DOI:
10.1039/D0MA00925C
(Communication)
Mater. Adv., 2021,
2, 937-941
Boron-vertex modification of carba-closo-dodecaborate for high-performance magnesium-ion battery electrolyte†
Received
25th November 2020
, Accepted 25th December 2020
First published on 26th January 2021
Abstract
Further advances in magnesium ion batteries (MIBs) require the development of electrolyte solutions with highly optimized properties. Here we report the design, synthesis and electrolytic properties of a new family of Mg salts of carba-closo-dodecaborate anions (CCAs). Detailed calculations suggested that modification at the boron vertices, previously considered inappropriate for MIB electrolytes, would be effective. Experimental studies showed that simple halogenation at the 12-B vertex of CCA drastically improves the solubility in low viscosity solvents without compromising the chemical and redox stability, and the ionic conductivity reached 6.2 mS/cm. The synthesized electrolytes showed low overpotential (≤200 mV) for Mg deposition and excellent reversible magnesium deposition/stripping with high Coulombic efficiency (≥95%).
Introduction
Multivalent-ion systems are promising candidates for high-energy-density storage to replace lithium ion batteries (LIBs).1 In this context, Mg ion batteries (MIBs) have attracted much attention because of the advantages of Mg metal, which include high volumetric capacity as an anode and high abundance in nature.2,3 Practical MIBs require high-voltage-capacity cathodes combined with Mg anodes,3 but so far their development has been limited by the lack of electrolytes compatible with these electrodes.3 Consequently, development efforts have been devoted to not only cathodes, but also electrolytes.3 In particular, Mg salts play an important role in the design of electrolytes, because they affect the solubility in various solvents,4 the behavior of solvated Mg ions,5 and the formation of the passivation layer on Mg anodes.6 Recent advances have led to the discovery of a new class of Mg salts with weakly coordinating anions (WCAs).7,8 Some electrolytes including WCAs afford qualitative reversibility of Mg deposition/stripping and excellent stability at Mg anodes.8 In particular, Mg salts based on carba-closo-dodecaborate anion HCB11H11− (denoted as CCA in this manuscript), one of the most stable WCAs (Fig. 1A), are considered promising electrolytes because of their chemical, redox, moisture and thermal stability and very low overpotential for Mg deposition.8c,d,9 Further, Persson and Zavadil et al. found that the introduction of an electron-withdrawing substituent at the C-vertex of CCA improves the oxidation stability.10 However, CCA Mg salts still suffer from low ion conductivity, due to their poor solubility in low viscosity solvents such as dimethoxyethane (DME) (Fig. 1B).8c,d,9,10
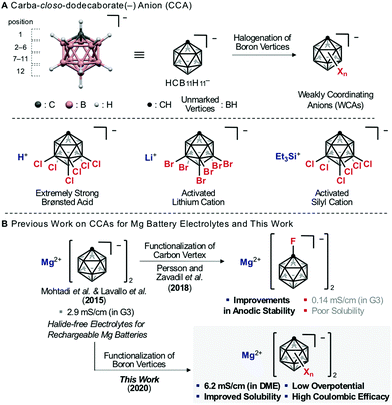 |
| Fig. 1 (A) The structure of carba-closo-dodecaborate anion (CCA). (B) Previous work on CCA Mg salts for Mg battery electrolytes and this work. | |
It has been experimentally established that halogenation of the B-vertices of CCA improves its weak coordinating ability and chemical stability by delocalizing and shielding the charge of the anion.11 Therefore, this strategy has been traditionally utilized for the creation of super (Brønsted) acids12 and highly activated cations (Fig. 1A).13 However, modification of the B-vertices of CCA has not been investigated for MIB electrolytes, probably because halogenation of the B-vertices is expected to significantly decrease the reductive stability.14 We wondered if this idea is correct. To address this question, we conducted theoretical and experimental studies on the effects of modification of the lower hemisphere (7–12-B vertices) of CCA on the solubility and conductivity.
Results and discussion
To obtain insight into the redox stability of B-substituted CCAs, we commenced our study by computing the electrochemical window at the B3LYP-D3(BJ)/6-311++G(d,p) level, relative to the Mg/Mg2+ redox pair set at 0.0 V (Fig. 2).15 The electron affinity (EA) and ionization potential (IP) were calculated under the adiabatic approximation, which accounts for orbital and geometric relaxation between charge states. The EA is the energy gained for a reduced anion, while IP represents the energy penalty to oxidize an anion. The calculation of HCB11H5Br6− (7–12-Br6-CCA), a commonly used WCA, indicated improved oxidative stability compared with CCA, to the same degree as FCB11H11− (1-F-CCA), whereas the reduction stability was significantly decreased, which is consistent with the conventional idea.14 Next, since electrophilic functionalization first occurs at the 12-B vertex, we computationally examined 12-mono-functionalized derivatives. Notably, DFT calculations showed that electronic perturbation at the 12-position of CCA has little or no effect on the cathodic stability. In contrast, the anodic stability depends markedly on the character of the substituent. The introduction of electron-withdrawing halogens at the 12-B vertex does not have a significant impact on the anodic stability, whereas electron-donating groups such as CH3 at the 12-position decrease the anodic stability. Oxidized and reduced states of the 12-iodinated compound HCB11H10I− (12-I-CCA) were decomposed during geometry optimization, implying that the B–I bond is unstable in the redox process.16
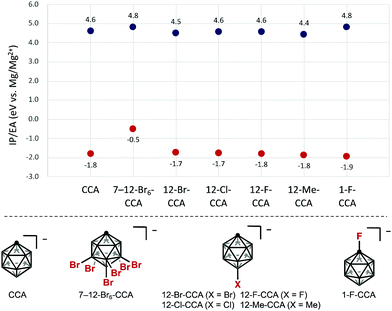 |
| Fig. 2 Calculated electrochemical windows (IP/EA) of different CCAs. All values are reported versus Mg/Mg2+. Red dots are EAs; blue dots are IPs. | |
To evaluate experimentally the effects of halogenations of the lower hemisphere, several CCAs other than 12-F-CCA, which requires extremely dangerous hydrogen fluoride for synthesis,17 were then synthesized as the tetrabutylammonium (Bu4N) salts.18 As expected from the DFT calculations, the 12-halogenated compounds HCB11H10Cl− (12-Cl-CCA) and HCB11H10Br− (12-Br-CCA) showed comparable oxidative stability to the parent CCA (Table 1). On the other hand, the oxidation stability of 7–12-Br6-CCA was significantly improved, but the reduction stability was significantly decreased.19 However, 12-Cl-CCA and 12-Br-CCA showed no reduction in the same way as the parent CCA, suggesting that compounds halogenated at the 12-B vertex are potentially attractive as novel electrolytes without reducing redox stability.
Table 1 Cyclic voltammetry of selected CCAs in HFIPa and MeCNb
Compound |
Oxidation (in HFIP) Epaa |
Reduction (in MeCN) Epcb |
The values are ordered by their cathodic peak potentials (CV value). All values [V] are referenced against the Fc0/+ redox couple. Oxidations of TBA-CCAs were performed with [nBu4N]+[PF6]− as a supporting electrolyte.
Reduction measurements of TBA-CCAs were performed with [nBu4N]+[ASF6]− as a supporting electrolyte.
No reduction was observed within the available range of the solvent/electrolyte system.
|
[Bu4N]+[CCA] |
2.08 |
—c |
[Bu4N]+[7–12-Br6-CCA] |
2.31 |
−2.85 |
[Bu4N]+[12-Br-CCA] |
2.03 |
—c |
[Bu4N]+[12-Cl-CCA] |
2.07 |
—c |
The solubility of various mono-functionalized CCAs was next examined and compared that of unsubstituted CCA. Mg salts of mono-functionalized CCAs were prepared using Persson and Zavadil's elegant “deprotonative magnesiation” protocol (Scheme 1).10 This method yields only butane (gas) and NR3 (R = Me, Et) as by-products, and we found that it could be used to synthesize pure Mg salts on a gram scale. The “cation exchange” method8c starting from the Ag salts of CCAs proved to be unsuitable for large-scale synthesis of high-purity samples.
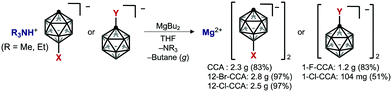 |
| Scheme 1 Synthesis of Mg salts of functionalized CCAs. | |
Since most Mg salts with WCAs coordinate with solvents such as DME or THF, we compared two kinds of unsubstituted compounds (Mg2+[CCA]2·DME and Mg2+[CCA]2·THF) which differ in the coordinating solvent (DME or THF), in order to exclude the effect of coordinating solvents on the solubility of Mg salts (Fig. 3A). Mg2+[CCA]2·DME and Mg2+[CCA]2·THF showed the similar solubility in DME (<0.1 M), indicating that the type of coordinating solvent has little effect. Thus, for further investigations, we adopted Mg salts containing THF as the coordinating solvent. The 1(C-vertex)-fluorinated compound Mg2+[1-F-CCA]2, which was reported to show improved oxidative stability,10 was practically insoluble in DME (<0.1 M), and the 1-chlorinated compound Mg2+[1-Cl-CCA]2 showed low solubility in DME (∼0.3 M). In contrast, 12-B vertex halogenated compounds Mg2+[12-Cl-CCA]2 and Mg2+[12-Br-CCA]2 exhibited high solubility in DME (∼1 M). Thus, the introduction of halogen at the 12-B vertex markedly improves the solubility. In terms of cation-negative charge interaction, the Coulombic attraction between a +1 point charge placed 2.5 Å from the hydrogen atom of a vertex on the B–H/C–H axis and the natural atomic charge in the anion increases in the order 1-C ≪ 2–6-B < 7–11-B < 12-B positions, as shown by Michl et al., for unperturbed CCA.20 Therefore, substitution of the hydrogen atom with an electronegative, lower charge density, sterically shielding, and lipophilic halogen (Br or Cl) at the 12-B vertex should inhibit the interaction between CCA and the Mg2+ cation center more effectively than substitution at the 1-C vertex, and this is presumably the reason for the remarkably improved solubility of Mg2+[12-Cl-CCA]2 and Mg2+[12-Br-CCA]2. Thus, based on the computational and fundamental physicochemical studies, we found that simple halogenation at 12-B vertex is an effective approach to increase solubility without decreasing redox stability.
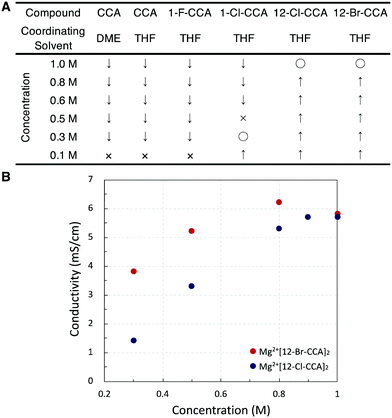 |
| Fig. 3 (A) Dissolution behavior of CCA derivatives in DME (○: soluble, ×: insoluble). (B) Ionic conductivity measurements of Mg2+[12-Cl-CCA]2 in DME and Mg2+[12-Br-CCA]2 in DME at various salt concentrations. | |
The ionic conductivities of Mg2+[12-X-CCA]2 (X = Cl and Br) at various concentrations in DME at room temperature were then measured (Fig. 3B). The ionic conductivities increased with increasing concentration, and reached maximum values at around 1.0 M for Mg2+[12-Cl-CCA]2 in DME (5.6 mS/cm) and 0.8 M for Mg2+[12-Br-CCA]2 in DME (6.2 mS/cm). These values are higher than those of conventional CCA-based Mg2+ salts, such as 0.65–0.85 M Mg2+[CCA]2 in G3 (2.9 mS/cm) and 60 mM Mg2+[1-F-CCA]2 in G3 (0.14 mS/cm).8c,10 The reason for this is that the solubility in low viscosity solvent is drastically improved by the substitution at the 12-position.
Finally, we measured the electrochemical properties of Mg2+[12-X-CCA]2 (X = Cl and Br) to examine the compatibility of these electrolyte solutions with Mg anodes. The cyclic voltammograms are shown in Fig. 4A. They exhibited excellent Mg deposition with very low overpotential (≤200 mV) and high Coulombic efficiency (CE): CE of more than 95% was maintained for at least 100 cycles (Fig. 4B). The reduced product of Mg2+[12-Br-CCA]2 on a Pt disk working electrode was also analyzed by means of SEM, and energy dispersive X-ray spectroscopy (EDS) mapping indicated that the product is pure Mg metal (Fig. 4C). Furthermore, the absence of B, C, and Br supports the high redox and chemical stability of the 12-X-CCA skeleton. Overall, the results demonstrate good compatibility of these electrolyte solutions with Mg anodes.
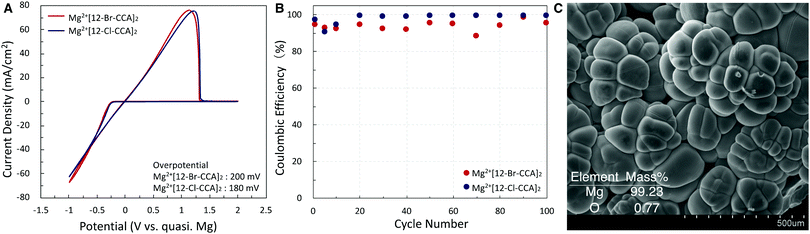 |
| Fig. 4 (A) Cyclic voltammograms of 1.0 M Mg2+[12-Cl-CCA]2 and 0.8 M Mg2+[12-Br-CCA]2 in DME using Pt as the working electrode and Mg as the reference at a scan rate of 100 mV/s. (B) Cycling behavior of Coulombic efficiency. (C) Scanning electron microscopy (SEM) image of Mg deposited on the Pt substrate from Mg2+[12-Br-CCA]2 in DME. | |
Conclusions
In summary, our combined computational and experimental studies demonstrate that functionalization of the lower hemisphere of the CCA skeleton with an appropriate choice of elements/functional groups can improve the electrochemical and physicochemical properties, such as conductivity and solubility. In particular, simple halogenation at the 12-B vertex of CCA dramatically improves the solubility in low viscosity solvents without compromising redox stability. Mg2+[12-Cl-CCA]2 and Mg2+[12-Br-CCA]2 showed excellent reversible magnesium deposition/stripping, with high Coulombic efficiency (≥95%).
Given that CCA is readily customizable, we anticipate that CCAs will be an excellent platform for the development of electrolytes for MIBs. Further studies to develop a higher performance CCA-based electrolyte by means of combined functionalizations of the C and B vertices are ongoing in our laboratory.
Conflicts of interest
There are no conflicts to declare.
Acknowledgements
This work was partly supported by JSPS KAKENHI (S) (No. 17H06173) (to M. U.), JST CREST (No. JPMJCR19R2) (to M. U.). Foundation NAGASE Science Technology Development, Sumitomo Foundation (to M. U.), JSPS KAKENHI (No. 19K23621 and 20K15950) (to J. K.), and JSPS KAKENHI (B) (No. 17H03017) (to K. M.). A generous allotment of computational resources (Projects G19012) from HOKUSAI GreatWave (RIKEN) is gratefully acknowledged. We are grateful to Prof. Dr Mutsumi Kimura for helpful discussion.
Notes and references
- A. Ponrouch, J. Bitenc, R. Dominko, N. Lindahl, P. Johansson and M. R. Palacin, Energy Storage Mater., 2019, 20, 253 CrossRef.
- D. Aurbach, Z. Lu, A. Schechter, Y. Gofer, H. Gizbar, R. Turgeman, Y. Cohen, M. Moshkovich and E. Levi, Nature, 2000, 407, 724 CrossRef CAS.
- Z. Ma, D. R. MacFarlane and M. Kar, Batteries Supercaps, 2019, 2, 115 CrossRef.
- M. Salama, I. Shterenberg, H. Gizbar, N. N. Eliaz, M. Kosa, K. Keinan-Adamsky, M. Afri, L. J. W. Shimon, H. E. Gottlieb, D. T. Major, Y. Gofer and D. Aurbach, J. Phys. Chem. C, 2016, 120, 19586 CrossRef CAS.
-
(a) L. F. Wan and D. Prendergast, J. Am. Chem. Soc., 2014, 136, 14456 CrossRef CAS;
(b) T. Kimura, K. Fujii, Y. Sato, M. Morita and N. Yoshimoto, J. Phys. Chem. C, 2015, 119, 18911 CrossRef CAS.
-
(a) Z. Lu, A. Schechter, M. Moshkovich and D. Aurbach, J. Electroanal. Chem., 1999, 46, 203 CrossRef;
(b) R. Jay, A. W. Tomich, J. Zhang, Y. Zhao, A. D. Gorostiza, V. Lavallo and J. Guo, Appl. Mater. Interfaces, 2019, 11, 11414 CrossRef CAS.
- E. N. Keyzer, H. F. J. Glass, Z. L. Liu, P. M. Bayley, S. E. Dutton, C. P. Grey and D. S. Wright, J. Am. Chem. Soc., 2016, 138, 8682 CrossRef CAS.
-
(a) Y. Shao, T. Liu, G. Li, M. Gu, Z. Nie, M. Engelhard, J. Xiao, D. Lv, C. Wang, J. G. Zhang and J. Liu, Sci. Rep., 2013, 3, 3130 CrossRef;
(b) T. J. Carter, R. Mohtadi, T. S. Arthur, F. Mizuno, R. Zhang, S. Shirai and J. W. Kampf, Angew. Chem., Int. Ed., 2014, 53, 3173 CrossRef CAS;
(c) O. Tutusaus, R. Mohtadi, T. S. Arthur, F. Mizuno, E. G. Nelson and Y. V. Sevryugina, Angew. Chem., Int. Ed., 2015, 54, 7900 CrossRef CAS;
(d) S. G. McArthur, L. X. Geng, J. C. Guo and V. Lavallo, Inorg. Chem. Front., 2015, 2, 1101 RSC;
(e) J. T. Herb, C. A. Nist-Lund and C. B. Arnold, ACS Energy Lett., 2016, 1, 1227 CrossRef CAS;
(f) Z. Zhao-Karger, M. E. G. Bardaji, O. Fuhr and M. Fichtner, J. Mater. Chem. A, 2017, 5, 10815 RSC;
(g) E. N. Keyzer, J. Lee, Z. Liu, A. D. Bond, D. S. Wright and C. P. Grey, J. Mater. Chem. A, 2019, 7, 2677 RSC;
(h) K. C. Lau, T. J. Seguin, E. V. Carino, N. T. Hahn, J. G. Connell, B. J. Ingram, K. A. Persson, K. R. Zavadil and C. Liao, J. Electrochem. Soc., 2019, 166, A1510 CrossRef CAS;
(i) J. Luo, Y. Bi, L. Zhang, X. Zhang and T. L. Liu, Angew. Chem., Int. Ed., 2019, 58, 6967 CrossRef CAS.
- For reviews, see:
(a) S. P. Fisher, A. W. Tomich, S. O. Lovera, J. F. Kleinsasser, J. Guo, M. J. Asay, H. M. Nelson and V. Lavallo, Chem. Rev., 2019, 119, 8262 CrossRef CAS;
(b) S. P. Fisher, A. Tomich, J. Guo and V. Lavallo, Chem. Commun., 2019, 55, 1684 RSC;
(c) B. Ringstrand and P. Kaszynski, Acc. Chem. Res., 2013, 46, 214 CrossRef CAS.
- N. T. Hahn, T. J. Seguin, K. C. Lau, C. Liao, B. J. Ingram, K. A. Persson and K. R. Zavadil, J. Am. Chem. Soc., 2018, 140, 11076 CrossRef CAS.
- For recent reviews on CCAs, see:
(a) C. Douvris and J. Michl, Chem. Rev., 2013, 113 Search PubMed , PR179;
(b)
R. N. Grimes, Carboranes, Elsevier, Amsterdam, 3rd edn, 2016 Search PubMed;
(c)
N. S. Hosmane, Boron Science: New Technologies and Applications, Taylor & Francis Books/CRC, Boca Raton, FL, 2011 Search PubMed;
(d) R. N. Grimes, Dalton Trans., 2015, 44, 5939 RSC;
(e) J. Kanazawa, Y. Kitazawa and M. Uchiyama, Chem. – Eur. J., 2019, 25, 9123 CrossRef CAS.
- For reviews, see:
(a) C. A. Reed, Chem. Commun., 2005, 1669 RSC;
(b) C. A. Reed, Acc. Chem. Res., 2010, 43, 121 CrossRef CAS;
(c) C. A. Reed, Acc. Chem. Res., 2013, 46, 2567 CrossRef CAS;
(d)
C. Knapp, Weakly coordinating anions: Halogenated borates and dodecaborates, in Comprehensive Inorganic Chemistry II, Elsevier, Amsterdam, 2013, vol. 1, p. 651 Search PubMed;
(e) I. M. Riddlestone, A. Kraft, J. Schaefer and I. Krossing, Angew. Chem., Int. Ed., 2018, 57, 13982 CrossRef CAS For selected examples of super (Brønsted) acid, see: ;
(f) M. Nava, I. V. Stoyanova, S. Cummings, E. S. Stoyanov and C. A. Reed, Angew. Chem., Int. Ed., 2014, 53, 1131 CrossRef CAS.
-
(a) S. Moss, B. T. King, A. de Meijere, S. I. Kozhushkov, P. E. Eaton and J. Michl, Org. Lett., 2001, 3, 2375 CrossRef CAS;
(b) V. Volkis, C. Douvris and J. Michl, J. Am. Chem. Soc., 2011, 133, 7801 CrossRef CAS;
(c) Y. Kitazawa, R. Takita, K. Yoshida, A. Muranaka, S. Matsubara and M. Uchiyama, J. Org. Chem., 2017, 82, 1931 CrossRef CAS;
(d) C. Douvris and O. V. Ozerov, Science, 2008, 321, 1188 CrossRef CAS;
(e) O. Allemann, S. Duttwyler, K. K. Baldridge and J. S. Siegel, Science, 2011, 332, 574 CrossRef CAS;
(f) B. Shao, A. L. Bagdasarian, S. Popov and H. M. Nelson, Science, 2017, 355, 1403 CrossRef CAS;
(g) S. Popov, B. Shao, A. L. Bagdasarian, T. R. Benton, L. Zou, Z. yang, K. N. Houk and H. M. Nelson, Science, 2018, 361, 381 CrossRef CAS.
- In ref. 10, the authors stated “Furthermore, selective derivatization of B sites on the HCB11H11− cage has been demonstrated to significantly increase the oxidative stability of the anion, although at the cost of decreased reductive stability in every case.” For further evidence supporting this idea, see:
(a) R. T. Boere, C. Bolli, M. Finze, A. Himmelspach, C. Knapp and T. L. Roemmele, Chem. – Eur. J., 2013, 19, 1784 CrossRef CAS;
(b) A. Wahab, C. Douvris, J. Klima, F. Sembera, J. Ugolotti, J. Kaleta, J. Ludvik and J. Michl, Inorg. Chem., 2017, 56, 269 CrossRef CAS.
-
(a) J. A. Pople and L. A. Curtiss, J. Phys. Chem., 1987, 91, 155 CrossRef CAS;
(b) S. P. Ong and G. Ceder, Electrochim. Acta, 2010, 55, 3804 CrossRef CAS;
(c) S. P. Ong, O. Andreussi, Y. Wu, N. Marzari and G. Ceder, Chem. Mater., 2011, 23, 2979 CrossRef CAS;
(d) X. H. Qu, A. Jain, N. N. Rajput, L. Cheng, Y. Zhang, S. P. Ong, M. Brafman, E. Maginn, L. A. Curtiss and K. A. Persson, Comput. Mater. Sci., 2015, 103, 56 CrossRef CAS;
(e) N. N. Rajput, X. Qu, N. Sa, A. K. Burrell and K. A. Persson, J. Am. Chem. Soc., 2015, 137, 3411 CrossRef CAS.
- In ref. 14a and 14b, 7–12 or 2–12 iodinated CCAs were found to have much lower oxidation and reduction stability than the corresponding brominated and chlorinated CCAs.
- S. V. Ivanov, A. J. Lupinetti, S. M. Miller, O. P. Anderson, K. A. Solntsev and S. H. Strauss, Inorg. Chem., 1995, 34, 6419 CrossRef CAS.
- The anodic stability of Mg2+ salt of the CCA electrolytes was studied by linear sweep voltammetry using aluminum electrode. The oxidation current was observed at 3.5 V, which corresponds to glyme solvent decomposition. Therefore, to evaluate the oxidation stability of CCAs, we measured oxidation stability of CCAs in HFIP. For details, see ESI.†.
- No obvious Mg deposition and stripping peaks were detected from Mg2+[7–12-Br6-CCA]2, see ESI.†.
- I. Zharov, T. Weng, A. Orendt, D. Barich, J. Penner-Hahn, D. Grant, Z. Havlas and J. Michl, J. Am. Chem. Soc., 2004, 126, 12033 CrossRef CAS.
Footnote |
† Electronic supplementary information (ESI) available. See DOI: 10.1039/d0ma00925c |
|
This journal is © The Royal Society of Chemistry 2021 |