permission from ref. 265, ©American Chemical Society, 2017]. | |
Table 2 Recently developed temperature, light, electricity, and pH-responsive hydrogels
Responsive to |
Synthesis technique |
Crosslinking method |
Main materials |
Application area |
Ref. |
Abbreviations (temperature responsive hydrogels): poly(acrylic acid)-graft-β-cyclodextrin (PAAc-g-β-CD); and polyacrylamide (PAAm); ammonium persulfate (APS): poly(propylene oxide) (PPO); poly(ethylene oxide) (PEO); polycaprolactone (PCL); N-isopropylacrylamide (NIPAAm); 2-hydroxyethyl methacrylate (HEMA); dimethyl-γ-butyrolactone acrylate (DBA); tris(2-(dimethylamino)ethyl) amine (Me6TREN); dimethyl formamide (DMF); tetrahydrofuran (THF); phosphate bufferer saline (PBS); poly(N-vinylcaprolactam) (PNVCL); sodium alginate (Na–Alg); N,N,N′,N′-tetramethylethylenediamine (TEMED); ethylene oxide (EO). Abbreviations (light responsive hydrogels): N-isopropylacrylamide (NIPAAm); acrylic acid (AAc); ammonium persulfate (APS); azobenzene-branched polyacrylic acid (AzBPAAc); deoxycholate-β-cyclodextrin (D-β-CD); N,N′-methylenebisacrylamide (MBAAm); graphene oxide (GO); glycidyl methacrylate (GMA); dimethyl sulfoxide (DMSO); polypyrrole (PPy), 4-dimethylaminopyridine (DMAP). Abbreviations (electrical stimuli responsive hydrogels): Al(OH)3 nanoparticles (AH NPs); acrylic acid (AAc); N,N-dimethyl-acrylamide (DMAAm); N,N′-methylenebisacrylamide (MBAAm); graphene oxide (GO); acrylamide (AAm); sodium alginate (Na–Alg); potassium peroxodisulfate (KPDS); N,N,N′,N′-tetramethylethylenediamine (TEMED); micro-fluidic spinning (MFS); benzoin dimethyl ether (BDK); 2-acryloylamino-2-methyl-1-propanesulfonic acid (AMPS); PF127 diacrylate (PF127DA); dimethyl sulfoxide (DMSO); 2-hydroxy-2-methylpropiophenone (HMPP); 3-acrylamidophenylboronic acid (PBA); polydimethylsiloxane (PDMS); N,N-dimethylamino ethyl acrylate (DMAEA). Abbreviations (pH responsive hydrogels): poly-(L-lactide)-co-polyethyleneglycol-co-poly(L-lactide)dimethacrylates (MA–PLLA–PEG–PLLA–MA); acrylic acid (AAc); N-isopropyl acrylamide (NIPAAm); 2-(dimethylamino)ethyl methacrylate (DMAEMA); N,N′-methylenebisacrylamide (MBAAm); N,N,N′,N′-tetramethylethylenediamine (TEMED). Abbreviations (magnetically responsive hydrogels): N-isopropylacrylamide (NIPAAm), acrylamide (AAm), O-acetyl galactoglucomannan (AcGGM). Abbreviations (sound responsive hydrogels): polyethylene glycol (PEG); polyethylene glycol-block-poly(lactic-co-glycolic acid) (PEG-b-PLGA); phosphate buffered saline (PBS); N-hydroxysuccinimide (NHS); poly(ethyleneimine) (PEI); poly(methacrylic acid) (PMAAc); poly(N-vinylpyrrolidone) (PNVP); 1-ethyl-3-(3-(dimethylamino) propyl)-carbodiimide hydrochloride (EDC); ethylenediaminetetraacetic acid sodium salt (EDTA); polyvinyl alcohol (PVA); N-isopropylacrylamide (NIPAAm); bovine serum albumin (BSA); N,N′-methylenebisacrylamide (MBAAm); ammonium persulfate (APS); N,N,N′,N′-tetramethylethylenediamine (TEMED); cyclodextrin (CD); dimethylformamide (DMF); dimethyl sulfoxide (DMSO). Abbreviations (glucose responsive hydrogels): phenylboronic acid (PBA); polyethylene glycol (PEG); acrylamide (AAm); 2-hydroxyethyl methacrylate (HEMA). |
Temperature |
IPN method |
Chemical crosslinking |
PAAc-g-β-CD and PAAm |
Drug delivery |
266 |
Grafting |
Physical crosslinking |
Xyloglucan and xyloglucan/pectin |
Oral drug delivery |
96 and 267 |
PEO/PPO block copolymerization |
N/A |
PO and EO |
Biomedical engineering |
268 |
Atom transfer radical polymerization |
Covalent crosslinking |
PCL, NIPAAm, HEMA, DBA, and Me6TREN |
Myocardial injection |
269 |
Radiation polymerization |
Chemical cross-linking |
PNVCL |
Drug release |
270 |
Graft copolymerization |
Chemical cross-linking |
Phenolated alkali lignin, NIPAAm |
Tissue repair, drug release, and water purification |
271 |
Full-IPN hydrogels |
Chemical cross-linking |
NIPAAm and Na–Alg |
Biomedical engineering |
272 |
|
Light |
Surfactant-free emulsion polymerization (SFEP) |
Covalently crosslinking |
NIPAAm, AAc, and Au NPs |
Drug delivery |
124 |
Branched copolymerization and dissolution |
Crosslinking via a supramolecular complex |
Deoxycholate-β-CD and (AzBPAAc) |
Light controlled encapsulation or release of molecular and cellular species |
125 |
Photopolymerization in dispersion |
Chemical crosslinking via MBAAm |
GO, GMA, NIPAAm, and DMSO |
Microelectromechanical systems, microfluidic devices, and lab on chips |
126 |
Dissolution, mixing, and gelation |
Physical crosslinking |
PPy NPs, agarose, and alginate |
Biological applications |
121 |
Photopolymerized chemical crosslinking |
Crosslinking via MBAAm |
NIPAAm, AAc, and spiropyrans |
Microfluidic devices |
123 |
|
Electricity |
Photo-initiated free radical polymerization and physical crosslinking or chemical crosslinking |
Crosslinked by AH NPs or MBAAm chemical crosslinking |
AAc and DMAAm |
Sensors, actuators, switches, and artificial muscles |
273 |
MFS and photo-initiated polymerization and chemical crosslinking |
Chemical crosslinking by MBA |
GO, AAm, Na–Alg, and CaCl2 |
Artificial muscle actuators |
274 |
In situ free-radical polymerization and chemical crosslinking |
Chemical crosslinking by the macro-crosslinker PF127DA |
AAm & AMPS |
Sensors, actuators, switches, and artificial muscles |
275 |
Photo-initiated free radical polymerization and chemical crosslinking |
Chemical crosslinking by MBAAm |
AAm, 98% PBA, DMSO, and 97% HMPP |
Glucose monitoring |
276 |
|
pH |
TEMPO-laccase catalytic redox system |
Dynamic imine bonds (i.e. Schiff base reaction) |
Chitosan (CHT) |
Medicine and food |
277 |
Photo-initiated free radical polymerized chemical crosslinking |
Crosslinking by the macro-crosslinker MA–PLLA–PEG–PLLA–MA |
MA–PLLA–PEG–PLLA–MA, AAc and NIPAAm |
Drug delivery, oral drug administration, and antimicrobial |
278 |
Dissolution & chemical crosslinking |
Chemical crosslinking by glutaraldehyde |
CHT, PVA, and humic acid (HA) |
Biotechnological applications and drug delivery systems |
279 |
Free radical polymerized chemical crosslinking |
Chemical crosslinking by a macro-crosslinker (MLS) |
NIPAAm, itaconic acid (IA) |
Release of pesticides or drugs |
280 |
Dissolution & chemical crosslinking |
Schiff base reaction |
Chitosan, dihydrocaffeic acid (DA), and oxidized pullulan (OP) |
Mucoadhesive drug delivery systems and antimicrobial |
281 |
Photo-initiated free radical polymerized chemical crosslinking |
Chemical crosslinking by MBAAm in PAAc and physical crosslinking in PVA |
PAAc, gelatin powder, and PVA |
Medical transplantation, tissue engineering, and sensor technology |
282 |
Free radical polymerized physical crosslinking |
Physical crosslinking |
AAc and DMAEMA |
Drug release and biomedical industry |
193 |
|
Magnet |
Spontaneous localization at the interface of magnetic particles |
Self-assembly of inorganic colloidal particles at a liquid–liquid interface |
Iron oxide (Fe2O3), NIPAm, and AAm |
A delivery system for biomolecules, drugs, food supplements, cosmetics, living cells, etc. |
161 |
Hybrid hollow spheres by infiltration with a TiO2 precursor |
Organic–inorganic hybrid hollow sphere hydrogel |
TiO2, polystyrene |
Drug release system, containers for catalyst components |
283 |
A one-pot methodology for developing a hemicellulose-based hydrogel by covalent cross-linking |
Hemicellulose-based hydrogel |
Fe3O4 magnetic nanoparticles, TiO2-co-pectin, AcGGM, Fe3O4, FeCl2, FeCl3·6H2O |
Biomedical field of tissue engineering applications, controlled drug delivery, magnetically assisted bioseparation |
284 |
Magnetic nanoparticles incorporated as a multi-functional cross-linker |
A ferrohydrogel synthesized from magnetic nanoparticles and the PAAm hydrogel network |
Siloxane-based unsaturated methacrylic group, CoFe2O4, PAAm |
Soft actuators, separation devices, etc. |
285 |
|
Sound |
Membrane emulsification method followed to prepare the drug and then an amidation reaction to form the hydrogel patch |
Four-armed PEG-based hydrogel patches containing diclofenac sodium (DS) loaded polyester microcapsules |
DS, PEG-b-PLGA, PBS, 4-arm PEG–NH2 and 4-arm PEG–NHS |
Transdermal drug delivery |
286 |
Cross-linking process |
Crosslinked alginate hydrogels |
Alginate polymer, calcium sulfate |
Cancer therapies, drug release with potential therapeutic benefits |
287 |
Shaking, deposition, and cross-linking |
Multilayer hydrogel microcapsules |
DNA loaded calcium carbonate, PEI, PMAAc, PNVP, EDC, EDTA |
Nucleic acid drug release. Also, the developed hydrogel can be applied for controlled delivery of sensitive biomolecules, as a carrier for DNA vaccines, and in cancer or tissue generation therapies |
288 |
Mixing of drug and PVA, followed by freezing |
Composite hydrogel |
PVA, silicone oil, Teflon mold |
Hydrophobic drug release |
289 |
Cross-linking copolymerization |
NIPAAm based reusable hydrogel |
AAc, NIPAAm, BSA, MBAAm, APS, TEMED |
Controlled and accelerated release of large drug molecules |
290 |
N2 atmosphere, precipitation, centrifugation, and decantation |
Supramolecular polymeric hydrogels |
PEG, NHS-activated carboxylic acid group and amine group-modified β-CD, anhydrous DMF |
Protein drug release |
291 |
Block copolymerization |
Injectable thermoresponsive biocompatible hydrogels |
D,L-Lactide and glycolide, methoxy-PEG (mPEG), tin(II) 2-ethyl hexanoate, dry toluene. PLGA, DMSO |
One-week controlled drug release |
292 |
|
Glucose |
Reversible covalent interaction |
Shear-thinning and self-healing glucose-responsive hydrogels |
Phenylboronic acid (PBA), cis-diols, PEG, hexafluorophosphate benzotriazole tetramethyl uronium (HBTU) activated 4-carboxy-3-fluorophenylboronic acid (FPBA) or 4-carboxyphenylboronic acid (PBA) |
Delivery of protein therapeutics, 3D cell culture substrate, biocompatible substance, etc. |
293 and 294 |
Complex formation between PBA and diols |
Boronate gel-based insulin delivery hydrogel |
PBA derivatives, 1,2 or 1,3-cis-diols |
Artificial pancreas, non-electric insulin delivery system |
295 |
Incubation of glucose/galactose binding protein in the acrylamide hydrogel network |
Glucose-responsive hydrogel network based on protein recognition |
Glucose/galactose binding protein (GBP), AAm |
Controlled drug delivery, implantable drug delivery system |
296 |
Copolymerization of a glucose-responsive monomer with the main monomer |
Glucose-responsive hydrogel with a semiconductor-based field-effect transistor (FET) |
HEMA and vinylphenylboronic acid (VPBA) |
Biosensor for eye contact lens, sheets adhering to the skin, etc. |
210 |
|
Enzyme |
Entrapping cationic organic substances within a supramolecular amphiphilic urea-based hydrogel |
Gel-to-sol phase transition-based hydrogel due to enzymatic hydrolysis |
Urea, rhodamine 6G (RH6G), β-galactosidase (β-Gal) |
Carrier for cationic biomaterials and dyes |
256 |
Incorporation of modified gelatin, a cleavable protease sequence, and hyaluronic acid (HA) into a cell-laden gel network |
Dynamic gelatin–hyaluronic acid (HyA) hybrid hydrogel |
Gelatin, carbic anhydride, high purity alumina (HPA) |
3D cell culture platforms, tumor microenvironment, activation of pancreatic stellate cells |
297–301 |
Covalent cross-linking between cellulose and chitin |
Covalently cross-linked hydrogel prepared via dissolution or gelation |
β-(1 → 4) linked D-glucose unit, chitin, 2-acetamido-2-deoxy-β-D-glucose |
Drug delivery (controlled release and targeted release), tissue engineering, wound dressing, water purification, supporting materials for functional additives, thermal insulators, etc. |
84 |
GOx-mediated radical initiation and polymerization technique |
Enzyme-mediated radical polymerized bioactive hydrogel |
Glucose oxidase (GOx), β-D-glucose, Fe2+, N-hydroxyiamide compound, N-hydroxy-5-norbornene-2,3-dicarboximide (HONB) |
Cell-specific drug delivery, drug carrier |
302 |
The list of recently developed major stimuli-responsive hydrogels is given below.
5. Synthesis of hydrogels for targeted applications
Functional hydrogels are programmed by surface modifications that can partly endow hydrogels with desired properties while retaining the original intrinsic physicochemical properties of the hydrogel network. This surface functionalization is considered as an alternative strategy for the fabrication of multifunctional hydrogels for targeted applications.303,304 Functionalization of the surfaces of hydrogels can be done in three ways: the construction of a surface structure, physical incorporation of functional micro/nanomaterials, and chemical grafting.127,305–313
The properties of hydrogels are easily tunable by modifying functional groups314 and incorporating functional materials.315–317 Thus, hydrogels that possess important features including self-healing170,318 and extreme flexibility319–323 with superior mechanical properties324–326 and stretchability327,328 can be synthesized.
5.1. Hydrogels for superior mechanical applications
Hydrogels have immense applications in tissue engineering, wastewater treatment, wound healing, antimicrobial applications,329 and controlled drug release, but the lack of mechanical strength has limited their applications substantially.324,325 The bio-mimicking ability of hydrogels have made researchers explore the means of enhancing the mechanical properties of hydrogels. Since soft tissues such as tendons, ligaments, and other supporting tissues are tough materials, which are vulnerable to injury and incapable of regenerating spontaneously, materials with similar toughness and hydration properties could be a great choice for remodeling such injuries.326 To utilize hydrogels in mechanical strength demanding applications, increasing the toughness of hydrogels is a prerequisite. Many of the reported hydrogels are weak, brittle, and have low toughness, which somewhat limits their applications.330,331 Therefore, techniques of double networking,332 interpenetrated networking,329 triple networking,333 synergistic copolymerization effects,334 and nano-additive enforced networking325 have been employed to tune the mechanical properties of hydrogels. For instance, Yan et al. developed double networked hydrogels of PAAm and gelatin reinforced with GO nanosheets (gelating/PAAm/GO) (Fig. 22a). Due to the hydrophilic nature of GO, it functions as a multi-crosslinker with PAAm and can interact with gelatin via electrostatic and/or hydrogen bonding interactions, which significantly increases its elasticity, fracture stress/strain, and softening properties relative to the neat PAAm and gelatin/PAAm hydrogels. The presence of GO made the hydrogel denser, resulting in the reduction of the swelling property. Additionally, the interactions between GO and gelatin caused the deterioration of self-recovery properties.332 A semi-interpenetrating networked hydrogel of BC and CHT (Fig. 22b) was developed by Wahid et al. The CHT part of the hydrogel was cross-linked by glutaraldehyde, while the BC part adhered via hydrogen bonding. The resulted hydrogel demonstrated better mechanical and thermal properties than the neat hydrogels of BC and CHT. The scanning electron microscopic image exhibited a dense network structure, which could be due to the enhanced entanglement of bacterial cellulose in cross-linked chitosan networks.329 Triple-networked (Fig. 22c) hydrogels of PVA, PVP, and PAAc–Zn2+ obtained via similar physical–chemical crosslinking techniques were developed by Li et al. Formation of hydrogen bonding between PVA and PVP chains along with chemical-crosslinking of PAAc resulted in triple-networked hydrogels with superior tensile and frictional properties to those of neat PVA and PVP/PVA hydrogels.333 A combination of hydrophilic and hydrophobic polymeric segments can endow hydrogels with versatile properties. For example, hydrogel containing hydrophobic rich segments can provide hydrogel with stiffness and strength, whereas low hydrophilic segments can provide properties like stretchability and toughness. A mechanically enhanced copolymeric hydrogel fabricated from hydrophobic phenyl acrylate and hydrophilic AAm monomers was reported by Mredha et al. (Fig. 22c) Formation of lower water content and higher phenyl acrylate containing hard regions with high water content and higher AAm containing soft regions was observed.334 The presence of nanomaterials within hydrogel networks can significantly enhance the mechanical properties of hydrogels. The hydrogels reported by Chen et al. incorporated lignin nanoparticles within PAAm hydrogel networks. Presence of the lignin nanocomposite endowed the hydrogels with better compressive stress enabling them to distribute the applied load efficiently. The improvement was mainly due to the presence of nanonetworks and hydrogen bonding between the hydrogel forming materials.325
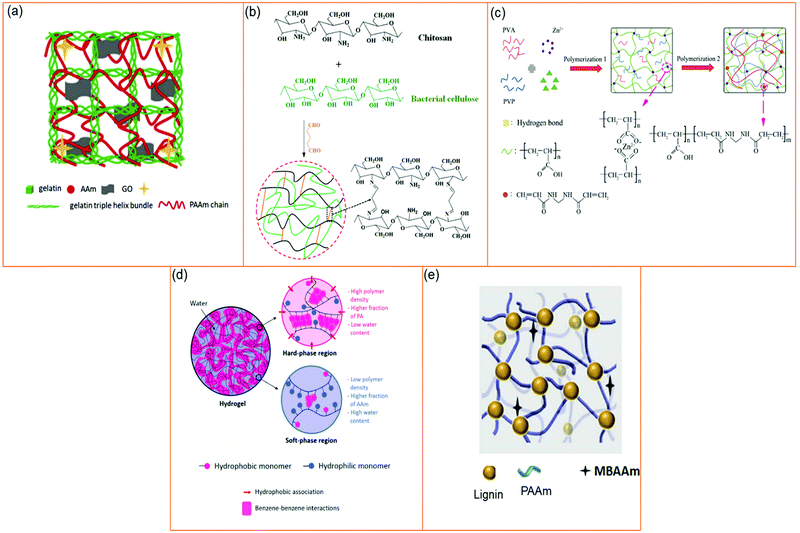 |
| Fig. 22 Means of tuning the mechanical properties of hydrogels. (a) Double networking [reprinted with permission from ref. 332, ©Elsevier, 2018]. (b) Interpenetrated networking [reprinted with permission from ref. 329, ©Elsevier, 2019]. (b) Triple networking [reprinted with permission from ref. 333, ©Elsevier, 2019]. (d) Synergistic effect of copolymers [reprinted with permission from ref. 334, ©Elsevier, 2018]. (e) Nano-additive enforced networking [reprinted with permission from ref. 325, ©Elsevier, 2019]. | |
To use hydrogels in loadbearing areas, researchers have developed numerous methods, such as chemical cross-linking, hydrogel networking (i.e. interpenetrating and double networking), and nano-additives, to improve their mechanical properties.324–326Table 3 summarizes the synthesis methods/techniques, hydrogel types, process flow, test results, and applications of several hydrogels with superior mechanical properties.
Table 3 Preparation of hydrogels with superior mechanical properties
Synthesis method/techniques |
Hydrogel type |
Cross-linking method |
Main materials |
Applications |
Ref. |
Abbreviations: N,N′-methylene bis-acrylamide (MBAAm); sodium dodecyl sulfate (SDS); regenerated silk fibroin (RSF); methacrylic acid (MAAc); N-(pyridine-2-yl) acrylamide (NPAM); dimethyl sulfoxide (DMSO); bacterial cellulose (BC); methyltrimethoxysilane (MTMS); polyvinylpyrrolidone (PVP). |
Chemical cross-linking |
Cellulosic nanomaterials (cellulose nanocrystals, bacterial cellulose fibers, and TEMPO-oxidized cellulose nanofibers) dispersed interpenetrating hydrogels |
Ionic cross-linked networks |
PAAm, polyelectrolyte Na–Alg |
Wastewater treatment |
324 |
Quinone |
Nanofiber-reinforced gelatin |
Chemical cross-linking |
Deacetaldehyde chitin nano-fibrillated suspension, DI water, PTFE membrane |
Agricultural and biomedical fields |
326 |
Physical cross-linking |
RSF based double networked hydrogels |
RSF/SDS network |
AAm, Irgacure 2959, SDS, NaCl, RSF, PTFE spacer |
Strain sensors, touch screen pen, and the electronic skin of artificial robots |
335 |
Physical cross-linking |
The 3D-printed tough hydrogel of MAAc and NPAM |
Hydrogen bonded cross-linking |
DMSO, MAAc, NPAM, photoinitiator IRGACURE 2959 |
Soft robots, implant devices, and tissue engineering |
336 |
Chemical–physical crosslinking |
BC and CHT semi-interpenetrating networked hydrogels |
Glutaraldehyde cross-linked network |
BC membrane, CHT, glutaraldehyde |
Antibacterial applications |
329 |
Chemical–physical crosslinking |
PAAm/lignin nanoparticle nanocomposite hydrogels |
Free radical networking in lignin nanoparticle dispersion |
PAAm, MBAAm, ascorbic acid, hydrogen peroxide |
Tissue engineering or regeneration, artificial muscles, strong underwater antifouling materials |
325 |
Chemical–physical crosslinking |
Silicon dioxide/PVA composite hydrogels |
Injection molding of the hydrolyzed solution |
PVA, MTMS, HCl |
Artificial articular cartilage, drug delivery, and biosensors |
337 |
Chemical cross-linking |
Hydrophobic and hydrophilic monomer-based hydrogels |
MBAAm cross-linked network |
Phenyl acrylate, AAm, DMSO medium, MBAAm, 2,2-azobisisobutyronitrile |
Diverse load-bearing applications |
334 |
Chemical–physical crosslinking |
Triple-network hydrogels of PVP/PVA/PAAc |
MBAAm cross-linked network |
PVP, PVA, AAc, MBAAm, ZnCl2 |
Engineering applications with better mechanical properties and lower friction coefficients |
333 |
Chemical cross-linking |
Non-swellable gradient hydrogels |
HCL cross-linked network |
Sodium pyrophosphate dispersion, LAPONITE®, PAAm, HCL |
Biomedical applications |
338 |
5.2. Hydrogels for antimicrobial applications
Tailoring high-performance and multi-functional properties of hydrogels, especially natural polymer hydrogels, has drawn attention recently.339,340 Different types of techniques like double-network hydrogels,341 nanocomposite hydrogels,342 macromolecular microsphere composite hydrogels,343 polyampholyte hydrogels, etc.344,345 have been developed to impart functional properties. The produced hydrogels have the functional properties of high swelling in water, soft pliable nature, elastic properties, self-healing performance, and compatibility with human physiology. These improved properties have added a new dimension in the field of drug delivery, tissue engineering, food packaging products, cosmetics, and biomedical engineering.346–350 Many studies were performed to develop hydrogels as alternative materials for antibacterial applications. For example, the desired properties of hydrogels, such as hydrophilicity and porosity, can be integrated by selecting the types of monomers and crosslinkers. Moreover, some types of hydrogels also have inherent antibacterial properties. Based on the hydrogel matrices and antibacterial agents, antibacterial hydrogels are divided into (i) inorganic nanoparticle-containing hydrogels, (ii) antibacterial agent-containing hydrogels, and (iii) hydrogels with inherent antibacterial capabilities.351,352 However, antimicrobial hydrogels can be synthesized in the ways mentioned in Fig. 23.
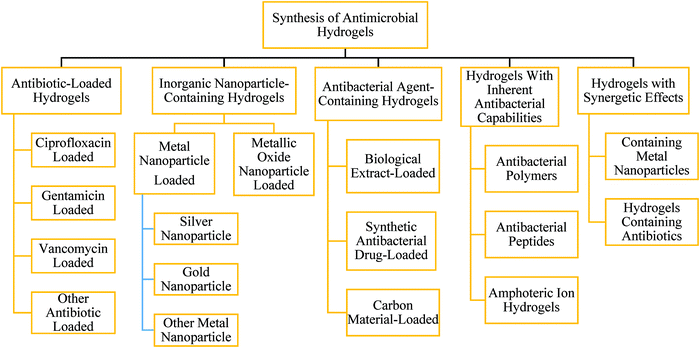 |
| Fig. 23 Synthesis of antimicrobial hydrogels (based on ref. 351). | |
Incorporation of metals is one of the most pristine and widely used techniques to functionalize antimicrobial properties, but with the recent development of nanoscience, the incorporation of metals, especially Ag, Au, Cu, and Zn, with hydrogels is done mainly in the form of NPs.351,353 Ag is most widely used due to its good antibacterial properties and relatively low toxicity. However, other metals, such as gold, copper, and zinc, have their advantages and provides antibacterial properties as well.352 It is hypothesized that the interaction between silver ions (Ag+) and the thiol group in proteins of the bacterial cell membrane is responsible for affecting the bacterial cell viability by inhibiting the replication of DNA (Fig. 24).351
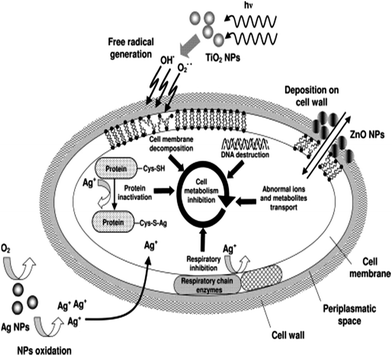 |
| Fig. 24 Antibacterial mechanisms of metals and metal oxide NPs [reprinted with permission from ref. 354, ©Elsevier, 2013]. | |
Both natural and synthetic polymers are used as the matrix of hydrogels containing Ag NPs. Polysaccharides, mainly alginate, chitin, CHT, and CMC, are common natural polymers.351 The antimicrobial action of CHT is more rapid against fungi and algae than toward bacteria.354–356 The resistance against microbes depends on several intrinsic factors such as the type of CHT,357 the degree of CHT polymerization, the host, the natural nutrient constituency, the chemical or nutrient composition of the substrates or both, and the environmental conditions (e.g., substrate water activity, moisture or both).358
Both chitin and CHT have antimicrobial and metal-binding properties. Chitin- or CHT-based hydrogels such as the CHT/2-glycerophosphate/nano-silver hydrogel and silver molybdate nanoparticle/chitin matrix (Ag2Mo2O7/chitin) hydrogels are resistant to E. coli.359,360 The other polysaccharide hydrogels include the iota-carrageenan-based Ag NP hydrogel and the Ag NP-loaded PVA/gum acacia hydrogel; both actively show good antibacterial activity against the Gram-negative bacterium E. coli.361,362 The recent developments of antibacterial hydrogels incorporating metal particles and their oxides are summarized in Table 4.
Table 4 Information on hydrogels with different metal NPs
Metal |
Synthesis technique |
Hydrogel matrix |
Bactericides |
Applications |
Ref. |
Abbreviations: FA, fumaric acid; GA, gum acacia; HEMA-2, hydroxyethyl methacrylate; IPN, interpenetrating polymer network; MRSA, methicillin-resistant S. aureus; PBS, phosphate buffered saline; PMAAc, poly(methacrylic acid); QPVA, quaternized polyvinyl alcohol; RGO, reduced graphene oxide; NW, nanowires; AAc, acrylic acid; SF, silk fibroin; CMCh, carboxymethyl chitosan; κC, κ-carrageenan; PEGMA, poly(ethylene glycol) methyl ether methacrylate; Ni NPs, nickel nanoparticles; AG-N3, 4-azidobenzoic agarose; NaCMC, CMC sodium salt; HAP, hydroxyapatite. |
Ag NPs |
Electrochemical synthesis and rehydration |
Alginate nanocomposite hydrogels |
S. aureus, E. coli |
Potential wound dressing |
363 |
Free radical polymerization and green process |
Alginate/PVA silver nanocomposite hydrogel |
E. coli, S. aureus |
Wound dressing, water purification |
364 |
Free radical polymerization |
NIPAAm-based nanogels |
E. coli, S. epidermidis |
Biomedical applications |
365 |
Cross-linking via radical redox polymerization |
Sodium alginate-based semi-IPN hydrogels |
E. coli, S. aureus |
Drug delivery |
366 |
Synthesis at low temperature |
Thermosensitive CHT/2-glycerophosphate/nanosilver hydrogels |
S. aureus, P. aeruginosa |
Wound dressing |
359 |
Free radical cross-linking polymerization and follow-up reduction of silver nitrate |
P(MMA-co-MAA)/silver nanocomposite hydrogels |
S. aureus, B. subtilis |
Smart material |
367 |
Physical cross-linking in sodium tripolyphosphate as the cross-linker |
CHT hydrogel beads |
E. coli, S. aureus |
Potential drug delivery carrier |
368 |
NaCMC was dissolved in distilled water. FA was added to the solution |
FA cross-linked CMC hydrogel |
S. aureus, K. pneumonia |
Cotton fabric |
369 |
In situ reduction of GO using vitamin C in the presence of heat (90 °C) |
RGO-based composite hydrogel |
N/A |
Wastewater treatment |
370 |
Freeze–thaw method |
QPVA hydrogel |
E. coli, S. aureus, P. aeruginosa |
Antimicrobial dressing |
371 |
Au NPs |
Incorporation of nanosized HAP particles into porous SF hydrogels |
SF/nano-HAP hydrogel |
MRSA, E. coli |
Bone tissue engineering |
372 |
Genipin cross-linking |
Gelatin hydrogels |
N/A |
Carriers in drug delivery |
373 |
Au NPs through sodium borohydride reduction method and liposomes through the standard extrusion method |
AuC–liposome hydrogel |
S. aureus |
Microbial infections |
374 |
ZnO |
The hydrogel was made from ZnO NWs and the combination of CHT and AAc. ZnO NWs were prepared by a hydrothermal strategy using zinc acetate with NaOH as precursors and PEG as an assistant. CHT-co-AAc hydrogels were prepared by free-radical graft polymerization |
CHT-based composite hydrogel |
E. coli, S. aureus |
Biomaterials, cosmetics, food packaging |
354 |
CHT pellet was mixed with alginate solution as a cross-linker to strengthen the hydrogel through a freeze-dry process |
Alginate hydrogel |
E. coli, S. aureus, C. albicans, MRSA |
Wound infections |
375 |
ZnO–PEGMA and AG-N3 IPN nanocomposite hydrogels were synthesized under UV irradiation |
AG with IPN structure |
Gram-positive and Gram-negative bacteria |
Wound dressing |
376 |
CMCh solution cross-linked with epichlorohydrin at 80 °C |
CMCh hydrogel |
Gram-positive and Gram-negative bactericides |
Applications in biomedical fields |
377 |
Ni NPs |
Nickel NPs prepared by a hydrothermal method |
Ni NP chitin nanogels |
S. aureus |
Biomedical field |
378 |
MgO |
κC was blended with Na–CMC dissolved in distilled water |
Modified κC hydrogel |
N/A |
Drug delivery, especially in gastrointestinal tract studies |
379 |
5.3. Hydrogels for cell adhesive applications
The structure of hydrogels is analogous to that of biological soft tissues which have excellent potential to be used as scaffolds for cell growth and proliferation.380,381 A magnetic PAAm hydrogel synthesized by integrating magnetic nanoparticles into gelatin can act as a cell adhesive hydrogel interface. Furthermore, this gel can act as a multi-array cell culture matrix. The order, degree, and distribution of cell adhesives, as well as cell–cell and cell–matrix interactions throughout the multilayer matrix, determine the cell behavior including emulating the extracellular matrix (ECM). It is possible to induce a different form of hepatocyte 3D spheroids and thus transition by controlling cell adhesion affecting the cell behavior. A magnetic anisotropic hydrogel structure can be formed using Fe3O4 MNPs, AAm monomers, MBAAm, and tetraethyl ethylenediamine all mixed in water. The solution is subjected to ultrasonic agitation in a polytetrafluoroethylene (PTFE) module. PAAm gelation is induced by heating (approx. at 50 °C) after the exposure of the resulting solution to a magnetostatic field for a certain time. This hydrogel with a superior cell adhesive functionality is thought to hold great potential for anticancer drug and hypothermia therapy.382–385 A peptide modified PEG scaffold is a cell-adhesive hydrogel scaffold that can act as a vessel for human blood-driven endothelial progenitor cells (EPC). It is a potential cell source for applications like vascular tissue engineering. A mild photocrosslinking process is used to encapsulate the EPC within a PEG-based hydrogel. After cell culture and network formation to prepare EPC, a solution is formed by reacting the cell adhesive peptide ligand, arginyl glycyl aspartic acid (RGDS), with acrylate–PEG–succinimidyl valerate (Acryl–PEG–SVA) to provide purified RGDS conjugated PEG (PEG–RGDS).18 A matrix metalloproteinase (MMP) sensitive peptide sequence (PQ) was also reacted with Acryl–PEG–SVA to give the Acryl–PEG–PQ–PEG–Acryl (PEG–PQ) conjugate. A solution of both PEG–PQ and PEG–RGDS was formed by dissolving both into PBS. Later, the hydrogel was formed by exposure to white light (Fig. 25a).386,387
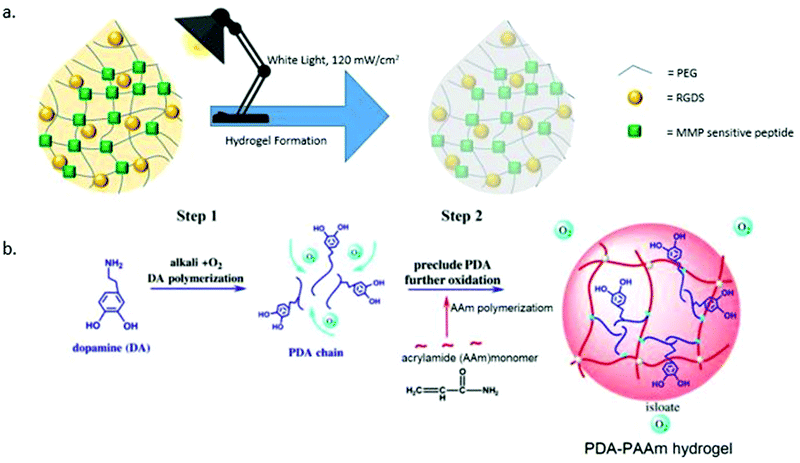 |
| Fig. 25 (a) Schematic diagram of hydrogel synthesis from the PEG–RGDS and PEG–PQ solution [reprinted with permission from ref. 386, ©Springer Nature, 2015]. (b) Synthesis of PDA–PAAm hydrogels [reprinted with permission from ref. 388, ©Springer Nature, 2017]. | |
A polydopamine–polyacrylamide (PDA–PAAm) single network hydrogel with super-stretchability, high toughness, cell affinity, and tissue adhesiveness properties was synthesized. The PDA–PAAm hydrogel was prepared in the following two steps. In the first step, PDA was prepared by oxidizing dopamine (DA) under alkali conditions. In the second step, AAm was polymerized and crosslinked to form PDA–PAAm hydrogels (Fig. 25b).388
An alginate-based cell adhesive bio-hydrogel (AdhHG) is an important potential discovery in the field of biomedical engineering. Such hydrogels are capable of encapsulating and delivering mesenchymal stem cells (MSC) to the desired location and thus contribute to tissue engineering and regenerative medicine. Cell adhesion plays an important role in both cell regeneration and sealing tissue or coating implants in the desired location. L-DOPA amino acid (DA) modified alginate hydrogels show strong adhesion to cells. First, a methacrylate alginate hydrogel is synthesized by polymerization of the methacryloyl group in the alginate chain. DA hydrochloride is integrated to induce the crosslinking ability. The adhesive property is further improved by reacting with collagen emulating short peptides to form AdhHG. Crosslinking is carried out in the Ca2+ medium. Finally, the hydrogel is formed by photo-polarization through exposure to blue-green light.389–392 Similarly, a novel peptide-based hydrogel containing cell adhesion and cell proliferation properties is expected to show inherent antibacterial characteristics and act as a potential scaffold for cutaneous wound healing. The hydrogel is synthesized from a series of peptides, Poly (Lys)x(Ala)y. These are derived from ring-opening polymerization of N-carboxylic anhydride (NCA), i.e. NCA-Lys and NCA-Ala monomers. PEG-amide succinimidyl glutarate (ASG) is chemically cross-linked with peptides to form a hydrogel exhibiting cell adhesion properties. Wound dressings containing such hydrogels provide not only resistance against infections but also a suitable physiological environment for cell generation.393–396
Another general cell adhesive hydrogel synthesis process starts with prepolymer solutions and several cross-linking methods including chemical, enzymatic, or photoreactions (Fig. 26A). For a successful tissue engineering process, an additional method is also required including tailoring biomechanical properties, tailoring degradability, tailoring bioactivity, and finally cell culture. Biomechanical properties can be improved by increasing the polymer concentration and by changing the architecture like linear, branched, etc. (Fig. 26B). The degradation properties of hydrogels can be controlled by the integration of crosslinkers in polymer networks. For instance, the incorporation of a matrix metalloproteinase sensitive peptide sequence in hydrogels makes them prone to cell-mediated degradation (Fig. 26C). The bioactivity, another important property for cell adhesion and proliferation, of hydrogels can be improved by binding bioactive compounds with hydrogels (Fig. 26D).397 For example, a collagen-binding peptide is incorporated in an alginate hydrogel.398
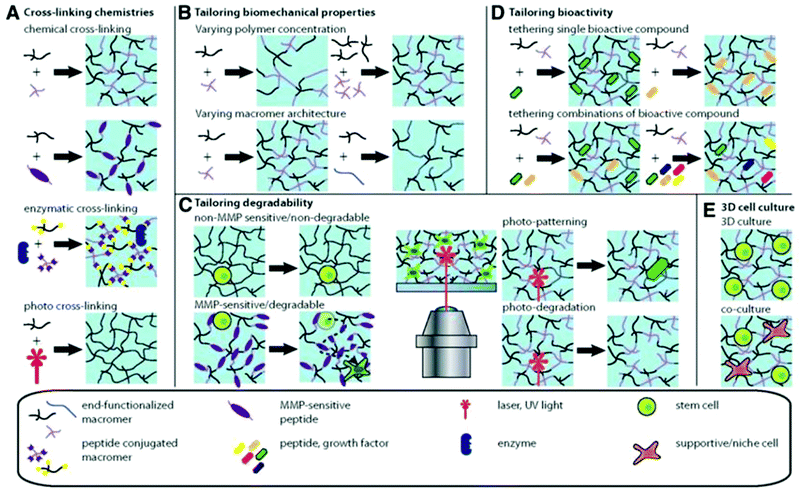 |
| Fig. 26 Schematic representation of the synthetic hydrogel for cell adhesion and proliferation. (A) Cross-linking chemistries. (B) Tailoring biomechanical properties. (C) Tailoring degradability. (D) Tailoring bioactivity. (E) 3D cell culture [reprinted with permission from ref. 398, ©AlphaMed Press, 2015]. | |
5.4. Hydrogels for self-healing applications
Self-healing is the capability of materials to recover from their damage themselves. Self-healing materials are considered as the next-generation materials for high-performance structures. This fascinating property can also be induced in hydrogels. Several underlying mechanisms of self-healing hydrogels are mainly divided into two broad categories: dynamic covalent bonds and non-covalent interactions (Fig. 27). Self-healing hydrogels possess dynamic covalent bonds that are mostly synthesized from Diels–Alder reaction, coordination bonds, imine bonds, boronate ester bonds, and disulfide bonds, whereas noncovalent interaction methods include hydrogel bonds, host–guest interactions, hydrophobic interactions, and electrostatic interactions.399
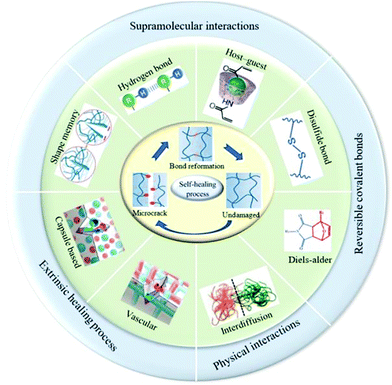 |
| Fig. 27 Chemistry of the synthesis of self-healing hydrogels.400 | |
The chemistry of self-healing can be successfully incorporated in both natural and synthetic polymers (Fig. 28). The generally used natural polymers to synthesize self-healing hydrogels are polysaccharide-based polymers including alginate, chitosan, and hyaluronic acid. Self-healing hydrogels with natural polymers are normally biocompatible. Synthetic polymers including PEG, PAAc, PVA, and PAAm give stronger and more elastic self-healing hydrogels.401
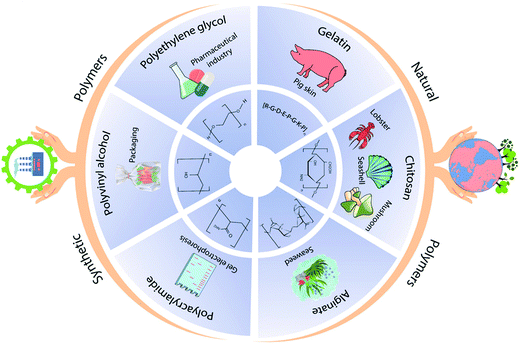 |
| Fig. 28 Synthetic and natural polymers used to synthesize self-healable hydrogels are highlighted here [reprinted with permission from ref. 401, ©John Wiley and Sons, 2019]. | |
A multifunctional composite self-healing hydrogel can be developed from a series of Li–alginate/poly(acrylamide-co-stearyl methacrylate) [Li–alginate/P(AAm-co-SMA)] hydrogels with a fully physically cross-linked interpenetrating network.402 In addition to their excellent stretchability and self-healing ability, the produced hydrogels exhibit the maximum fracture energy that enables them to be used as fire-resistant materials.403 A rapid self-healing hydrogel was developed by aligning the polymer network with dangling hydrocarbon side chains containing polar functional groups. These groups can be hydrophilic and hydrophobic moieties that allow the side chains to mediate hydrogen bonding across two separate hydrogel pieces or a rupture in the hydrogel. A polysaccharide derivative cross-linked with PVA forms a hydrogel that is capable of displaying rapid self-healing behavior when subjected to rupture. The hydrogel synthesis technique involves the extraction of bioactive laminarins (polysaccharides) from alginic acid, fucoidan, or some other seaweeds. These laminarins contain β-glucan which is modified by reacting with phenylboronic acid. Later, the resultant biopolymer and PVA are cross-linked by boronate esterification and form a self-healing hydrogel.404,405
Another type of self-healing hydrogel called a fluorochromic hydrogel that responds to light and ferric (Fe3+) ions shows decent self-healing capability. Such a hydrogel is synthesized via the physical crosslinking of PVA. γ-Cyclodextrin-spiropyran (γ-CD-SP), a fluorophore, forms hydrogen bonds with PVA and thus is integrated into the PVA gel. The presence of tannic acid creates numerous binding sites to the PVA chain. This helps to improve both the mechanical strength and self-healing ability of the hydrogel (Fig. 29).406–408
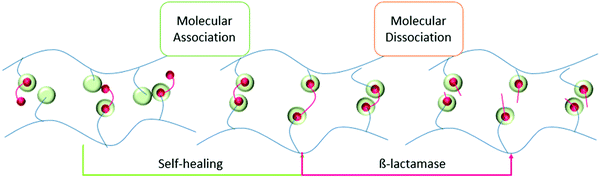 |
| Fig. 29 Schematic diagram of molecular association and dissociation [reprinted with permission from ref. 409, ©American Chemical Society, 2020]. | |
Similarly, a self-healing hydrogel based on CNC can be synthesized via a one-pot polymerization technique. Herein, sodium alginate along with CNC acts as a macro-cross-linker. The reaction is initiated by introducing the aldehyde group via mild oxidization of both CNC and sodium alginate. Next, a Schiff-base linkage is incorporated to bind the CNC surface with the oxidized sodium alginate core. The hydrogel is capable of self-healing within several hours of dissociation at room temperature.400,410
5.5. Other applications
Hydrogels are emerging materials that have attracted significant fundamental and applied interest for wastewater treatment,411 tissue engineering,325,412 drug delivery,413,414 wearable electronics,415 and energy-storage materials.314 Apart from the aforementioned synthetic processes, the following recent works of functional hydrogels have extended their applications in numerous fields (Table 5).
Table 5 Preparation of hydrogels for special applications
Special properties |
Synthesis method/techniques |
Hydrogel type |
Materials |
Applications |
Ref. |
Abbreviations: chitosan (CHT), acetic acid (AA), acrylic acid (AAc), N,N′-methylenebisacrylamide (MBAAm), polydopamine (PDA), Pluronic F127 diacrylate (PF127 DA), poly(lactide-co-glycolide) (PLGA); graphene oxide (GO); polyvinylpyrrolidone (PVP), polyvinyl alcohol (PVA), sodium alginate (Na-Alg); layered rare-earth hydroxide (LREH); sodium polyacrylate (PAAS); alginate hydrogel (Alg), carboxymethyl tamarind kernel gum (CMTKG). |
Heavy metal adsorption |
Physical crosslinking |
Cellulose-based |
Cellulose, glycine, NaOH, acetic acid |
Wastewater treatment & cartilage tissue engineering in soft electronics |
411 |
Biocompatibility, Fe3+ removal |
Ionotropic crosslinking |
Cellulose–CHT based |
CHT, AA, alkali lignin, NH4OH |
Tissue engineering, wound healing, regenerative medicine, & water treatment |
412 |
Super-absorbency |
Chemical crosslinking |
Lignin based |
Lignin, montmorillonite, K2S2O8–Na2SO3, AAc and MBAAm |
Cu(II) ion removal |
416 |
Higher compressive and tensile strength |
Physical crosslinking/in situ free radical polymerization |
PAAm/lignin NPs based |
AAm, lignin dispersion, MBAAm, ascorbic acid, and hydrogen peroxide water solution |
Tissue engineering |
325 |
Super-absorbency to gatifloxacin |
Chemical crosslinking/chemical co-polymerization |
Alginate based |
PDA particles, alginate hydrogel (Alg–PDA), ammonia aqueous solution of sodium periodate and CaCl2 |
Drug delivery |
414 |
High mechanical toughness with shape memory |
Chemical crosslinking |
Composite based |
PF127, DA, PLGA and GO |
Biomedical applications as drug carriers and antibacterial scaffolds |
413 |
Viscoelastic solid-like properties |
Chemical crosslinking |
Composite based |
DNA, synthetic hectorite (SWN), alkaline cations |
Structural applications, such as gas barrier films and load-bearing applications |
417 |
Viscoelastic |
Chemical crosslinking |
Composite based |
PVA and PVP polymer mixture, brine, resorcinol solution, formaldehyde, NaOH |
Water shut-off performance at higher temperatures |
418 |
Superabsorbent |
Cross-linked/free radical co-polymerization |
CMTKG based |
CMTKG, Na–Alg, K2S2O8, MBAAm |
Water conserver, soil conditioner and nutrient carrier |
419 |
3-D printing performance |
Physical cross-linking |
Nanocomposite based |
LREH aqueous dispersion, aqueous solutions of Na–Alg and PAAS420,421 |
Electronic skin, wearable electronics, and soft robotics |
415 |
6. Future prospects and conclusion
Hydrogels, a unique class of soft materials, have significant potential to be used in a wide range of applications including biomedicine, skin, and personal care products. Due to their significant potential, over the past few decades, rapid integration of 3D structures and easy customization with advanced synthesis techniques have spurred the popularity of hydrogels with a wide variety of applications, thus attracting research attention. To cope with these exponential demands, researchers are continuously developing new design and synthesis strategies. In this paper, some recently developed hydrogels and their fabrication techniques have been summarized. Researchers are developing several processes to synthesize stimuli-responsive and functional hydrogels. However, numerous complexities associated with hydrogels mimicking the natural 3D structure have been reported. Multi-stimuli (physical, chemical, and biological)-responsive hydrogels mimicking the natural 3D structure and improvements in their responsive characteristics could be developed in future research. Additionally, these 3D structured hydrogel fabrication techniques inevitably destroyed the intrinsic hydrophilicity and biocompatibility of a hydrogel. These research booms involve the fabrication of diverse hydrogels in combination with different polymers, which also directs to the explanations of new mechanisms of the external stimuli-responsive hydrogel. The scope for developing smart hydrogels with desired functional and intact intrinsic physicochemical properties is yet to be explored.
In particular, substantial research is required to develop novel smart hydrogels with complex but programmed self-folding, twisting, and bending behaviors. An adverse immune response occurs when hydrogen scaffolds are inserted into a body which may lead to the rejection of scaffolds. Thus, future research should focus on synthesizing such hydrogels that can control and reduce immune cells like macrophages and adjust the plasticity and reprogramming to form/repair/reconstruct tissues. Another important property of natural hydrogels is having less toxicity. However, during the manufacturing process, different solvents have been used which may increase the toxicity level. Future research should focus more on incorporating natural materials in cross-linked hydrogels. Future research should also emphasize on scaling the size of hydrogels down to the single-cell sampling level. This is important as the present sequencing in bulk samples provides information on the population level, losing key information about biological subsets within the sample. It is possible to individualize sequencing ‘omic’ data on a cell-by-cell level by partitioning single cells into separate wells or droplets.
Injectable hydrogels have recently attracted the significant attention of researchers as they offer several benefits including cytocompatibility, non-invasive administration, tunable mechanical properties, high permeability, controllable degradability, and injectability, and these hydrogels can be used as scaffolds or as carriers of therapeutic agents such as drugs, cells, and proteins. However, several essential challenges still need to be overcome to use these injectable hydrogels successfully. Two important properties for the effective application of injectable hydrogels are strength and degradation. Although researchers are now producing high-strength hydrogels, the balance of strength with degradation is crucial for hydrogels. The degradation rate of hydrogels should be well adjusted with cell adhesion and proliferation. Also, developing advanced controllable electro-sensitive hydrogels with a faster response rate for smart drug delivery is another area to explore further in depth.
Finally, we may conclude that significant developments have been made over the past few decades to improve the properties of hydrogels and these improvements need to be continued in the near future to improve, modify, and create a new paradigm of application opportunities. Therefore, the authors of the manuscript feel that a comprehensive overview of synthesis processes and understanding the strategies of tuning special functional properties of smart hydrogels are a pressing need.
Conflicts of interest
The authors have no potential conflicts of interest.
Acknowledgements
This research did not receive any specific grant from funding agencies in the public, commercial, or non-profit sectors.
References
- J. Hu, H. Meng, G. Li and S. I. Ibekwe, A review of stimuli-responsive polymers for smart textile applications, Smart Mater. Struct., 2012, 21(5), 1–23 CrossRef.
- M. Vockley, Game-changing technologies: 10 promising innovations for healthcare, Biomed. Instrum. Technol., 2017, 51(2), 96–108, DOI:10.2345/0899-8205-51.2.96.
- N. P. Murphy and K. J. Lampe, Mimicking biological phenomena in hydrogel-based biomaterials to promote dynamic cellular responses, J. Mater. Chem. B, 2015, 3(40), 7867–7880 RSC.
- S. Tang, B. M. Richardson and K. S. Anseth, Dynamic covalent hydrogels as biomaterials to mimic the viscoelasticity of soft tissues, Prog. Mater. Sci., 2020, 100738, DOI:10.1016/j.pmatsci.2020.100738.
- A. Skardal, M. Devarasetty, H. W. Kang, I. Mead, C. Bishop and T. Shupe, et al., A hydrogel bioink toolkit for mimicking native tissue biochemical and mechanical properties in bioprinted tissue constructs, Acta Biomater., 2015, 25, 24–34, DOI:10.1016/j.actbio.2015.07.030.
- S. K. Samal, M. Dash, P. Dubruel and S. Van Vlierberghe, Smart polymer hydrogels: Properties, synthesis and applications, Smart Polymers and their Applications, Elsevier Ltd, 2014, pp. 237–270 Search PubMed.
- N. N. Ferreira, L. M. B. Ferreira, V. M. O. Cardoso, F. I. Boni, A. L. R. Souza and M. P. D. Gremião, Recent advances in smart hydrogels for biomedical applications: From self-assembly to functional approaches, Eur. Polym. J., 2018, 99, 117–133 CrossRef CAS.
- J. R. McLaughlin, N. L. Abbott and C. A. Guymon, Responsive superabsorbent hydrogels via photopolymerization in lyotropic liquid crystal templates, Polymer, 2018, 142, 119–126, DOI:10.1016/j.polymer.2018.03.016.
- A. Riedinger, M. Pernia Leal, S. R. Deka, C. George, I. R. Franchini and A. Falqui, et al., “Nanohybrids” Based on pH-Responsive Hydrogels and Inorganic Nanoparticles for Drug Delivery and Sensor Applications, Nano Lett., 2011, 11(8), 3136–3141 CrossRef CAS PubMed.
- S. Dutta, P. Samanta and D. Dhara, Temperature, pH and redox responsive cellulose based hydrogels for protein delivery, Int. J. Biol. Macromol., 2016, 87, 92–100, DOI:10.1016/j.ijbiomac.2016.02.042.
- Y. Zhu, Y. Matsumura, M. Velayutham, L. M. Foley, T. K. Hitchens and W. R. Wagner, Reactive oxygen species scavenging with a biodegradable, thermally responsive hydrogel compatible with soft tissue injection, Biomaterials, 2018, 177, 98–112, DOI:10.1016/j.biomaterials.2018.05.044.
- R. Zhang, M. Tang, A. Bowyer, R. Eisenthal and J. Hubble, A novel pH- and ionic-strength-sensitive carboxy methyl dextran hydrogel, Biomaterials, 2005, 26(22), 4677–4683 CrossRef CAS PubMed . Available from: http://www.sciencedirect.com/science/article/pii/S014296120401052X.
- J. Kolosnjaj-Tabi, L. Gibot, I. Fourquaux, M. Golzio and M. P. Rols, Electric field-responsive nanoparticles and electric fields: physical, chemical, biological mechanisms and therapeutic prospects, Adv. Drug Delivery Rev., 2019, 138, 56–67, DOI:10.1016/j.addr.2018.10.017.
- Z. R. Lu, P. Kopečkovci and J. Kopeček, Antigen responsive hydrogels based on polymerizable antibody Fab′ fragment, Macromol. Biosci., 2003, 3, 296–300 CrossRef CAS.
- M. Ikeda, R. Ochi, A. Wada and I. Hamachi, Supramolecular hydrogel capsule showing prostate specific antigen-responsive function for sensing and targeting prostate cancer cells, Chem. Sci., 2010, 1(4), 491–498 RSC.
- H. Yang, H. Liu, H. Kang and W. Tan, Engineering Target-Responsive Hydrogels Based on Aptamer-Target Interactions, J. Am. Chem. Soc., 2008, 130, 6320–6321 CrossRef CAS PubMed.
- S. L. Haag and M. T. Bernards, Polyampholyte Hydrogels in Biomedical Applications, Gels, 2017, 3(4), 41 CrossRef PubMed.
- J. Ma, X. Li and Y. Bao, Advances in cellulose-based superabsorbent hydrogels, RSC Adv., 2015, 5, 59745–59757 RSC.
- M. R. Guilherme, F. A. Aouada, A. R. Fajardo, A. F. Martins, A. T. Paulino and M. F. T. Davi, et al., Superabsorbent hydrogels based on polysaccharides for application in agriculture as soil conditioner and nutrient carrier: A review, Eur. Polym. J., 2015, 365–385 CrossRef CAS.
- K. Soleimani, A. D. D. Tehrani and M. Adeli, Bioconjugated graphene oxide hydrogel as an effective adsorbent for cationic dyes removal, Ecotoxicol. Environ. Saf., 2018, 147, 34–42 CrossRef CAS PubMed.
- K. Deng, C. Bellmann, Y. Fu, M. Rohn, M. Guenther and G. Gerlach, Miniaturized force-compensated hydrogel-based pH sensors, Sens. Actuators, B, 2018, 255(3), 3495–3504 CrossRef CAS.
- Y. Cheng, K. Ren, D. Yang and J. Wei, Bilayer-type fluorescence hydrogels with intelligent response serve as temperature/pH driven soft actuators, Sens. Actuators, B, 2018, 255, 3117–3126 CrossRef CAS . Available from: http://www.sciencedirect.com/science/article/pii/S0925400517318038.
- X. Wang, H. Hu, Z. Yang, L. He, Y. Kong and B. Fei, et al., Smart hydrogel-functionalized textile system with moisture management property for skin application, Smart Mater. Struct., 2014, 23, 125027 CrossRef.
- D. Snoeck, L. Pel and N. De Belie, The water kinetics of superabsorbent polymers during cement hydration and internal curing visualized and studied by NMR, Sci. Rep., 2017, 7, 9514 CrossRef CAS PubMed.
- I. Cunha, R. Barras, P. Grey, D. Gaspar, E. Fortunato and R. Martins, et al., Reusable Cellulose-Based Hydrogel Sticker Film Applied as Gate Dielectric in Paper Electrolyte-Gated Transistors, Adv. Funct. Mater., 2017, 27, 1606755 CrossRef.
- B. Smitha, S. Sridhar and A. A. Khan, Polyelectrolyte complexes of chitosan and poly(acrylic acid) as proton exchange membranes for fuel cells, Macromolecules, 2004, 37(6), 2233–2239 CrossRef CAS.
- R. Das, S. Ghorai and S. Pal, Flocculation characteristics of polyacrylamide grafted hydroxypropyl methyl cellulose: An efficient biodegradable flocculant, Chem. Eng. J., 2013, 229, 144–152 CrossRef CAS.
- S. Chatterjee and P. Chi-leung Hui, Stimuli-Responsive Hydrogels: An Interdisciplinary Overview, Hydrogels - Smart Materials for Biomedical Applications, IntechOpen, 2019 Search PubMed.
- M. Akhtar and M. Hanif, Journal NR-SP, 2016 U. Methods of synthesis of hydrogels: A review, Saudi Pharm. J., 2016, 24(5), 554–559 CrossRef PubMed.
- W. Zhao, X. Jin, Y. Cong, Y. Liu and J. Fu, Degradable natural polymer hydrogels for articular cartilage tissue engineering, J. Chem. Technol. Biotechnol., 2013, 88, 327–339 CrossRef CAS.
- E. M. Ahmed, Hydrogel: Preparation, characterization, and applications: A review, J. Adv. Res., 2015, 6, 105–121 CrossRef CAS PubMed.
- N. A. Peppas, P. Bures, W. Leobandung and H. Ichikawa, Hydrogels in pharmaceutical formulations, Eur. J. Pharm. Biopharm., 2000, 27–46 CrossRef CAS.
- L. A. Sharpe, A. M. Daily, S. D. Horava and N. A. Peppas, Therapeutic applications of hydrogels in oral drug delivery, Expert Opin. Drug Delivery, 2014, 11, 901–915 CrossRef CAS PubMed.
- N. Kashyap, N. Kumar and M. N. V. R. Kumar, Hydrogels for pharmaceutical and biomedical applications, Crit. Rev. Ther. Drug Carrier Syst., 2005, 22, 107–149 CrossRef CAS PubMed.
- N. Sood, A. Bhardwaj, S. Mehta and A. Mehta, Stimuli-responsive hydrogels in drug delivery and tissue engineering, Drug Delivery, 2016, 23(3), 748–770 CrossRef CAS PubMed.
- M. Zelzer, S. J. Todd, A. R. Hirst, T. O. McDonald and R. V. Ulijn, Enzyme responsive materials: Design strategies and future developments, Biomater. Sci., 2013, 1, 11–39 RSC.
- D. Roy, J. N. Cambre and B. S. Sumerlin, Future perspectives and recent advances in stimuli-responsive materials, Prog. Polym. Sci., 2010, 35, 278–301 CrossRef CAS.
- J. E. Ghadiali and M. M. Stevens, Enzyme-Responsive Nanoparticle Systems, Adv. Mater., 2008, 20(22), 4359–4363 CrossRef CAS.
- R. J. Williams, R. J. Mart and R. V. Ulijn, Exploiting biocatalysis in peptide self-assembly, Biopolymers, 2010, 94(1), 107–117 CrossRef CAS PubMed.
- M. Zelzer and R. V. Ulijn, Next-generation peptide nanomaterials: Molecular networks, interfaces and supramolecular functionality, Chem. Soc. Rev., 2010, 39, 3351–3357 RSC.
- T. Miyata, N. Asami and T. Uragani, A reversibly antigen-responsive hydrogen, Nature, 1999, 399(6738), 766–768 CrossRef CAS PubMed.
- S. H. Um, J. B. Lee, N. Park, S. Y. Kwon, C. C. Umbach and D. Luo, Enzyme-catalysed assembly of DNA hydrogel, Nat. Mater., 2006, 5(10), 797–801, DOI:10.1038/nmat1741.
- D. Wang, Y. Hu, P. Liu and D. Luo, Bioresponsive DNA Hydrogels: Beyond the Conventional Stimuli Responsiveness, Acc. Chem. Res., 2017, 50(4), 733–739 CrossRef CAS PubMed.
- X. Xiong, C. Wu, C. Zhou, G. Zhu, Z. Chen and W. Tan, Responsive DNA-based hydrogels and their applications, Macromol. Rapid Commun., 2013, 34(16), 1271–1283 CrossRef CAS PubMed.
- J. Shin, A. G. Cherstvy and R. Metzler, Sensing viruses by mechanical tension of DNA in responsive hydrogels, Phys. Rev. X, 2014, 4(2), 1–13 Search PubMed.
- L. Klouda and A. G. Mikos, Thermoresponsive hydrogels in biomedical applications, Eur. J. Pharm. Biopharm., 2008, 68, 34–45 CrossRef CAS.
- S. J. Buwalda, K. W. M. Boere, P. J. Dijkstra, J. Feijen, T. Vermonden and W. E. Hennink, Hydrogels in a historical perspective: From simple networks to smart materials, J. Controlled Release, 2014, 190, 254–273 CrossRef CAS PubMed.
- M. C. Koetting, J. T. Peters, S. D. Steichen and N. A. Peppas, Stimulus-responsive hydrogels: Theory, modern advances, and applications, Mater. Sci. Eng., R, 2015, 1–49 CrossRef PubMed.
- A. M. Villalba-Rodríguez, K. Dhama and H. M. N. Iqbal, Biomaterials-based hydrogels and their drug delivery potentialities, Int. J. Pharmacol., 2017, 13(7), 864–873 Search PubMed.
- Q. Chai, Y. Jiao and X. Yu, Hydrogels for Biomedical Applications: Their Characteristics and the Mechanisms behind Them, Gels, 2017, 3(1), 6 CrossRef . Available from: https://www.ncbi.nlm.nih.gov/pubmed/30920503.
- L. J. Del Valle, A. Díaz and J. Puiggalí, Hydrogels for Biomedical Applications: Cellulose, Chitosan, and Protein/Peptide Derivatives, Gels, 2017, 3(3), 27 CrossRef PubMed . Available from: https://www.ncbi.nlm.nih.gov/pubmed/30920524.
- R. Sanchez-Orozco, B. Timoteo-Cruz, T. Torres-Blancas and F. Urena-Nunez, Valorization of Superabsorbent Polymers from Used Disposable Diapers as Soil Moisture Retainer, Int. J. Res. Granthaalayah, 2017, 5(4), 105–117, DOI:10.29121/granthaalayah.v5.i4.2017.1800.
- F. Yokoyama, I. Masada, K. Shimamura, T. Ikawa and K. Monobe, Morphology and structure of highly elastic poly(vinyl alcohol) hydrogel prepared by repeated freezing-and-melting, Colloid Polym. Sci., 1986, 264, 595–601 CrossRef CAS.
- M. Rizwan, R. Yahya, A. Hassan, M. Yar, A. D. Azzahari and V. Selvanathan, pH sensitive hydrogels in drug delivery: Brief history, properties, swelling, and release mechanism,
material selection and applications, Polymers, 2017, 9(4), 1–37, DOI:10.3390/polym9040137.
- E. Mah and R. Ghosh, Thermo-responsive hydrogels for stimuli-responsive membranes, Processes, 2013, 238–262 CrossRef.
- L. Liu, W. D. Yao, Y. F. Rao, X. Y. Lu and J. Q. Gao, pH-responsive carriers for oral drug delivery: Challenges and opportunities of current platforms, Drug Delivery, 2017, 569–581 CrossRef CAS PubMed.
- J. Liu and Y. Yin, Temperature Responsive Hydrogels: Construction and Applications Abstract Construction Method of, Polym. Sci., 2015, 1(13), 1–6 Search PubMed.
- A. G. Venkatesan, A. Baji and S. Ramakrishna, Smart functional polymers - A new route towards creating a sustainable environment, RSC Adv., 2014, 4, 53352–53364 RSC.
- K. Bauri, M. Nandi and P. De, Amino acid-derived stimuli-responsive polymers and their applications, Polym. Chem., 2018, 9(11), 1257–1287 RSC.
- I. M. El-Sherbiny, I. A. Khalil and I. H. Ali, Updates on Stimuli-Responsive Polymers: Synthesis Approaches and Features BT, in Polymer Gels: Science and Fundamentals. ed. V. K. Thakur and M. K. Thakur, Springer Singapore, Singapore, 2018, pp. 129–146 DOI:10.1007/978-981-10-6086-1_4.
- A. M. A. Hasan and M. E.-S. Abdel-Raouf, Cellulose-Based Superabsorbent Hydrogels BT, in Cellulose-Based Superabsorbent Hydrogels, ed. M. I. H. Mondal, Springer International Publishing, Cham, 2019, pp. 245–267 DOI:10.1007/978-3-319-77830-3_11.
- N. Annabi, A. Tamayol, J. A. Uquillas, M. Akbari, L. E. Bertassoni and C. Cha, et al., 25th anniversary article: Rational design and applications of hydrogels in regenerative medicine, Adv. Mater., 2014, 26(1), 85–124 CrossRef CAS PubMed.
- M. F. Akhtar, M. Hanif and N. M. Ranjha, Methods of synthesis of hydrogels … A review, Saudi Pharm. J., 2016, 24(5), 554–559, DOI:10.1016/j.jsps.2015.03.022.
- N. D. Tsihlis, J. Murar, M. R. Kapadia, S. S. Ahanchi, C. S. Oustwani and J. E. Saavedra, et al., Hydrogels: Methods of Preparation, J. Vasc. Surg., 2010, 51(5), 1248–1259 CrossRef.
- J. M. Seidel and S. M. Malmonge, Synthesis of polyHEMA hydrogels for using as biomaterials. Bulk and solution radical-initiated polymerization techniques, Mater. Res. J. Mater., 2000, 3, 79–83 CrossRef CAS.
- W. E. Hennink and C. F. van Nostrum, Novel crosslinking methods to design hydrogels, Adv. Drug Delivery Rev., 2002, 54(1), 13–36 CrossRef CAS . Available from: http://www.sciencedirect.com/science/article/pii/S0169409X0100240X.
- T. Billiet, M. Vandenhaute, J. Schelfhout, S. Van Vlierberghe and P. Dubruel, A review of trends and limitations in hydrogel-rapid prototyping for tissue engineering, Biomaterials, 2012, 33, 6020–6041 CrossRef CAS PubMed.
- A. A. Abdel-Azim, M. S. Farahat, A. M. Atta and A. A. Abdel-Fattah, Preparation and properties of two-component hydrogels based on 2-acrylamido-2-methylpropane sulphonic acid, Polym. Adv. Technol., 1998, 9(5), 282–289 CrossRef CAS.
- N. Ranganathan, R. Joseph Bensingh, M. Abdul Kader and S. K. Nayak, Synthesis and Properties of Hydrogels Prepared by Various Polymerization Reaction Systems BT, in Cellulose-Based Superabsorbent Hydrogels, ed. M. I. H. Mondal, Springer International Publishing, Cham, 2018, pp. 1–25 DOI:10.1007/978-3-319-76573-0_18-1.
- J. Li, X. Jia and L. Yin, Hydrogel: Diversity of Structures and Applications in Food Science, Food Rev. Int., 2021, 1–59, DOI:10.1080/87559129.2020.1858313.
- M. A. Macchione, C. Biglione and M. Strumia, Design, Synthesis and Architectures of Hybrid Nanomaterials for Therapy and Diagnosis Applications, Polymers, 2018, 10(5), 527 CrossRef PubMed . Available from: https://pubmed.ncbi.nlm.nih.gov/30966561.
- C. E. Carraher, Introduction to Polymer Chemistry, CRC Press, 4th edn, 2017. Available from: https://books.google.com/books?id=TL3ZDQAAQBAJ Search PubMed.
- B. M. Shin, J.-H. Kim and D. J. Chung, Synthesis of pH-responsive and adhesive super-absorbent hydrogel through bulk polymerization, Macromol. Res., 2013, 21(5), 582–587, DOI:10.1007/s13233-013-1051-4.
- D. S. Achilias and G. D. Verros, Modeling of diffusion-controlled reactions in free radical solution and bulk polymerization: Model validation by dsc experiments, J. Appl. Polym. Sci., 2010, 116(3), 1842–1856 CAS.
- M. Chanda, Introduction to Polymer Science and Chemistry: A Problem-Solving Approach, CRC Press, 2nd edn, 2013 Search PubMed.
- J. R. Ebdon Introduction to polymers (second edition) R. J. Young and P. A. Lovell Chapman and Hall, London, 1991. pp. 443, price £16.95. ISBN 0-412-30640-9 (PB); ISBN 0–412–30630–1 (HB). Polym Int [Internet]. 1992;27(2):207–8. Available from: https://doi.org/10.1002/pi.4990270217.
- N. Ranganathan, R. Joseph Bensingh, M. Abdul Kader and S. K. Nayak, Synthesis and Properties of Hydrogels Prepared by Various Polymerization Reaction Systems, in Cellulose-Based Superabsorbent Hydrogels, ed. M. I. H. Mondal, Springer International Publishing, Cham, 2018, pp. 1–25 DOI:10.1007/978-3-319-76573-0_18-1.
- M. Chanda, Introduction to Polymer Science and Chemistry: A Problem-Solving Approach, 2006 Search PubMed.
- M. Liu, R. Liang, F. Zhan, Z. Liu and A. Niu, Preparation of superabsorbent slow release nitrogen fertilizer by inverse suspension polymerization, Polym. Int., 2007, 56(6), 729–737, DOI:10.1002/pi.2196.
- C. E. Carraher, Carraher's polymer chemistry, 2018 Search PubMed.
- R. Young and P. Lovell, Introduction to polymers, 2011, Available from: https://books.google.com/books?hl=en&lr=&id=7BbSBQAAQBAJ&oi=fnd&pgPP1&dq=introduction++polymers&ots=RKLmu7R1MD&sig=d0ih56bPI0RqfLnvBqJYSiNaMPI Search PubMed.
- H. A. Essawy, M. B. M. Ghazy, F. A. El-Hai and M. F. Mohamed, Superabsorbent hydrogels via graft polymerization of acrylic acid from chitosan-cellulose hybrid and their potential in controlled release of soil nutrients, Int. J. Biol. Macromol., 2016, 89, 144–151, DOI:10.1016/j.ijbiomac.2016.04.071.
- M. W. Tibbitt, A. M. Kloxin, L. A. Sawicki and K. S. Anseth, Mechanical Properties and Degradation of Chain and Step-Polymerized Photodegradable Hydrogels, Macromolecules, 2013, 46(7), 2785–2792, DOI:10.1021/ma302522x.
- X. Shen, J. L. Shamshina, P. Berton, G. Gurau and R. D. Rogers, Hydrogels based on cellulose and chitin: Fabrication, properties, and applications, Green Chem., 2015, 18(1), 53–75 RSC.
- M. Mondal and K. Trivedy, The silk proteins, sericin and fibroin in silkworm, Bombyx mori Linn., - a review, Casp. J. Environ. Sci., 2007, 5(2), 63–76 Search PubMed.
- H. M. James and E. Guth, Theory of the elastic properties of rubber, J. Chem. Phys., 1943, 11, 455 CrossRef CAS.
- K. P. Menard and N. Menard, Dynamic Mechanical Analysis, Encyclopedia of Analytical Chemistry, 2017, pp. 1–25 (Major Reference Works). DOI:10.1002/9780470027318.a2007.pub3.
- F. A. Morrison, Understanding Rheology, Oxford Univ Press, 2001. Available from: https://global.oup.com/ushe/product/understanding-rheology-9780195141665?cc=ca&lang=en& Search PubMed.
- S. Bucatariu, G. Fundueanu, I. Prisacaru, M. Balan, I. Stoica and V. Harabagiu, et al., Synthesis and characterization of thermosensitive poly(N-isopropylacrylamide-co-hydroxyethylacrylamide) microgels as potential carriers for drug delivery, J. Polym. Res., 2014, 21(11), 1–12, DOI:10.1007/s10965-014-0580-7.
- Y. Qiu and K. Park, Environment-sensitive hydrogels for drug delivery, Adv. Drug Delivery Rev., 2001, 53(3), 321–339 CrossRef CAS PubMed.
- Y. Samchenko, Z. Ulberg and O. Korotych, Multipurpose smart hydrogel systems, Adv. Colloid Interface Sci., 2011, 168(1–2), 247–262 CrossRef CAS PubMed.
- H. Katono, K. Sanui, N. Ogata, T. Okano and Y. Sakurai, Drug release OFF behavior and deswelling kinetics of thermo-responsive IPNs composed of poly(acrylamide-co-butyl methacrylate) and poly(acrylic acid), Polym. J., 1991, 23(10), 1179–1189 CrossRef CAS.
- S. Chatterjee, P. Chi-leung Hui and C. Kan, Thermoresponsive Hydrogels and Their Biomedical Applications: Special Insight into Their Applications in Textile Based Transdermal Therapy, Polymers, 2018, 10(5), 480, DOI:10.3390/polym10050480.
- A. S. Hoffman, Hydrogels for biomedical applications, Adv. Drug Delivery Rev., 2002, 54(1), 3–12 CrossRef CAS PubMed.
- M. W. Tibbitt and K. S. Anseth, Hydrogels as extracellular matrix mimics for 3D cell culture, Biotechnol. Bioeng., 2009, 103(4), 655–663 CrossRef CAS PubMed.
- L. Klouda, Thermoresponsive hydrogels in biomedical applications A seven-year update, Eur. J. Pharm. Biopharm., 2015, 97, 338–349 CrossRef CAS.
- B. Özkahraman, I. Acar and G. Güçlü, Synthesis and characterization of poly (VCL-HEA-IA) terpolymer for drug release applications, J. Polym. Mater., 2016, 33(2), 351–363 Search PubMed.
- X.-Z. Zhang, D.-Q. Wu and C.-C. Chu, Synthesis, characterization and controlled drug release of thermosensitive IPN–PNIPAAm hydrogels, Biomaterials, 2004, 25(17), 3793–3805 CrossRef CAS PubMed . Available from: http://www.sciencedirect.com/science/article/pii/S0142961203009955.
- J. Wei, Y. Li and T. Ngai, Tailor-made microgel particles: Synthesis and characterization, Colloids Surf., A, 2016, 489, 122–127 CrossRef CAS . Available from: http://www.sciencedirect.com/science/article/pii/S0927775715303010.
- S. Dogu and W. Oppermann, Influence of observation temperature on light scattering of poly-N-isopropylacrylamide hydrogels, Soft Matter, 2012, 8(9), 2705–2713, 10.1039/C2SM07316A.
- W. H. Blackburn and L. A. Lyon, Size-controlled synthesis of monodisperse core/shell nanogels, Colloid Polym. Sci., 2008, 286(5), 563–569, DOI:10.1007/s00396-007-1805-7.
- T. Iizawa, H. Taketa, M. Maruta, T. Ishido, T. Gotoh and S. Sakohara, Synthesis of porous poly(N-isopropylacrylamide) gel beads by sedimentation polymerization and their morphology, J. Appl. Polym. Sci., 2007, 104(2), 842–850, DOI:10.1002/app.25605.
- E. Isikci Koca, G. Bozdag, G. Cayli, D. Kazan and P. Cakir Hatir, Thermoresponsive hydrogels based on renewable resources, J. Appl. Polym. Sci., 2019, 48861 Search PubMed.
- M. S. H. Akash, K. Rehman, H. Sun and S. Chen, Assessment of release kinetics, stability and polymer interaction of poloxamer 407-based thermosensitive gel of interleukin-1 receptor antagonist, Pharm. Dev. Technol., 2014, 19(3), 278–284 CrossRef CAS.
- S. J. Bae, J. M. Suh, Y. S. Sohn, Y. H. Bae, S. W. Kim and B. Jeong, Thermogelling poly(caprolactone-6-ethylene glycol-b-caprolactone) aqueous solutions, Macromolecules, 2005, 38(12), 5260–5265 CrossRef CAS.
- S. E. Stabenfeldt, A. J. García and M. C. LaPlaca, Thermoreversible laminin-functionalized hydrogel for neural tissue engineering, J. Biomed. Mater. Res., Part A, 2006, 77(4), 718–725 CrossRef PubMed.
- H. Chen and M. Fan, Novel Thermally Sensitive pH-dependent Chitosan/ Carboxymethyl Cellulose Hydrogels, J. Bioact. Compat. Polym., 2008, 23(1), 38–48 CrossRef CAS.
- N. Bhattarai, H. R. Ramay, J. Gunn, F. A. Matsen and M. Zhang, PEG-grafted chitosan as an injectable thermosensitive hydrogel for sustained protein release, J. Controlled Release, 2005, 103(3), 609–624 CrossRef CAS PubMed.
- L. L. Y. Chiu and M. Radisic, Controlled release of thymosin β4 using collagen-chitosan composite hydrogels promotes epicardial cell migration and angiogenesis, J. Controlled Release, 2011, 155(3), 376–385 CrossRef CAS PubMed.
- F. P. W. Melchels, M. A. N. Domingos, T. J. Klein, J. Malda, P. J. Bartolo and D. W. Hutmacher, Additive manufacturing of tissues and organs, Prog. Polym. Sci., 2012, 37, 1079–1104 CrossRef CAS.
- R. Suntornnond, J. An, W. Y. Yeong and C. K. Chua, Biodegradable Polymeric Films and Membranes Processing and Forming for Tissue Engineering, Macromol. Mater. Eng., 2015, 300(9), 858–877 CrossRef CAS.
- J. An, J. E. M. Teoh, R. Suntornnond and C. K. Chua, Design and 3D Printing of Scaffolds and Tissues, Engineering, 2015, 1(2), 261–268 CrossRef.
- S. V. Murphy, A. Skardal and A. Atala, Evaluation of hydrogels for bio-printing applications, J. Biomed. Mater. Res., Part A, 2013, 101(1), 272–284 CrossRef.
- J. Malda, J. Visser, F. P. Melchels, T. Jüngst, W. E. Hennink and W. J. A. Dhert, et al., 25th Anniversary Article: Engineering Hydrogels for Biofabrication, Adv. Mater., 2013, 25(36), 5011–5028 CrossRef CAS PubMed.
- R. Suntornnond, J. An and C. K. Chua, Bioprinting of Thermoresponsive Hydrogels for Next Generation Tissue Engineering: A Review, Macromol. Mater. Eng., 2017, 302(1), 1600266 CrossRef.
- J. Zaragoza, B. Nasim, V. O’brien, A. Chang, M. Blanco and A. Zabalegui, et al., Experimental Investigation of Mechanical and Thermal Properties of Silica Nanoparticle-Reinforced Poly(acrylamide) Nanocomposite Hydrogels, PLoS One, 2015, 10(8), e0136293 CrossRef PubMed.
- L.-Y. Chu, R. Xie, X.-J. Ju and W. Wang, Smart Hydrogel Functional Materials, 2013 Search PubMed.
- J. Sun, Y. Guo, R. Xing, T. Jiao, Q. Zou and X. Yan, Synergistic in vivo photodynamic and photothermal antitumor therapy based on collagen-gold hybrid hydrogels with inclusion of photosensitive drugs, Colloids Surf., A, 2017, 514(5), 155–160 CrossRef CAS.
- X. Wang, C. Wang, Q. Zhang and Y. Cheng, Near infrared light-responsive and injectable supramolecular hydrogels for on-demand drug delivery, Chem. Commun., 2016, 52(5), 978–981 RSC.
- N. Roshan and M. Rahul, Photosensitive hydrogels for advanced drug delivery, Int. J. Rev. Artic. Pharm. Innov., 2013, 3(2), 11 Search PubMed.
- R. C. Luo, Z. H. Lim, W. Li, P. Shi and C. H. Chen, Near-infrared light triggerable deformation-free polysaccharide double network hydrogels, Chem. Commun., 2014, 50(53), 7052–7055 RSC.
- L. Li, J. M. Scheiger and P. A. Levkin, Design and Applications of Photoresponsive Hydrogels, Adv. Mater., 2019, 31(26), 1–17, DOI:10.1002/adma.201807333.
- J. Ter Schiphorst, S. Coleman, J. E. Stumpel, A. Ben Azouz, D. Diamond and A. P. H. J. Schenning, Molecular Design of Light-Responsive Hydrogels, for in Situ Generation of Fast and Reversible Valves for Microfluidic Applications, Chem. Mater., 2015, 27(17), 5925–5931 CrossRef CAS.
- J. H. Kim and T. R. Lee, Thermo- and pH-responsive hydrogel-coated gold nanoparticles, Chem. Mater., 2004, 16(19), 3647–3651 CrossRef CAS.
- Y. L. Zhao and J. Fraser Stoddart, Azobenzene-based light-responsive hydrogel system, Langmuir, 2009, 25(15), 8442–8446 CrossRef CAS PubMed.
- C. W. Lo, D. Zhu and H. Jiang, An infrared-light responsive graphene-oxide incorporated poly(N-isopropylacrylamide) hydrogel nanocomposite, Soft Matter, 2011, 7(12), 5604–5609 RSC.
- L. Han, Y. Zhang, X. Lu, K. Wang, Z. Wang and H. Zhang, Polydopamine Nanoparticles Modulating Stimuli-Responsive PNIPAM Hydrogels with Cell/Tissue Adhesiveness, ACS Appl. Mater. Interfaces, 2016, 8(42), 29088–29100 CrossRef CAS PubMed.
- Y. Wu, K. Wang, S. Huang, C. Yang and M. Wang, Near-Infrared Light-Responsive Semiconductor Polymer Composite Hydrogels: Spatial/Temporal-Controlled Release via a Photothermal “sponge” Effect, ACS Appl. Mater. Interfaces, 2017, 9(15), 13602–13610 CrossRef CAS PubMed.
- A. Guiseppi-Elie, Electroconductive hydrogels: Synthesis, characterization and biomedical applications, Biomaterials, 2010, 31(10), 2701–2716, DOI:10.1016/j.biomaterials.2009.12.052.
- J. P. Gong, T. Nitta and Y. Osada, Electrokinetic modeling of the contractile phenomena of polyelectrolyte gels. one-dimensional capillary model, J. Phys. Chem., 1994, 98(38), 9583–9587 CrossRef CAS.
- M. Zrínyi, J. Fehér and G. Filipcsei, Novel gel actuator containing TiO2 particles operated under static electric field, Macromolecules, 2000, 33(16), 5752–5753 CrossRef.
- Y. K. Yew, T. Y. Ng, H. Li and K. Y. Lam, Analysis of pH and electrically controlled swelling of hydrogel-based micro-sensors/actuators, Biomed. Microdevices, 2007, 9(4), 487–499 CrossRef CAS.
- Y. Osada, H. Okuzaki and H. Hori, A polymer gel with electrically driven motility, Nature, 1992, 355(6357), 242–244 CrossRef CAS.
- W. Zhang, P. Feng, J. Chen, Z. Sun and B. Zhao, Electrically conductive hydrogels for flexible energy storage systems, Prog. Polym. Sci., 2019, 88, 220–240, DOI:10.1016/j.progpolymsci.2018.09.001.
- Y. Zhou, C. Wan, Y. Yang, H. Yang, S. Wang and Z. Dai, et al., Highly Stretchable, Elastic, and Ionic Conductive Hydrogel for Artificial Soft Electronics, Adv. Funct. Mater., 2019, 29(1), 1–8 CrossRef.
- Z. Wang, H. Zhou, J. Lai, B. Yan, H. Liu and X. Jin, et al., Extremely stretchable and electrically conductive hydrogels with dually synergistic networks for wearable strain sensors, J. Mater. Chem. C, 2018, 6(34), 9200–9207 RSC.
- H. A. Alhadrami, Biosensors: Classifications, medical applications, and future prospective, Biotechnol. Appl. Biochem., 2018, 65(3), 497–508 CrossRef CAS.
- M. X. Chu, K. Miyajima, D. Takahashi, T. Arakawa, K. Sano and S. Sawada, et al., Soft contact lens biosensor for in situ monitoring of tear glucose as non-invasive blood sugar assessment, Talanta, 2011, 83(3), 960–965 CrossRef CAS PubMed . Available from: http://www.sciencedirect.com/science/article/pii/S0039914010008519.
- P. Mehrotra, Biosensors and their applications – A review, J. Oral. Biol. Craniofac. Res., 2016, 6(2), 153–159 CrossRef PubMed . Available from: http://www.sciencedirect.com/science/article/pii/S2212426815001323.
- A. Kaushik and M. A. Mujawar, Point of Care Sensing Devices: Better Care for Everyone, Sensors, 2018, 18(12), 4303 CrossRef . Available from: https://pubmed.ncbi.nlm.nih.gov/30563249.
- G. Kaklamani, D. Kazaryan, J. Bowen, F. Iacovella, S. H. Anastasiadis and G. Deligeorgis, On the electrical conductivity of alginate hydrogels, Regener. Biomater., 2018, 5(5), 293–301 CrossRef CAS.
- E. C. G. Frachini and D. F. S. Petri, Magneto-responsive hydrogels: Preparation, characterization, biotechnological and environmental applications, J. Braz. Chem. Soc., 2019, 30(10), 2010–2028 CAS.
- J. Zhang, Q. Huang and J. Du, Recent advances in magnetic hydrogels, Polym. Int., 2016, 65(12), 1365–1372 CrossRef CAS.
- K. Hu, J. Sun, Z. Guo, P. Wang, Q. Chen and M. Ma, et al., A novel magnetic hydrogel with aligned magnetic colloidal assemblies showing controllable enhancement of magnetothermal effect in the presence of alternating magnetic field, Adv. Mater., 2015, 27(15), 2507–2514 CrossRef CAS PubMed.
- O. Ozay, S. Ekici, Y. Baran, N. Aktas and N. Sahiner, Removal of toxic metal ions with magnetic hydrogels, Water Res., 2009, 43(17), 4403–4411 CrossRef CAS PubMed.
- M. Häring, J. Schiller, J. Mayr, S. Grijalvo, R. Eritja and D. Díaz, Magnetic Gel Composites for Hyperthermia Cancer Therapy, Gels, 2015, 1(2), 135–161 CrossRef PubMed.
- Y.-Y. Liang, L.-M. Zhang, W. Jiang and W. Li, Embedding Magnetic Nanoparticles into Polysaccharide-Based Hydrogels for Magnetically Assisted Bioseparation, ChemPhysChem, 2007, 8(16), 2367–2372 CrossRef CAS PubMed.
- G. Beaune and C. Ménager, In situ precipitation of magnetic fluid encapsulated in giant liposomes, J. Colloid Interface Sci., 2010, 343(1), 396–399 CrossRef CAS.
- M. K. Shin, S. I. Kim, S. J. Kim, S. Y. Park, Y. H. Hyun and Y. P. Lee, et al., Controlled magnetic nanofiber hydrogels by clustering ferritin, Langmuir, 2008, 24(21), 12107–12111 CrossRef CAS PubMed.
- G. Giani, S. Fedi and R. Barbucci, Hybrid Magnetic Hydrogel: A Potential System for Controlled Drug Delivery by Means of Alternating Magnetic Fields, Polymers, 2012, 4(2), 1157–1169 CrossRef.
- S. Kango, S. Kalia, A. Celli, J. Njuguna, Y. Habibi and R. Kumar, Surface modification of inorganic nanoparticles for development of organic-inorganic nanocomposites - A review, Prog. Polym. Sci., 2013, 38, 1232–1261 CrossRef CAS.
- M. F. Horst, M. D. Ninago and V. Lassalle, Magnetically responsive gels based on crosslinked gelatin: An overview on the synthesis, properties, and their potential in water remediation, Int. J. Polym. Mater. Polym. Biomater., 2018, 67(11), 647–659 CrossRef CAS.
- T. Glaser, V. B. Bueno, D. R. Cornejo, D. F. S. Petri and H. Ulrich, Neuronal adhesion, proliferation and differentiation of embryonic stem cells on hybrid scaffolds made of xanthan and magnetite nanoparticles, Biomed. Mater., 2015, 10(4), 045002 CrossRef PubMed.
- P. S. Castro, M. Bertotti, A. F. Naves, L. H. Catalani, D. R. Cornejo and G. D. Bloisi, et al., Hybrid magnetic scaffolds: The role of scaffolds charge on the cell proliferation and Ca2+ ions permeation, Colloids Surf., B, 2017, 156, 388–396 CrossRef CAS PubMed.
- R. Muzzalupo, L. Tavano, C. O. Rossi, N. Picci and G. A. Ranieri, Novel pH sensitive ferrogels as new approach in cancer treatment: Effect of the magnetic field on swelling and drug delivery, Colloids Surf., B, 2015, 134, 273–278 CrossRef CAS PubMed.
- J. I. Kim, C. J. Chun, B. Kim, J. M. Hong, J. K. Cho and S. H. Lee, et al., Thermosensitive/magnetic poly(organophosphazene) hydrogel as a long-term magnetic resonance contrast platform, Biomaterials, 2012, 33(1), 218–224 CrossRef CAS PubMed.
- Y. Li, G. Huang, X. Zhang, B. Li, Y. Chen and T. Lu, et al., Magnetic Hydrogels and Their Potential Biomedical Applications, Adv. Funct. Mater., 2013, 23(6), 660–672 CrossRef CAS.
- Y. Xia, T. D. Nguyen, M. Yang, B. Lee, A. Santos and P. Podsiadlo, et al., Self-assembly of self-limiting monodisperse supraparticles from polydisperse nanoparticles, Nat. Nanotechnol., 2011, 6(9), 580–587 CrossRef CAS.
- S. Laurent, S. Dutz, U. O. Häfeli and M. Mahmoudi, Magnetic fluid hyperthermia: Focus on superparamagnetic iron oxide nanoparticles, Adv. Colloid Interface Sci., 2011, 166, 8–23 CrossRef CAS PubMed.
- C. S. S. R. Kumar and F. Mohammad, Magnetic nanomaterials for hyperthermia-based therapy and controlled drug delivery, Adv. Drug Delivery Rev., 2011, 63, 789–808 CrossRef CAS PubMed.
- H. Liu, C. Wang, Q. Gao, X. Liu and Z. Tong, Magnetic
hydrogels with supracolloidal structures prepared by suspension polymerization stabilized by Fe2O3 nanoparticles, Acta Biomater., 2010, 6(1), 275–281, DOI:10.1016/j.actbio.2009.06.018.
- D. Ogomi, T. Serizawa and M. Akashi, Controlled release based on the dissolution of a calcium carbonate layer deposited on hydrogels, J. Controlled Release, 2005, 103(2), 315–323 CrossRef CAS.
- D. S. Couto, Z. Hong and J. F. Mano, Development of bioactive and biodegradable chitosan-based injectable systems containing bioactive glass nanoparticles, Acta Biomater., 2009, 5(1), 115–123 CrossRef CAS.
- C. Wang, H. Liu, Q. Gao, X. Liu and Z. Tong, Alginate-calcium carbonate porous microparticle hybrid hydrogels with versatile drug loading capabilities and variable mechanical strengths, Carbohydr. Polym., 2008, 71(3), 476–480 CrossRef CAS.
- N. Häntzschel, F. Zhang, F. Eckert, A. Pich and M. A. Winnik, Poly(N-vinylcaprolactam-co-glycidyl methacrylate) aqueous microgels labeled with fluorescent LaF3:Eu nanoparticles, Langmuir, 2007, 23(21), 10793–10800 CrossRef.
- A. K. Nayak and B. Das, Introduction to polymeric gels, Polymeric Gels, Elsevier, 2018, pp. 3–27 Search PubMed.
- B. Mishra, M. Upadhyay, A. S. Reddy, B. Vasant and M. Muthu, Hydrogels: An introduction to a controlled drug delivery device, synthesis and application in drug delivery and tissue engineering, Austin J. Biomed. Eng., 2017, 4(1), 1–13 Search PubMed.
- M. O. Lamminen, H. W. Walker and L. K. Weavers, Mechanisms and factors influencing the ultrasonic cleaning of particle-fouled ceramic membranes, J. Membr. Sci., 2004, 237(1–2), 213–223 CrossRef CAS.
- L. V. Wang and S. Hu, Photoacoustic tomography: In vivo imaging from organelles to organs, Science, 2012, 335, 1458–1462 CrossRef CAS.
- N. Huebsch, C. J. Kearney, X. Zhao, J. Kim, C. A. Cezar and Z. Suo, et al., Ultrasound-triggered disruption and self-healing of reversibly cross-linked hydrogels for drug delivery and enhanced chemotherapy, Proc. Natl. Acad. Sci. U. S. A., 2014, 111(27), 9762–9767 CrossRef CAS.
- Y. Zhang, R. Jiang, A. Fang, Y. Zhao, T. Wu and X. Cao, et al., A highly transparent, elastic, injectable sericin hydrogel induced by ultrasound, Polym. Test., 2019, 77, 105890 CrossRef CAS.
- K. P. Mercado, M. Helguera, D. C. Hocking and D. Dalecki, Noninvasive Quantitative Imaging of Collagen Microstructure in Three-Dimensional Hydrogels Using High-Frequency Ultrasound, Tissue Eng., Part C, 2015, 21(7), 671–682 CrossRef CAS.
- L. Yan, L. Wang, S. Gao, C. Liu, Z. Zhang and A. Ma, et al., Celery cellulose hydrogel as carriers for controlled release of short-chain fatty acid by ultrasound, Food Chem., 2019, 309, 125717 CrossRef PubMed.
- W. C. Huang, C. J. Bettinger, K. Rhee and C. J. Bettinger, Ultrasound-Mediated Self-Healing Hydrogels Based on Tunable Metal-Organic Bonding, Biomacromolecules, 2017, 18(4), 1162–1171 CrossRef CAS.
- Z. Sun, C. Song, C. Wang, Y. Hu and J. Wu, Hydrogel-Based Controlled Drug Delivery for Cancer Treatment: A Review, Mol. Pharmaceutics, 2020, 17(2), 373–391 CAS.
- Y. Z. Zhao, L. N. Du, C. T. Lu, Y. G. Jin and S. P. Ge, Potential and problems in ultrasound-responsive drug delivery systems, Int. J. Nanomed., 2013, 8, 1621–1633 Search PubMed.
- Y. Z. Zhang, K. H. Lee, D. H. Anjum, R. Sougrat, Q. Jiang and H. Kim, et al., MXenes stretch hydrogel sensor performance to new limits, Sci. Adv., 2018, 4(6), eaat0098 CrossRef PubMed.
- C. S. Kwok, P. D. Mourad, L. A. Crum and B. D. Ratner, Self-assembled molecular structures as ultrasonically-responsive barrier membranes for pulsatile drug delivery, J. Biomed. Mater. Res., 2001, 57(2), 151–164 CrossRef CAS PubMed.
- C. S. Kwok, P. D. Mourad, L. A. Crum and B. D. Ratner, Surface modification of polymers with self-assembled molecular structures: Multitechnique surface characterization, Biomacromolecules, 2000, 1(1), 139–148 CrossRef CAS PubMed.
- Y. Si, L. Wang, X. Wang, N. Tang, J. Yu and B. Ding, Ultrahigh-Water-Content, Superelastic, and Shape-Memory Nanofiber-Assembled Hydrogels Exhibiting Pressure-Responsive Conductivity, Adv. Mater., 2017, 29, 1700339 CrossRef PubMed.
- Z. Shao and F. Vollrath, Surprising strength of silkworm silk, Nature, 2002, 418(6899), 741 CrossRef CAS.
- E. Steven, W. R. Saleh, V. Lebedev, S. F. A. Acquah, V. Laukhin and R. G. Alamo, et al., Carbon nanotubes on a spider silk scaffold, Nat. Commun., 2013, 4(1), 1–8 Search PubMed.
- J. Visser, F. P. W. Melchels, J. E. Jeon, E. M. Van Bussel, L. S. Kimpton and H. M. Byrne, et al., Reinforcement of hydrogels using three-dimensionally printed microfibres, Nat. Commun., 2015, 6(1), 1–10 Search PubMed.
- T. A. Schaedler, A. J. Jacobsen, A. Torrents, A. E. Sorensen, J. Lian and J. R. Greer, et al., Ultralight metallic microlattices, Science, 2011, 334(6058), 962–965 CrossRef CAS PubMed.
- T. Hiratani, O. Kose, W. Y. Hamad and M. J. Maclachlan, Stable and sensitive stimuli-responsive anisotropic hydrogels for sensing ionic strength and pressure, Mater. Horiz., 2018, 5(6), 1076–1081 RSC.
- X. Y. Lin, Z. J. Wang, P. Pan, Z. L. Wu and Q. Zheng, Monodomain hydrogels prepared by shear-induced orientation and subsequent gelation, RSC Adv., 2016, 6(97), 95239–95245 RSC.
- X. Wang, B. Ding, G. Sun, M. Wang and J. Yu, Electro-spinning/netting: A strategy for the fabrication of three-dimensional polymer nano-fiber/nets, Prog. Mater. Sci., 2013, 58, 1173–1243 CrossRef CAS.
- A. R. Hibbins, P. Kumar, Y. E. Choonara, P. P. D. Kondiah, T. Marimuthu and L. C. du Toit, et al., Design of a versatile pH-responsive hydrogel for potential oral delivery of gastric-sensitive bioactives, Polymers, 2017, 9(10), 1–19, DOI:10.3390/polym9060189.
- G. Kocak, C. Tuncer and V. Bütün, PH-Responsive polymers, Polym. Chem., 2017, 8(1), 144–176 RSC.
- S. Chatterjee and P. C. Hui, Delivery and Textile Application, Molecules, 2019, 24(14), 2547 CrossRef CAS.
- N. Ninan, A. Forget, V. P. Shastri, N. H. Voelcker and A. Blencowe, Antibacterial and Anti-Inflammatory pH-Responsive Tannic Acid-Carboxylated Agarose Composite Hydrogels for Wound Healing, ACS Appl. Mater. Interfaces, 2016, 8(42), 28511–28521 CrossRef CAS.
- Y. Dong, W. Wang, O. Veiseh, E. A. Appel, K. Xue and M. J. Webber, et al., Injectable and Glucose-Responsive Hydrogels Based on Boronic Acid-Glucose Complexation, Langmuir, 2016, 32(34), 8743–8747 CrossRef CAS.
- D. Suhag, R. Bhatia, S. Das, A. Shakeel, A. Ghosh and A. Singh, et al., Physically cross-linked pH-responsive hydrogels with tunable formulations for controlled drug delivery, RSC Adv., 2015, 5(66), 53963–53972 RSC.
- A. Hebeish, S. Farag, S. Sharaf and T. I. Shaheen, Radically new cellulose nanocomposite hydrogels: Temperature and pH responsive characters, Int. J. Biol. Macromol., 2015, 81, 356–361, DOI:10.1016/j.ijbiomac.2015.08.014.
- A. El-Hag Ali Said, Radiation synthesis of interpolymer polyelectrolyte complex and its application as a carrier for colon-specific drug delivery system, Biomaterials, 2005, 26(15), 2733–2739 CrossRef CAS PubMed.
- S. K. Gunasekar, J. S. Haghpanah and J. K. Montclare, Review Assembly of bioinspired helical protein fibers, Polym. Adv. Technol., 2008, 454–468 CrossRef CAS.
- L. Li, J. Gu, J. Zhang, Z. Xie, Y. Lu and L. Shen, et al., Injectable and biodegradable pH-responsive hydrogels for localized and sustained treatment of human fibrosarcoma, ACS Appl. Mater. Interfaces, 2015, 7(15), 8033–8040 CrossRef CAS PubMed.
- Y. Zhang, L. Tao, S. Li and Y. Wei, Synthesis of multiresponsive and dynamic chitosan-based hydrogels for controlled release of bioactive molecules, Biomacromolecules, 2011, 12(8), 2894–2901 CrossRef CAS PubMed.
- J. Huang and X. Jiang, Injectable and Degradable pH-Responsive Hydrogels via Spontaneous Amino-Yne Click Reaction, ACS Appl. Mater. Interfaces, 2018, 10(1), 361–370 CrossRef CAS.
- K. L. Shantha and D. R. K. Harding, Preparation and in-vitro evaluation of poly[N-vinyl-2-pyrrolidone-polyethylene glycol diacrylate]-chitosan interpolymeric pH-responsive hydrogels for oral drug delivery, Int. J. Pharm., 2000, 207(1–2), 65–70 CrossRef CAS.
- X. J. Loh and O. A. Scherman, in Polymeric and Self Assembled Hydrogels, ed. X. J. Loh and O. A. Scherman, Royal Society of Chemistry, 2012, (Monographs in Supramolecular Chemistry) Search PubMed.
- B. D. Fairbanks, M. P. Schwartz, A. E. Halevi, C. R. Nuttelman, C. N. Bowman and K. S. Anseth, A versatile synthetic extracellular matrix mimic via thiol-norbornene photopolymerization, Adv. Mater., 2009, 21(48), 5005–5010 CrossRef CAS PubMed.
- S. V. Wegner, F. C. Schenk, S. Witzel, F. Bialas and J. P. Spatz, Cobalt Cross-Linked Redox-Responsive PEG Hydrogels: From Viscoelastic Liquids to Elastic Solids, Macromolecules, 2016, 49(11), 4229–4235 CrossRef CAS.
- L. Liu, Y. Pei, C. He and L. Chen, Synthesis of novel thermo- and redox-sensitive polypeptide hydrogels, Polym. Int., 2017, 66(5), 712–718, DOI:10.1002/pi.5313.
- R. Cheng, F. Feng, F. Meng, C. Deng, J. Feijen and Z. Zhong, Glutathione-responsive nano-vehicles as a promising platform for targeted intracellular drug and gene delivery, J. Controlled Release, 2011, 152(1), 2–12, DOI:10.1016/j.jconrel.2011.01.030.
- F. Meng, W. E. Hennink and Z. Zhong, Reduction-sensitive polymers and bioconjugates for biomedical applications, Biomaterials, 2009, 30(12), 2180–2198 CrossRef CAS.
- F. Schafer and G. Buettner, Redox Environment of the Cell, Free Radical Biol. Med., 2001, 30(11), 1191–1212 CrossRef CAS PubMed.
- C. Legros, M. C. De Pauw-Gillet, K. C. Tam, S. Lecommmandoux and D. Taton, PH and redox responsive hydrogels and nanogels made from poly(2-ethyl-2-oxazoline), Polym. Chem., 2013, 4, 4801–4808 RSC.
- M. Nakahata, Y. Takashima, H. Yamaguchi and A. Harada, Redox-responsive self-healing materials formed from host–guest polymers, Nat. Commun., 2011, 2(1), 1–6, DOI:10.1038/ncomms1521.
- T. Kajisa and T. Sakata, Glucose-responsive hydrogel electrode for biocompatible glucose transistor, Sci. Technol. Adv. Mater., 2017, 18(1), 26–33 CrossRef CAS.
- K. M. Bratlie, R. L. York, M. A. Invernale, R. L. Langer and D. G. Anderson, Materials for diabetes therapeutics, Adv. Healthcare Mater., 2012, 1(3), 267–284 CrossRef CAS.
- R. Ma and L. Shi, Phenylboronic acid-based glucose-responsive polymeric nanoparticles: Synthesis and applications in drug delivery, Polym. Chem., 2014, 5, 1503–1518 RSC.
- T. Yang, R. Ji, X. X. Deng, F. S. Du and Z. C. Li, Glucose-responsive hydrogels based on dynamic covalent chemistry and inclusion complexation, Soft Matter, 2014, 10(15), 2671–2678 RSC.
- G. Lin, S. Chang, H. Hao, P. Tathireddy, M. Orthner and J. Magda, et al., Osmotic swelling pressure response of smart hydrogels suitable for chronically implantable glucose sensors, Sens. Actuators, B, 2010, 144(1), 332–336 CrossRef CAS.
- S. Tierney, S. Volden and B. T. Stokke, Glucose sensors based on a responsive gel incorporated as a Fabry-Perot cavity on a fiber-optic readout platform, Biosens. Bioelectron., 2009, 24(7), 2034–2039 CrossRef CAS PubMed.
- O. Garcia-Valdez, T. Brescacin, J. Arredondo, J. Bouchard, P. G. Jessop and P. Champagne, et al., Grafting CO2-responsive polymers from cellulose nanocrystals: Via nitroxide-mediated polymerisation, Polym. Chem., 2017, 8(28), 4124–4131 RSC.
- W. Zheng, G. Yang, N. Shao, L. J. Chen, B. Ou and S. T. Jiang, et al., CO2 stimuli-responsive, injectable block copolymer hydrogels cross-linked by discrete organoplatinum(II) metallacycles via stepwise post-assembly polymerization, J. Am. Chem. Soc., 2017, 139(39), 13811–13820 CrossRef CAS PubMed.
- Y. Wang, Y. Gu, E. G. Keeler, J. V. Park, R. G. Griffin and J. A. Johnson, Star PolyMOCs with Diverse Structures, Dynamics, and Functions by Three-Component Assembly, Angew. Chem., Int. Ed., 2017, 56(1), 188–192 CrossRef CAS PubMed.
- J. A. Foster, R. M. Parker, A. M. Belenguer, N. Kishi, S. Sutton and C. Abell, et al., Differentially addressable cavities within metal-organic cage-cross-linked polymeric hydrogels, J. Am. Chem. Soc., 2015, 137(30), 9722–9729 CrossRef CAS PubMed.
- K. Kawamoto, S. C. Grindy, J. Liu, N. Holten-Andersen and J. A. Johnson, Dual role for 1,2,4,5-tetrazines in polymer networks: Combining Diels-Alder reactions and metal coordination to generate functional supramolecular gels, ACS Macro Lett., 2015, 4(4), 458–461 CrossRef CAS.
- Y. Wang, M. Zhong, J. V. Park, A. V. Zhukhovitskiy, W. Shi and J. A. Johnson, Block Co-PolyMOCs by Stepwise Self-Assembly, J. Am. Chem. Soc., 2016, 138(33), 10708–10715 CrossRef CAS.
- A. V. Zhukhovitskiy, M. Zhong, E. G. Keeler, V. K. Michaelis, J. E. P. Sun and M. J. A. Hore, et al., Highly branched and loop-rich gels via formation of metal-organic cages linked by polymers, Nat. Chem., 2016, 8(1), 33–41 CrossRef CAS PubMed.
- X. Yan, S. Li, T. R. Cook, X. Ji, Y. Yao and J. B. Pollock, et al., Hierarchical self-assembly: Well-defined supramolecular nanostructures and metallohydrogels via amphiphilic discrete organoplatinum(II) metallacycles, J. Am. Chem. Soc., 2013, 135(38), 14036–14039 CrossRef CAS.
- W. Zheng, L. J. Chen, G. Yang, B. Sun, X. Wang and B. Jiang, et al., Construction of Smart Supramolecular Polymeric Hydrogels Cross-linked by Discrete Organoplatinum(II) Metallacycles via Post-Assembly Polymerization, J. Am. Chem. Soc., 2016, 138(14), 4927–4937 CrossRef CAS PubMed.
- Z. Y. Li, Y. Zhang, C. W. Zhang, L. J. Chen, C. Wang and H. Tan, et al., Cross-linked supramolecular polymer gels constructed from discrete multi-pillar[5]arene metallacycles and their multiple stimuli-responsive behavior, J. Am. Chem. Soc., 2014, 136(24), 8577–8589 CrossRef CAS.
- X. Yan, S. Li, J. B. Pollock, T. R. Cook, J. Chen and Y. Zhang, et al., Supramolecular polymers with tunable topologies via hierarchical coordination-driven self-assembly and hydrogen bonding interfaces, Proc. Natl. Acad. Sci. U. S. A., 2013, 110(39), 15585–15590 CrossRef CAS.
- G. Yu, Y. Lu, X. Liu, W. J. Wang, Q. Yang and H. Xing, et al., Polyethylenimine-assisted extraction of α-tocopherol from tocopherol homologues and CO2-triggered fast recovery of the extractant, Ind. Eng. Chem. Res., 2014, 53(41), 16025–16032 CrossRef CAS.
- Y. Ma and L. Y. L. Yung, Detection of dissolved CO2 based on the aggregation of gold nanoparticles, Anal. Chem., 2014, 86(5), 2429–2435 CrossRef CAS PubMed.
- Q. Yan and Y. Zhao, CO2-stimulated diversiform deformations of polymer assemblies, J. Am. Chem. Soc., 2013, 135(44), 16300–16303 CrossRef CAS PubMed.
- W. Lu, J. P. Sculley, D. Yuan, R. Krishna, Z. Wei and H.-C. Zhou, Polyamine-Tethered Porous Polymer Networks for Carbon Dioxide Capture from Flue Gas, Angew. Chem., Int. Ed., 2012, 51(30), 7480–7484 CrossRef CAS PubMed.
- Q. Yan, R. Zhou, C. Fu, H. Zhang, Y. Yin and J. Yuan, CO2-Responsive Polymeric Vesicles that Breathe, Angew. Chem., Int. Ed., 2011, 50(21), 4923–4927 CrossRef CAS PubMed.
- B. Ochiai, K. Yokota, A. Fujii, D. Nagai and T. Endo, Reversible trap - Release of CO2 by polymers bearing DBU and DBN moieties, Macromolecules, 2008, 41(4), 1229–1236 CrossRef CAS.
- H. J. Zhang, Y. N. Zhang, B. Yang and L. G. Wang, Swelling or deswelling? Composition-dependent CO2-responsive swelling behaviors of poly(acrylamide-co-2-dimethylaminoethyl methacrylate) hydrogels, Colloid Polym. Sci., 2018, 296(2), 393–403 CrossRef CAS.
- L. Wang, J. Shang, S. Liu, L. Liu, S. Zhang and Y. Deng, Environmentally benign and effective syntheses of N-substituted carbamates via alcoholysis of disubstituted ureas over TiO2/SiO2 catalyst, Pure Appl. Chem., 2011, 84(3), 461–471 Search PubMed.
- L. Wang, H. Li, S. Xin, P. He, Y. Cao and F. Li, et al., Highly efficient synthesis of diethyl carbonate via one-pot reaction from carbon dioxide, epoxides and ethanol over KI-based binary catalyst system, Appl. Catal., A, 2014, 471, 19–27 CrossRef CAS.
- D. Nagai, A. Suzuki, Y. Maki and H. Takeno, Reversible chain association/dissociation via a CO2 responsive crosslinking/decrosslinking system, Chem. Commun., 2011, 47(31), 8856–8858 RSC.
- J. Cheng, G. Shan and P. Pan, Triple Stimuli-Responsive N-Isopropylacrylamide Copolymer toward Metal Ion Recognition and Adsorption via a Thermally Induced Sol-Gel Transition, Ind. Eng. Chem. Res., 2017, 56(5), 1223–1232 CrossRef CAS.
- X. Q. Wang, R. Hong, C. F. Wang, P. F. Tan, W. Q. Ji and S. Chen, Ultrafast mechano-responsive photonic hydrogel towards multicolor displays via the pressure sensation, Mater. Lett., 2017, 189, 321–324 CrossRef CAS.
- B. W. Liu, H. Zhou, S. T. Zhou, H. J. Zhang, A. C. Feng and C. M. Jian, et al., Synthesis and self-assembly of CO2-temperature dual stimuli-responsive triblock copolymers, Macromolecules, 2014, 47(9), 2938–2946 CrossRef CAS.
- J. Hu, G. Zhang and S. Liu, Enzyme-responsive polymeric assemblies, nanoparticles and hydrogels, Chem. Soc. Rev., 2012, 41(18), 5933–5949 RSC.
- M. E. Hahn and N. C. Gianneschi, Enzyme-directed assembly and manipulation of organic nanomaterials, Chem. Commun., 2011, 47(43), 11814–11821 RSC.
- R. Chandrawati, Enzyme-responsive polymer hydrogels for therapeutic delivery, Exp. Biol. Med., 2016, 241(9), 972–979, DOI:10.1177/1535370216647186.
- Y. M. Abul-Haija and R. V. Ulijn, Enzyme-responsive hydrogels for biomedical applications, Soft Matter, 2014,(2), 112–134 CAS.
- N. C. Inestrosa, A. Alvarez, C. A. Pérez, R. D. Moreno, M. Vicente and C. Linker, et al., Acetylcholinesterase accelerates assembly of amyloid-β-peptides into Alzheimer's fibrils: Possible role of the peripheral site of the enzyme, Neuron, 1996, 16(4), 881–891 CrossRef CAS PubMed.
- V. Turk, J. Kos and B. Turk, Cysteine cathepsins (proteases) - On the main stage of cancer?, Cancer Cell, 2004, 5, 409–410 CrossRef CAS.
- O. Wichterle and D. Lím, Hydrophilic Gels for Biological Use, Nature, 1960, 185(4706), 117–118 CrossRef.
- G. A. Silva, C. Czeisler, K. L. Niece, E. Beniash, D. A. Harrington and J. A. Kessler, et al., Selective Differentiation of Neural Progenitor Cells by High-Epitope Density Nanofibers, Science, 2004, 303(5662), 1352–1355 CrossRef CAS PubMed.
- B. D. Ratner, A. S. Hoffman, F. J. Schoen and J. E. Lemons, Biomaterials science: an introduction to materials in medicine, Chem. Eng., 2004, 26 Search PubMed.
- M. Dong and Y. Chen, The stimuli-responsive properties of hydrogels based on natural polymers, Hydrogels Based on Natural Polymers, Elsevier Inc., 2019, pp. 173–222 Search PubMed.
- A. Tanaka, Y. Fukuoka, Y. Morimoto, T. Honjo, D. Koda and M. Goto, et al., Cancer cell death induced by the intracellular self-assembly of an enzyme-responsive supramolecular gelator, J. Am. Chem. Soc., 2015, 137(2), 770–775 CrossRef CAS PubMed.
- D. Ishii, D. Tatsumi, T. Matsumoto, K. Murata, H. Hayashi and H. Yoshitani, Investigation of the structure of cellulose in LiCl/DMAc solution and its gelation behavior by small-angle X-ray scattering measurements, Macromol. Biosci., 2006, 6(4), 293–300 CrossRef CAS PubMed.
- H. Song, Y. Niu, Z. Wang and J. Zhang, Liquid crystalline phase and gel-sol transitions for concentrated microcrystalline cellulose (MCC)/1-ethyl-3-methylimidazolium acetate (EMIMAc) solutions, Biomacromolecules, 2011, 12(4), 1087–1096 CrossRef CAS PubMed.
- Molecular interactions (noncovalent interactions).
- R. Koprowski, Book review of “The Biomedical Engineering Handbook” fourth edition, edited by Joseph D. Bronzino, Donald R. Peterson. Biomed. Eng. Online. 2016;15(1): 1. DOI:10.1186/s12938-015-0119-0.
- G. Bartkowiak and I. Frydrych, Superabsorbents and their medical applications, Handbook of Medical Textiles, 2011 Search PubMed.
- T. Maki, R. Yoshisaki, S. Akama and M. Yamanaka, Enzyme responsive properties of amphiphilic urea supramolecular hydrogels, Polym. J., 2020, 2020, 1–8 Search PubMed.
- Z. Yang and B. Xu, Supramolecular hydrogels based on biofunctional nanofibers of self-assembled small molecules, J. Mater. Chem., 2007, 17(23), 2385–2393 RSC.
- A. R. Hirst, B. Escuder, J. F. Miravet and D. K. Smith, High-Tech Applications of Self-Assembling Supramolecular Nanostructured Gel-Phase Materials: From Regenerative Medicine to Electronic Devices, Angew. Chem., Int. Ed., 2008, 47(42), 8002–8018 CrossRef CAS PubMed.
- M. de Loos, J. H. van Esch, R. M. Kellogg and B. L. Feringa, C3-Symmetric, amino acid based organogelators and thickeners: a systematic study of structure-property relations, Tetrahedron, 2007, 63(31), 7285–7301 CrossRef CAS.
- L. A. Estroff and A. D. Hamilton, Water gelation by small organic molecules, Chem. Rev., 2004, 104(3), 1201–1217 CrossRef CAS PubMed.
- R. Zhang, A. Bowyer, R. Eisenthal and J. Hubble, A smart membrane based on an antigen-responsive hydrogel, Biotechnol. Bioeng., 2007, 97, 976–984 CrossRef CAS PubMed.
- Y. Huang, Y. Ma, Y. Chen, X. Wu, L. Fang and Z. Zhu, et al., Target-responsive DNAzyme cross-linked hydrogel for visual quantitative detection of lead, Anal. Chem., 2014, 86(22), 11434–11439 CrossRef CAS PubMed.
- R. Liu, Y. Huang, Y. Ma, S. Jia, M. Gao and J. Li, et al., Design and synthesis of target-responsive aptamer-cross-linked hydrogel for visual quantitative detection of ochratoxin A, ACS Appl. Mater. Interfaces, 2015, 7(12), 6982–6990 CrossRef CAS PubMed.
- B. Lin, Z. Guan, Y. Song, E. Song, Z. Lu and D. Liu, et al., Lateral flow assay with pressure meter readout for rapid point-of-care detection of disease-associated protein, Lab Chip, 2018, 18(6), 965–970 RSC.
- D. Liu, S. Jia, H. Zhang, Y. Ma, Z. Guan and J. Li, et al., Integrating Target-Responsive Hydrogel with Pressuremeter Readout Enables Simple, Sensitive, User-Friendly, Quantitative Point-of-Care Testing, ACS Appl. Mater. Interfaces, 2017, 9(27), 22252–22258, DOI:10.1021/acsami.7b05531.
- Q. Wang, S. Li, Z. Wang, H. Liu and C. Li, Preparation and characterization of a positive thermoresponsive hydrogel for drug loading and release, J. Appl. Polym. Sci., 2009, 111(3), 1417–1425 CrossRef CAS.
- K. Itoh, M. Yahaba and A. Takahashi, RT-I journal of, 2008 undefined, In situ gelling xyloglucan/pectin formulations for oral sustained drug delivery, Elsevier.
- P. Alexandridis and T. Alan Hatton, Poly(ethylene oxide)poly(propylene oxide)poly(ethylene oxide) block copolymer surfactants in aqueous solutions and at interfaces: thermodynamics, structure, dynamics, and modeling, Colloids Surf., A, 1995, 96, 1–46 CrossRef CAS.
- Z. Li, X. Guo, S. Matsushita and J. Guan, Differentiation of cardiosphere-derived cells into a mature cardiac lineage using biodegradable poly(N-isopropylacrylamide) hydrogels, Biomaterials, 2011, 32(12), 3220–3232 CrossRef CAS.
- S. C. Cheng, W. Feng, I. I. Pashikin, L. H. Yuan, H. C. Deng and Y. Zhou, Radiation polymerization of thermo-sensitive poly (N-vinylcaprolactam), Radiat. Phys. Chem., 2002, 63(3–6), 517–519 CrossRef CAS.
- P. Jiang, X. Sheng, S. Yu, H. Li, J. Lu and J. Zhou, et al., Preparation and characterization of thermo-sensitive gel with phenolated alkali lignin, Sci. Rep., 2018, 8(1), 1–10, DOI:10.1038/s41598-018-32672-z.
- H. Gai, J. Wu, C. Wu, X. Sun, F. Jia and Y. Yu, Synthesis and characterization of thermosensitive hydrogel with improved mechanical properties, J. Mater. Res., 2015, 30(16), 2400–2407, DOI:10.1557/jmr.2015.233.
- H. Jiang, L. Fan, S. Yan, F. Li, H. Li and J. Tang, Tough and electro-responsive hydrogel actuators with bidirectional bending behavior, Nanoscale, 2019, 11(5), 2231–2237 RSC.
- L. Peng, Y. Liu, J. Huang, J. Li, J. Gong and J. Ma, Microfluidic fabrication of highly stretchable and fast electro-responsive graphene oxide/polyacrylamide/alginate hydrogel fibers, Eur. Polym. J., 2018, 103, 335–341, DOI:10.1016/j.eurpolymj.2018.04.019.
- Y. Li, Y. Sun, Y. Xiao, G. Gao, S. Liu and J. Zhang, et al., Electric Field Actuation of Tough Electroactive Hydrogels Cross-Linked by Functional Triblock Copolymer Micelles, ACS Appl. Mater. Interfaces, 2016, 8(39), 26326–26331 CrossRef CAS PubMed.
- C. M. Daikuzono, C. Delaney, H. Tesfay, L. Florea, O. N. Oliveira and A. Morrin, et al., Impedance spectroscopy for monosaccharides detection using responsive hydrogel modified paper-based electrodes, Analyst, 2017, 142(7), 1133–1139 RSC.
- S. Botelho da Silva, M. Krolicka, L. A. M. van den Broek, A. E. Frissen and C. G. Boeriu, Water-soluble chitosan derivatives and pH-responsive hydrogels by selective C-6 oxidation mediated by TEMPO-laccase redox system, Carbohydr. Polym., 2018, 186, 299–309 CrossRef CAS PubMed.
- L. Xu, L. Qiu, Y. Sheng, Y. Sun, L. Deng and X. Li, et al., Biodegradable pH-responsive hydrogels for controlled dual-drug release, J. Mater. Chem. B, 2018, 6(3), 510–517 RSC.
- N. Kanmaz, D. Saloglu and J. Hizal, Humic acid embedded chitosan/poly (vinyl alcohol) pH-sensitive hydrogel: Synthesis, characterization, swelling kinetic and diffusion coefficient, Chem. Eng. Commun., 2019, 206(9), 1168–1180, DOI:10.1080/00986445.2018.1550396.
- C. Jin, W. Song, T. Liu, J. Xin, W. C. Hiscox and J. Zhang, et al., Temperature and pH Responsive Hydrogels Using Methacrylated Lignosulfonate Cross-Linker: Synthesis, Characterization, and Properties, ACS Sustainable Chem. Eng., 2018, 6(2), 1763–1771 CrossRef CAS.
- Y. Liang, X. Zhao, P. X. Ma, B. Guo, Y. Du and X. Han, pH-responsive injectable hydrogels with mucosal adhesiveness based on chitosan-grafted-dihydrocaffeic acid and oxidized pullulan for localized drug delivery, J. Colloid Interface Sci., 2019, 536, 224–234, DOI:10.1016/j.jcis.2018.10.056.
- Z. Wang, X. Han, Y. Wang, K. Men, L. Cui and J. Wu, et al., Facile preparation of low swelling, high strength, self-healing and pH-responsive hydrogels based on the triple-network structure, Front. Mater. Sci., 2019, 13(1), 54–63 CrossRef.
- T. Chen, P. J. Colver and S. A. F. Bon, Organic-Inorganic Hybrid Hollow Spheres Prepared from TiO2-Stabilized Pickering Emulsion Polymerization, Adv. Mater., 2007, 19, 2286–2289 CrossRef CAS.
- W. Zhao, K. Odelius, U. Edlund, C. Zhao and A. C. Albertsson, In Situ Synthesis of Magnetic Field-Responsive Hemicellulose Hydrogels for Drug Delivery, Biomacromolecules, 2015, 16(8), 2522–2528 CrossRef CAS PubMed.
- R. Messing, N. Frickel, L. Belkoura, R. Strey, H. Rahn and S. Odenbach, et al., Cobalt ferrite nanoparticles as multifunctional cross-linkers in PAAm ferrohydrogels, Macromolecules, 2011, 44(8), 2990–2999 CrossRef CAS.
- D. Huang, M. Sun, Y. Bu, F. Luo, C. Lin and Z. Lin, et al., Microcapsule-embedded hydrogel patches for ultrasound responsive and enhanced transdermal delivery of diclofenac sodium, J. Mater. Chem. B, 2019, 7(14), 2330–2337 RSC.
- T. Emi, K. Michaud, E. Orton, G. Santilli, C. Linh and M. O’Connell, et al., Ultrasonic generation of pulsatile and sequential therapeutic delivery profiles from Calcium-crosslinked alginate hydrogels, Molecules, 2019, 24(6), 1048 CrossRef CAS.
- A. Alford, B. Tucker, V. Kozlovskaya, J. Chen, N. Gupta and R. Caviedes, et al., Encapsulation and ultrasound-triggered release of G-Quadruplex DNA in multilayer hydrogel microcapsules, Polymers, 2018, 10(12), 1342 CrossRef PubMed.
- G. Li, Y. Wang, S. Wang and J. Jiang, A Tough Composite Hydrogel can Controllably Deliver Hydrophobic Drugs under Ultrasound, Macromol. Mater. Eng., 2018, 303(3), 1700483 CrossRef.
- C. H. Wu, M. K. Sun, J. Shieh, C. S. Chen, C. W. Huang and C. A. Dai, et al., Ultrasound-responsive NIPAM-based hydrogels with tunable profile of controlled release of large molecules, Ultrasonics, 2018, 83, 157–163 CrossRef CAS PubMed.
- S. Yamaguchi, K. Higashi, T. Azuma and A. Okamoto, Supramolecular polymeric hydrogels for ultrasound-guided protein release, Biotechnol. J., 2019, 14(5), 1800530 CrossRef.
- C. H. Wu, M. K. Sun, Y. Kung, Y. C. Wang, S. L. Chen and H. H. Shen, et al., One injection for one-week controlled release: In vitro and in vivo assessment of ultrasound-triggered drug release from injectable thermoresponsive biocompatible hydrogels, Ultrason. Sonochem., 2020, 62, 104875 CrossRef CAS PubMed.
- Injectable Self-Healing Glucose-Responsive Hydrogels with pH-Regulated Mechanical Properties.pdf.
- M. Peña Alvarez, P. Mayorga Burrezo, T. Iwamoto, L. Qiu, M. Kertesz and M. Taravillo, et al., Chameleon-like behaviour of cyclo[n]paraphenylenes in complexes with C\n 70\n: on their impressive electronic and structural adaptability as probed by Raman spectroscopy, Faraday Discuss., 2014, 4(8), 1166–1169 Search PubMed.
- A. Matsumoto, M. Tanaka, H. Matsumoto, K. Ochi, Y. Moro-Oka and H. Kuwata, et al., Synthetic “smart gel” provides glucose-responsive insulin delivery in diabetic mice, Sci. Adv., 2017, 3(11), eaaq0723 CrossRef PubMed.
- J. D. Ehrick, M. R. Luckett, S. Khatwani, Y. Wei, S. K. Deo and L. G. Bachas, et al., Glucose responsive hydrogel networks based on protein recognition, Macromol. Biosci., 2009, 9(9), 864–868 CrossRef CAS PubMed.
- H. Y. Liu, M. Korc and C. C. Lin, Biomimetic and enzyme-responsive dynamic hydrogels for studying cell-matrix interactions in pancreatic ductal adenocarcinoma, Biomaterials, 2018, 160, 24–36 CrossRef CAS PubMed.
- C. C. Lin, A. Raza and H. Shih, PEG hydrogels formed by thiol-ene photo-click chemistry and their effect on the formation and recovery of insulin-secreting cell spheroids, Biomaterials, 2011, 32(36), 9685–9695 CrossRef CAS PubMed.
- C. C. Lin, C. S. Ki and H. Shih, Thiol-norbornene photoclick hydrogels for tissue engineering applications, J. Appl. Polym. Sci., 2015, 132(8), 41563, DOI:10.1002/app.41563.
- Z. Mũnoz, H. Shih and C. C. Lin, Gelatin hydrogels formed by orthogonal thiol-norbornene photochemistry for cell encapsulation, Biomater. Sci., 2014, 2(8), 1063–1072 RSC.
- H. Y. Liu, T. Greene, T. Y. Lin, C. S. Dawes, M. Korc and C. C. Lin, Enzyme-mediated stiffening hydrogels for probing activation of pancreatic stellate cells, Acta Biomater., 2017, 48, 258–269 CrossRef CAS PubMed.
- T. Su, Z. Tang, H. He, W. Li, X. Wang and C. Liao, et al., Glucose oxidase triggers gelation of N-hydroxyimide-heparin conjugates to form enzyme-responsive hydrogels for cell-specific drug delivery, Chem. Sci., 2014, 5(11), 4204–4209 RSC.
- X. Yao, L. Chen, J. Ju, C. Li, Y. Tian and L. Jiang, et al., Superhydrophobic Diffusion Barriers for Hydrogels via Confined Interfacial Modification, Adv. Mater., 2016, 28(34), 7383–7389 CrossRef CAS PubMed.
- L. Chen, Y. Yin, Y.-X. Liu, L. Lin and M. J. Liu, Design and fabrication of functional hydrogels through interfacial engineering, Chin. J. Polym. Sci., 2017, 35, 1181–1193 CrossRef CAS.
- L. Lin, H. Yi, X. Guo, P. Zhang, L. Chen and D. Hao, et al., Nonswellable hydrogels with robust micro/nano-structures and durable superoleophobic surfaces under seawater, Sci. China Chem., 2018, 61(1), 64–70, DOI:10.1007/s11426-017-9149-x.
- S. Rose, A. Prevoteau, P. Elzière, D. Hourdet, A. Marcellan and L. Leibler, Nanoparticle solutions as adhesives for gels and biological tissues, Nature, 2014, 505(7483), 382–385, DOI:10.1038/nature12806.
- X. Huang, Y. Sun and S. Soh, Stimuli-Responsive Surfaces for Tunable and Reversible Control of Wettability, Adv. Mater., 2015, 27(27), 4062–4068, DOI:10.1002/adma.201501578.
- N. M. Oliveira, Y. S. Zhang, J. Ju, A.-Z. Chen, Y. Chen and S. R. Sonkusale, et al., Hydrophobic Hydrogels: Toward Construction of Floating (Bio)microdevices, Chem. Mater., 2016, 28(11), 3641–3648, DOI:10.1021/acs.chemmater.5b04445.
- L. Chen, X. Yao, Z. Gu, K. Zheng, C. Zhao and W. Lei, et al., Covalent tethering of photo-responsive superficial layers on hydrogel surfaces for photo-controlled release, Chem. Sci., 2017, 8(3), 2010–2016, 10.1039/C6SC04634G.
- C. Xu, R. He, B. Xie, M. Ismail, C. Yao and J. Luan, et al., Silicone hydrogels grafted with natural amino acids for ophthalmological application, J. Biomater. Sci., Polym. Ed., 2016, 27(13), 1354–1368, DOI:10.1080/09205063.2016.1201916.
- L. Xu, P. Ma, B. Yuan, Q. Chen, S. Lin and X. Chen, et al., Anti-biofouling contact lenses bearing surface-immobilized layers of zwitterionic polymer by one-step modification, RSC Adv., 2014, 4(29), 15030–15035, 10.1039/C3RA47119E.
- X. Deng, M. Korogiannaki, B. Rastegari, J. Zhang, M. Chen and Q. Fu, et al., “click” Chemistry-Tethered Hyaluronic Acid-Based Contact Lens Coatings Improve Lens Wettability and Lower Protein Adsorption, ACS Appl. Mater. Interfaces, 2016, 8(34), 22064–22073 CrossRef CAS PubMed.
- X. Hu, H. Tan, X. Wang and P. Chen, Surface functionalization of hydrogel by thiol-yne click chemistry for drug delivery, Colloids Surf., A, 2016, 489, 297–304 CrossRef CAS . Available from: http://www.sciencedirect.com/science/article/pii/S0927775715303277.
- Y. Guo, J. Bae, F. Zhao and G. Yu, Functional Hydrogels for Next-Generation Batteries and Supercapacitors, Trends Cogn. Sci., 2019, 1(3), 335–348 CAS.
- Y. S. Zhang and A. Khademhosseini, Advances in engineering hydrogels, Science, 2017, 356(6337), 1–10, DOI:10.1126/science.aaf3627.
- K. Tian, J. Bae, S. E. Bakarich, C. Yang, R. D. Gately and G. M. Spinks, et al., 3D Printing of Transparent and Conductive Heterogeneous Hydrogel–Elastomer Systems, Adv. Mater., 2017, 29(10), 1604827 CrossRef PubMed.
- H. P. Cong, X. C. Ren, P. Wang and S. H. Yu, Macroscopic multifunctional graphene-based hydrogels and aerogels by a metal ion induced self-assembly process, ACS Nano, 2012, 6(3), 2693–2703 CrossRef CAS PubMed.
- H. Qin, T. Zhang, H. N. Li, H. P. Cong, M. Antonietti and S. H. Yu, Dynamic Au-Thiolate Interaction Induced Rapid Self-Healing Nanocomposite Hydrogels with Remarkable Mechanical Behaviors, Chem, 2017, 3(4), 691–705 CAS.
- S. Hong, D. Sycks, H. F. Chan, S. Lin, G. P. Lopez and F. Guilak, et al., 3D Printing of Highly Stretchable and Tough Hydrogels into Complex, Cellularized Structures, Adv. Mater., 2015, 27(27), 4035–4040 CrossRef CAS PubMed.
- S. Lin, H. Yuk, T. Zhang, G. A. Parada, H. Koo and C. Yu, et al., Stretchable Hydrogel Electronics and Devices, Adv. Mater., 2016, 28(22), 4497–4505 CrossRef CAS PubMed.
- X. Liu, T. C. Tang, E. Tham, H. Yuk, S. Lin and T. K. Lu, et al., Stretchable living materials and devices with hydrogel-elastomer hybrids hosting programmed cells, Proc. Natl. Acad. Sci. U. S. A., 2017, 114(9), 2200–2205 CrossRef CAS PubMed.
- M. Sun, R. Bai, X. Yang, J. Song, M. Qin and Z. Suo, et al., Hydrogel Interferometry for Ultrasensitive and Highly Selective Chemical Detection, Adv. Mater., 2018, 30(46), 1804916 CrossRef PubMed.
- J. Sun, J. Du, J. Yang and J. Wang, Shear-horizontal waves in a rotated Y-cut quartz plate in contact with a viscous fluid, Ultrasonics, 2012, 52(1), 133–137 CrossRef CAS PubMed.
- Y. Yue, X. Wang, J. Han, L. Yu, J. Chen and Q. Wu, et al., Effects of nanocellulose on sodium alginate/polyacrylamide hydrogel: Mechanical properties and adsorption-desorption capacities, Carbohydr. Polym., 2019, 206, 289–301, DOI:10.1016/j.carbpol.2018.10.105.
- Y. Chen, K. Zheng, L. Niu, Y. Zhang, Y. Liu and C. Wang, et al., Highly mechanical properties nanocomposite hydrogels with biorenewable lignin nanoparticles, Int. J. Biol. Macromol., 2019, 128, 414–420, DOI:10.1016/j.ijbiomac.2019.01.099.
- C. Chen, D. Li, H. Yano and K. Abe, Insect Cuticle-Mimetic Hydrogels with High Mechanical Properties Achieved via the Combination of Chitin Nanofiber and Gelatin, J. Agric. Food Chem., 2019, 67(19), 5571–5578 CrossRef CAS PubMed.
- W. Liu, M. Song, B. Kong and Y. Cui, Flexible and Stretchable Energy Storage: Recent Advances and Future Perspectives, Adv. Mater., 2017, 29(1), 1603436, DOI:10.1002/adma.201603436.
- Y. Zhao, S. Chen, J. Hu, J. Yu, G. Feng and B. Yang, et al., Microgel-Enhanced Double Network Hydrogel Electrode with High Conductivity and Stability for Intrinsically Stretchable and Flexible All-Gel-State Supercapacitor, ACS Appl. Mater. Interfaces, 2018, 10(23), 19323–19330 CrossRef CAS PubMed.
- F. Wahid, X. H. Hu, L. Q. Chu, S. R. Jia, Y. Y. Xie and C. Zhong, Development of bacterial cellulose/chitosan based semi-interpenetrating hydrogels with improved mechanical and antibacterial properties, Int. J. Biol. Macromol., 2019, 122, 380–387, DOI:10.1016/j.ijbiomac.2018.10.105.
- H. Itagaki, T. Kurokawa, H. Furukawa, T. Nakajima, Y. Katsumoto and J. P. Gong, Water-Induced Brittle-Ductile Transition of Double Network Hydrogels, Macromolecules, 2010, 43(22), 9495–9500, DOI:10.1021/ma101413j.
- T. Nakajima, H. Furukawa, Y. Tanaka, T. Kurokawa, Y. Osada and J. P. Gong, True Chemical Structure of Double Network Hydrogels, Macromolecules, 2009, 42(6), 2184–2189, DOI:10.1021/ma802148p.
- X. Yan, J. Yang, F. Chen, L. Zhu, Z. Tang and G. Qin, et al., Mechanical properties of gelatin/polyacrylamide/graphene oxide nanocomposite double-network hydrogels, Compos. Sci. Technol., 2018, 163, 81–88, DOI:10.1016/j.compscitech.2018.05.011.
- X. Li, H. Qin, X. Zhang and Z. Guo, Triple-network hydrogels with high strength, low friction and self-healing by chemical-physical crosslinking, J. Colloid Interface Sci., 2019, 556, 549–556, DOI:10.1016/j.jcis.2019.08.100.
- M. T. I. Mredha, S. K. Pathak, V. T. Tran, J. Cui and I. Jeon, Hydrogels with superior mechanical properties from the synergistic effect in hydrophobic–hydrophilic copolymers, Chem. Eng. J., 2019, 362, 325–338, DOI:10.1016/j.cej.2018.12.023.
- F. Chen, S. Lu, L. Zhu, Z. Tang, Q. Wang and G. Qin, et al., Conductive regenerated silk-fibroin-based hydrogels with integrated high mechanical performances, J. Mater. Chem. B, 2019, 7(10), 1708–1715 RSC.
- Q. Zhou, K. Yang, J. He, H. Yang and X. Zhang, A novel 3D-printable hydrogel with high mechanical strength and shape memory properties, J. Mater. Chem. C, 2019, 7(47), 14913–14922 RSC.
- X. Luo, M. Y. Akram, Y. Yuan, J. Nie and X. Zhu, Silicon dioxide/poly(vinyl alcohol) composite hydrogels with high mechanical properties and low swellability, J. Appl. Polym. Sci., 2019, 136(1), 1–6 CrossRef.
- Manuscript A. Materials Chemistry B, 2020.
- X. Li, Y. Zhao, D. Li, G. Zhang, S. Long and H. Wang, Hybrid dual crosslinked polyacrylic acid hydrogels with ultrahigh mechanical strength, toughness and self-healing properties via soaking salt solution, Polymer, 2017, 121, 55–63 CrossRef CAS.
- P. Li, J. Zhao, Y. Chen, B. Cheng, Z. Yu and Y. Zhao, et al., Preparation and characterization of chitosan physical hydrogels with enhanced mechanical and antibacterial properties, Carbohydr. Polym., 2017, 157, 1383–1392 CrossRef CAS.
- C. B. Rodell, N. N. Dusaj, C. B. Highley and J. A. Burdick, Injectable and Cytocompatible Tough Double-Network Hydrogels through Tandem Supramolecular and Covalent Crosslinking, Adv. Mater., 2016, 28(38), 8419–8424 CrossRef CAS PubMed.
- Y. Zhou, P. F. Damasceno, B. S. Somashekar, M. Engel, F. Tian and J. Zhu, et al., Unusual multiscale mechanics of biomimetic nanoparticle hydrogels, Nat. Commun., 2018, 9(1), 181, DOI:10.1038/s41467-017-02579-w.
- T. Huang, H. Xu, K. Jiao, L. Zhu, H. R. Brown and H. Wang, A novel hydrogel with high mechanical strength: A macromolecular microsphere composite hydrogel, Adv. Mater., 2007, 19(12), 1622–1626 CrossRef CAS.
- T. L. Sun, T. Kurokawa, S. Kuroda, A. B. Ihsan, T. Akasaki and K. Sato, et al., Physical hydrogels composed of polyampholytes demonstrate high toughness and viscoelasticity, Nat. Mater., 2013, 12(10), 932–937 CrossRef CAS PubMed.
- D. J. Sarkar and A. Singh, pH-triggered release of boron and thiamethoxam from boric acid crosslinked carboxymethyl cellulose hydrogel based formulations, Polym.-Plast. Technol. Eng., 2018, 58(1), 83–96 Search PubMed.
- H. Yuk, T. Zhang, G. A. Parada, X. Liu and X. Zhao, Skin-inspired hydrogel-elastomer hybrids with robust interfaces and functional microstructures, Nat. Commun., 2016, 7(1), 12028, DOI:10.1038/ncomms12028.
- A. A. Oun and J.-W. Rhim, Carrageenan-based hydrogels and films: Effect of ZnO and CuO nanoparticles on the physical, mechanical, and antimicrobial properties Biodegradable films with addition of antimicrobial and antioxidant activity View project Isolation of nanocellulose using, Elsevier, 2017.
- T. Wu, J. Huang, Y. Jiang, Y. Hu, X. Ye and D. Liu, et al., Formation of hydrogels based on chitosan/alginate for the delivery of lysozyme and their antibacterial activity, Food Chem., 2018, 240, 361–369 CrossRef CAS PubMed.
- Chemistry MZ-TT in A, 2017 undefined, Application of nanocomposite polymer hydrogels for ultra-sensitive fluorescence detection of proteins in gel electrophoresis, Elsevier.
- S. Azevedo, A. M. S. Costa, A. Andersen, I. S. Choi, H. Birkedal and J. F. Mano, Bioinspired Ultratough Hydrogel with Fast Recovery, Self-Healing, Injectability and Cytocompatibility, Adv. Mater., 2017, 29(28), 1700759, DOI:10.1002/adma.201700759.
- S. Li, S. Dong, W. Xu, S. Tu, L. Yan and C. Zhao, et al., Antibacterial Hydrogels, Adv. Sci., 2018, 5(5), 1700527, DOI:10.1002/advs.201700527.
- B. Chen and J. Wang, Antimicrobial hydrogels: promising materials for medical application, Int. J. Nanomed., 2018, 13, 2217–2263, DOI:10.2147/IJN.S154748.
- Y. Lu, Y. Mei, M. Drechsler and M. Ballauff, Thermosensitive core-shell particles as carriers for Ag nanoparticles: Modulating the catalytic activity by a phase transition in networks, Angew. Chem., Int. Ed., 2006, 45(5), 813–816 CrossRef CAS PubMed.
- H. Chi, Y. Qiao, B. Wang, Y. Hou, Q. Li and K. Li, et al., Swelling, thermal stability, antibacterial properties enhancement on composite hydrogel synthesized by chitosan-acrylic acid and ZnO nanowires, Polym. Technol. Mater., 2019, 58(15), 1649–1661 CAS.
- E. M. Abdel Bary, A. N. Harmal, A. Saeed and M. A. Gouda, Design, Synthesis, Characterization, Swelling and in Vitro Drug Release Behavior of Composite Hydrogel Beads Based on Methotrexate and Chitosan Incorporating Antipyrine Moiety, Polym.-Plast.
Technol. Eng., 2018, 57(18), 1906–1914 CrossRef CAS.
- Y. Wang, J. Wang, Z. Yuan, H. Han, T. Li and L. Li, et al., Chitosan cross-linked poly(acrylic acid) hydrogels: Drug release control and mechanism, Colloids Surf., B, 2017, 152, 252–259 CrossRef CAS PubMed.
- S. Mondal, Review on Nanocellulose Polymer Nanocomposites, Polym.-Plast. Technol. Eng., 2018, 57, 1377–1391 CrossRef CAS.
- H. S. Samanta and S. K. Ray, Controlled release of tinidazole and theophylline from chitosan based composite hydrogels, Carbohydr. Polym., 2014, 106(1), 109–120 CrossRef CAS PubMed.
- H.-W. Chang, Y.-S. Lin, Y.-D. Tsai and M.-L. Tsai, Effects of chitosan characteristics on the physicochemical properties, antibacterial activity, and cytotoxicity of chitosan/2-glycerophosphate/nanosilver hydrogels, J. Appl. Polym. Sci., 2013, 127(1), 169–176, DOI:10.1002/app.37855.
- H. Tang, A. Lu, L. Li, W. Zhou, Z. Xie and L. Zhang, Highly antibacterial materials constructed from silver molybdate nanoparticles immobilized in chitin matrix, Chem. Eng. J., 2013, 234, 124–131 CrossRef CAS . Available from: http://www.sciencedirect.com/science/article/pii/S1385894713011492.
- T. Jayaramudu, G. M. Raghavendra, K. Varaprasad, R. Sadiku, K. Ramam and K. M. Raju, Iota-Carrageenan-based biodegradable AgO nanocomposite hydrogels for the inactivation of bacteria, Carbohydr. Polym., 2013, 95(1), 188–194 CrossRef CAS PubMed.
- K. A. Juby, C. Dwivedi, M. Kumar, S. Kota, H. S. Misra and P. N. Bajaj, Silver nanoparticle-loaded PVA/gum acacia hydrogel: Synthesis, characterization and antibacterial study, Carbohydr. Polym., 2012, 89(3), 906–913 CrossRef CAS PubMed . Available from: http://www.sciencedirect.com/science/article/pii/S0144861712003736.
- J. Stojkovska, D. Kostić, Ž. Jovanović, M. Vukašinović-Sekulić, V. Mišković-Stanković and B. Obradović, A comprehensive approach to in vitro functional evaluation of Ag/alginate nanocomposite hydrogels, Carbohydr. Polym., 2014, 111, 305–314 CrossRef CAS PubMed . Available from: http://www.sciencedirect.com/science/article/pii/S0144861714004202.
- H. Ghasemzadeh and F. Ghanaat, Antimicrobial alginate/PVA silver nanocomposite hydrogel, synthesis and characterization, J. Polym. Res., 2014, 21(3), 355, DOI:10.1007/s10965-014-0355-1.
- M. Zafar, T. Shah, A. Rawal and E. Siores, Preparation and characterisation of thermoresponsive nanogels for smart antibacterial fabrics, Mater. Sci. Eng., C, 2014, 40, 135–141 CrossRef CAS PubMed . Available from: http://www.sciencedirect.com/science/article/pii/S0928493114001593.
- K. Madhusudana Rao, K. S. V. Krishna Rao, G. Ramanjaneyulu, K. Chowdoji Rao, M. C. S. Subha and C.-S. Ha, Biodegradable sodium alginate-based semi-interpenetrating polymer network hydrogels for antibacterial application, J. Biomed. Mater. Res., Part A, 2014, 102(9), 3196–3206, DOI:10.1002/jbm.a.34991.
- K. Sivudu and K. Y. Rhee, Preparation and characterization of pH-responsive hydrogel magnetite nanocomposite, Colloids Surf., A, 2009, 349, 29–34 CrossRef CAS.
- M. Yadollahi, S. Farhoudian and H. Namazi, One-pot synthesis of antibacterial chitosan/silver bio-nanocomposite hydrogel beads as drug delivery systems, Int. J. Biol. Macromol., 2015, 79, 37–43 CrossRef CAS PubMed . Available from: http://www.sciencedirect.com/science/article/pii/S0141813015002706.
- E. Bozaci, E. Akar, E. Ozdogan, A. Demir, A. Altinisik and Y. Seki, Application of carboxymethylcellulose hydrogel based silver nanocomposites on cotton fabrics for antibacterial property, Carbohydr. Polym., 2015, 134, 128–135 CrossRef CAS PubMed Available from.
- T. Jiao, H. Guo, Q. Zhang, Q. Peng, Y. Tang and X. Yan, et al., Reduced Graphene Oxide-Based Silver
Nanoparticle-Containing Composite Hydrogel as Highly Efficient Dye Catalysts for Wastewater Treatment, Sci. Rep., 2015, 5, 11873 CrossRef CAS PubMed . Available from: https://pubmed.ncbi.nlm.nih.gov/26183266.
- S. Bhowmick, S. Mohanty and V. Koul, Fabrication of transparent quaternized PVA/silver nanocomposite hydrogel and its evaluation as an antimicrobial patch for wound care systems, J. Mater. Sci.: Mater. Med., 2016, 27(11), 160, DOI:10.1007/s10856-016-5772-8.
- M. Ribeiro, M. P. Ferraz, F. J. Monteiro, M. H. Fernandes, M. M. Beppu and D. Mantione, et al., Antibacterial silk fibroin/nanohydroxyapatite hydrogels with silver and gold nanoparticles for bone regeneration. Nanomedicine Nanotechnology, Biol. Med., 2017, 13(1), 231–239 CAS . Available from: http://www.sciencedirect.com/science/article/pii/S1549963416301423.
- A. L. Daniel-da-Silva, A. M. Salgueiro and T. Trindade, Effects of Au nanoparticles on thermoresponsive genipin-crosslinked gelatin hydrogels, Gold Bull., 2013, 46(1), 25–33, DOI:10.1007/s13404-012-0078-1.
- W. Gao, D. Vecchio, J. Li, J. Zhu, Q. Zhang and V. Fu, et al., Hydrogel Containing Nanoparticle-Stabilized Liposomes for Topical Antimicrobial Delivery, ACS Nano, 2014, 8(3), 2900–2907, DOI:10.1021/nn500110a.
- A. Mohandas, P. T. S. Kumar, B. Raja, V.-K. Lakshmanan and R. Jayakumar, Exploration of alginate hydrogel/nano zinc oxide composite bandages for infected wounds, Int. J. Nanomed., 2015, 10(Suppl. 1), 53–66 CAS . Available from: https://pubmed.ncbi.nlm.nih.gov/26491307.
- J. Wang, H. Hu, Z. Yang, J. Wei and J. Li, IPN hydrogel nanocomposites based on agarose and ZnO with antifouling and bactericidal properties, Mater. Sci. Eng., C, 2016, 61, 376–386 CrossRef CAS PubMed . Available from: http://www.sciencedirect.com/science/article/pii/S0928493115306354.
- F. Wahid, J.-J. Yin, D.-D. Xue, H. Xue, Y.-S. Lu and C. Zhong, et al., Synthesis and characterization of antibacterial carboxymethyl Chitosan/ZnO nanocomposite hydrogels, Int. J. Biol. Macromol., 2016, 88, 273–279 CrossRef CAS PubMed . Available from: http://www.sciencedirect.com/science/article/pii/S0141813016302689.
- N. A. Kumar, N. S. Rejinold, P. Anjali, A. Balakrishnan, R. Biswas and R. Jayakumar, Preparation of chitin nanogels containing nickel nanoparticles, Carbohydr. Polym., 2013, 97(2), 469–474 CrossRef CAS PubMed . Available from: http://www.sciencedirect.com/science/article/pii/S0144861713004840.
- H. Hezaveh and I. I. Muhamad, Impact of metal oxide nanoparticles on oral release properties of pH-sensitive hydrogel nanocomposites, Int. J. Biol. Macromol., 2012, 50(5), 1334–1340 CrossRef CAS PubMed . Available from: http://www.sciencedirect.com/science/article/pii/S0141813012001146.
- M. R. Alison, Stem cells in pathobiology and regenerative medicine, J. Pathol., 2009, 217(2), 141–143, DOI:10.1002/path.2497.
- S. J. Morrison and A. C. Spradling, Stem cells and niches: mechanisms that promote stem cell maintenance throughout life, Cell, 2008, 132(4), 598–611 CrossRef CAS PubMed . Available from: https://pubmed.ncbi.nlm.nih.gov/18295578.
- K. Hu, N. Zhou, Y. Li, S. Ma, Z. Guo and M. Cao, et al., Sliced Magnetic Polyacrylamide Hydrogel with Cell-Adhesive Microarray Interface: A Novel Multicellular Spheroid Culturing Platform, ACS Appl. Mater. Interfaces, 2016, 8(24), 15113–15119 CrossRef CAS PubMed.
- D. Loessner, K. S. Stok, M. P. Lutolf, D. W. Hutmacher, J. A. Clements and S. C. Rizzi, Bioengineered 3D platform to explore cell-ECM interactions and drug resistance of epithelial ovarian cancer cells, Biomaterials, 2010, 31(32), 8494–8506 CrossRef CAS PubMed.
- M. J. Powers, R. E. Rodriguez and L. G. Griffith, Cell-substratum adhesion strength as a determinant of hepatocyte aggregate morphology, Biotechnol. Bioeng., 1997, 53(4), 415–426 CrossRef CAS.
- T. Dvir, B. P. Timko, M. D. Brigham, S. R. Naik, S. S. Karajanagi and O. Levy, et al., Nanowired three-dimensional cardiac patches, Nat. Nanotechnol., 2011, 6(11), 720–725 CrossRef CAS PubMed.
- E. B. Peters, N. Christoforou, K. W. Leong, G. A. Truskey and J. L. West, Poly(Ethylene Glycol) Hydrogel Scaffolds Containing Cell-Adhesive and Protease-Sensitive Peptides Support Microvessel Formation by Endothelial Progenitor Cells, Cell. Mol. Bioeng., 2016, 9(1), 38–54 CrossRef CAS PubMed.
- M. S. Hahn, L. J. Taite, J. J. Moon, M. C. Rowland, K. A. Ruffino and J. L. West, Photolithographic patterning of polyethylene glycol hydrogels, Biomaterials, 2006, 27(12), 2519–2524 CrossRef CAS PubMed.
- L. Han, L. Yan, K. Wang, L. Fang, H. Zhang and Y. Tang, et al., Tough, self-healable and tissue-adhesive hydrogel with tunable multifunctionality, NPG Asia Mater., 2017, 9(4), e372, DOI:10.1038/am.2017.33.
- M. M. Hasani-Sadrabadi, P. Sarrion, S. Pouraghaei, Y. Chau, S. Ansari and S. Li, et al., An engineered cell-laden adhesive hydrogel promotes craniofacial bone tissue regeneration in rats, Sci. Transl. Med., 2020, 12(534), 1–13, DOI:10.1126/scitranslmed.aay6853.
- S. A. Bencherif, R. W. Sands, D. Bhatta, P. Arany, C. S. Verbeke and D. A. Edwards, et al., Injectable preformed scaffolds with shape-memory properties, Proc. Natl. Acad. Sci. U. S. A., 2012, 109(48), 19590–19595 CrossRef CAS PubMed.
- J. H. Waite, N. H. Andersen, S. Jewhurst and C. Sun, Mussel adhesion: Finding the tricks worth mimicking, J. Adhes., 2005, 81(3–4), 297–317 CrossRef CAS.
- J. Yu, W. Wei, E. Danner, R. K. Ashley, J. N. Israelachvili and J. H. Waite, Mussel protein adhesion depends on interprotein thiol-mediated redox modulation, Nat. Chem. Biol., 2011, 7(9), 588–590 CrossRef CAS PubMed.
- A. Song, A. A. Rane and K. L. Christman, Antibacterial and cell-adhesive polypeptide and poly(ethylene glycol) hydrogel as a potential scaffold for wound healing, Acta Biomater., 2012, 8(1), 41–50 CrossRef CAS PubMed.
- K. P. Sai and M. Babu, Collagen based dressings – A review, Burns, 2000, 26(1), 54–62 CrossRef.
- S. P. Zhong, Y. Z. Zhang and C. T. Lim, Tissue scaffolds for skin wound healing and dermal reconstruction, Wiley Interdiscip. Rev.: Nanomed. Nanobiotechnol., 2010, 2(5), 510–525 CAS.
- L. G. Ovington, Advances in wound dressings, Clin. Dermatol., 2007, 25(1), 33–38 CrossRef PubMed.
- S. Cosson, E. A. Otte, H. Hezaveh and J. J. Cooper-White, Concise review: tailoring bioengineered scaffolds for stem cell applications in tissue engineering and regenerative medicine, Stem Cells Transl. Med., 2015, 4(2), 156–164 CrossRef CAS PubMed Available from: https://pubmed.ncbi.nlm.nih.gov/25575526.
- J.-Y. Lee, J.-E. Choo, H.-J. Park, J.-B. Park, S.-C. Lee and I. Jo, et al., Injectable gel with synthetic collagen-binding peptide for enhanced osteogenesis in vitro and in vivo, Biochem. Biophys. Res. Commun., 2007, 357(1), 68–74 CrossRef CAS PubMed . Available from: http://www.sciencedirect.com/science/article/pii/S0006291X07005505.
- Y. Liu and S. Hsu, Synthesis and Biomedical Applications of Self-healing Hydrogels, Front. Chem., 2018, 6, 449 CrossRef CAS PubMed . Available from: https://www.frontiersin.org/article/10.3389/fchem.2018.00449.
- S. Islam and G. S. Bhat, Progress and challenges in self-healing composite materials, Mater. Adv., 2021, 2(6), 1896–1926, 10.1039/D0MA00873G.
- S. Talebian, M. Mehrali, N. Taebnia, C. P. Pennisi, F. B. Kadumudi and J. Foroughi, et al., Self-Healing Hydrogels: The Next Paradigm Shift in Tissue Engineering?, Adv. Sci., 2019, 6(16), 1801664 CrossRef PubMed . Available from: https://pubmed.ncbi.nlm.nih.gov/31453048.
- Designed Research; A. SVP, Performed Research; A SVP, Pnas G. Rapid self-healing hydrogel, 2012;109(12):4383–4388.
- X. F. Cui, W. J. Zheng, W. Zou, X. Y. Liu, H. Yang and J. Yan, et al., Water-retaining, tough and self-healing hydrogels and their uses as fire-resistant materials, Polym. Chem., 2019, 10(37), 5151–5158 RSC.
- A. J. R. Amaral, V. M. Gaspar and J. F. Mano, Responsive laminarin-boronic acid self-healing hydrogels for biomedical applications, Polym. J., 2020, 52(8), 997–1006, DOI:10.1038/s41428-020-0348-3.
- S. U. Kadam, B. K. Tiwari and C. P. O’Donnell, Extraction, structure and biofunctional activities of laminarin from brown algae, Int. J. Food Sci. Technol., 2015, 50(1), 24–31 CrossRef CAS.
- B. Wang, L. Liu and L. Liao, Light and ferric ion responsive fluorochromic hydrogels with high strength and self-healing ability, Polym. Chem., 2019, 10(47), 6481–6488 RSC.
- R. Xu, S. Ma, P. Lin, B. Yu, F. Zhou and W. Liu, High Strength Astringent Hydrogels Using Protein as the Building Block for Physically Cross-linked Multi-Network, ACS Appl. Mater. Interfaces, 2018, 10(9), 7593–7601 CrossRef CAS PubMed.
- L. Zhou, L. Fan, X. Yi, Z. Zhou, C. Liu and R. Fu, et al., Soft Conducting Polymer Hydrogels Cross-Linked and Doped by Tannic Acid for Spinal Cord Injury Repair, ACS Nano, 2018, 12(11), 10957–10967 CrossRef CAS PubMed.
- C. Yu, D. Alkekhia and A. Shukla, β-Lactamase Responsive Supramolecular Hydrogels with Host–Guest Self-Healing Capability, ACS Appl. Polym. Mater., 2020, 2, 55–65 CrossRef CAS.
- J. Tang, M. U. Javaid, C. Pan, G. Yu, R. M. Berry and K. C. Tam, Self-healing stimuli-responsive cellulose nanocrystal hydrogels, Carbohydr. Polym., 2020, 229, 115486 CrossRef CAS PubMed.
- S. Palantöken, K. Bethke, V. Zivanovic, G. Kalinka, J. Kneipp and K. Rademann, Cellulose hydrogels physically crosslinked by glycine: Synthesis, characterization, thermal and mechanical properties, J. Appl. Polym. Sci., 2020, 137(7), 23–25 CrossRef.
- K. Ravishankar, M. Venkatesan, R. P. Desingh, A. Mahalingam, B. Sadhasivam and R. Subramaniyam, et al., Biocompatible hydrogels of chitosan-alkali lignin for potential wound healing applications, Mater. Sci. Eng., C, 2019, 102, 447–457 CrossRef CAS PubMed.
- W. Dai, H. Guo, B. Gao, M. Ruan, L. Xu and J. Wu, et al., Double network shape memory hydrogels activated by near-infrared with high mechanical toughness, nontoxicity, and 3D printability, Chem. Eng. J., 2019, 356, 934–949 CrossRef CAS.
- B. Gao, L. Chen, Y. Zhao, X. Yan, X. Wang and C. Zhou, et al., Methods to prepare dopamine/polydopamine modified alginate hydrogels and their special improved properties for drug delivery, Eur. Polym. J., 2019, 110, 192–201 CrossRef CAS.
- Y. Ren and J. Feng, Skin-Inspired Multifunctional Luminescent Hydrogel Containing Layered Rare-Earth Hydroxide with 3D Printability for Human Motion Sensing, ACS Appl. Mater. Interfaces, 2020, 12(6), 6797–6805, DOI:10.1021/acsami.9b17371.
- X. F. Sun, Y. Hao, Y. Cao and Q. Zeng, Superadsorbent hydrogel based on lignin and montmorillonite for Cu(II) ions removal from aqueous solution, Int. J. Biol. Macromol., 2019, 127, 511–519 CrossRef CAS PubMed.
- A. Taki, B. John, S. Arakawa and M. Okamoto, Structure and rheology of nanocomposite hydrogels composed of DNA and clay, Eur. Polym. J., 2013, 49(4), 923–931 CrossRef CAS.
- Reena, A. Kumar, V. Mahto and A. K. Choubey, Synthesis and characterization of cross-linked hydrogels using polyvinyl alcohol and polyvinyl pyrrolidone and their blend for water shut-off treatments, J. Mol. Liq., 2020, 301, 112472, DOI:10.1016/j.molliq.2020.112472.
- Khushbu, S. G. Warkar and A. Kumar, Synthesis and assessment of carboxymethyl tamarind kernel gum based novel superabsorbent hydrogels for agricultural applications, Polymer, 2019, 182, 121823, DOI:10.1016/j.polymer.2019.121823.
- L. Wu, G. Chen and Z. Li, Layered Rare-Earth Hydroxide/Polyacrylamide Nanocomposite Hydrogels with Highly Tunable Photoluminescence, Small, 2017, 13(23), 1604070 CrossRef PubMed.
- M. Yadollahi, H. Namazi and M. Aghazadeh, Antibacterial carboxymethyl cellulose/Ag nanocomposite hydrogels cross-linked with layered double hydroxides, Int. J. Biol. Macromol., 2015, 79, 269–277 CrossRef CAS PubMed.
|
This journal is © The Royal Society of Chemistry 2021 |
Click here to see how this site uses Cookies. View our privacy policy here.