DOI:
10.1039/D0LC00855A
(Paper)
Lab Chip, 2021,
21, 154-162
A semi-dry chemistry hydrogel-based smart biosensing platform for on-site detection of metal ions†
Received
21st August 2020
, Accepted 9th November 2020
First published on 24th November 2020
Abstract
Balancing operability and performance has long been a focus of research in bioanalysis and biosensing. In this work, between the traditional wet chemistry and dry chemistry, we develop a semi-dry smart biosensing platform with favourable operability and performance for metal ions detection. This platform is based on the integration of a stimuli-responsive hydrogel with intelligent image recognition. The hydrogel consists of agarose as a matrix and well-designed fluorescent DNA probes as response elements. Target metal ions in a test sample can diffuse into the hydrogel and activate the DNA probes, outputting fluorescence signals for intelligent imaging. In this way, sensitive and convenient detection of metal ions such as potassium ions (K+) and mercury ions (Hg2+) can be achieved without the assistance of huge instruments and professional workers. The detection limits for K+ and Hg2+ are 0.34 mM and 5.6 nM, respectively. Detection of ions in serum and lake water is also available. Moreover, the hydrogel-based biosensing platform exhibits favorable selectivity, anti-degradation ability, and long-term stability. High-throughput testing can be also achieved by punching multiple test microwells in a single piece of hydrogel. The concept and successful practice of a semi-dry chemistry-based strategy make up for the shortcomings of wet chemistry and dry chemistry, and provide a promising approach for on-site testing.
Introduction
At present, various bioanalytical and biosensing technologies have become the cornerstones of disease diagnosis, environmental monitoring, and food safety.1–3 These techniques can be roughly divided into two categories in terms of operation: wet chemistry and dry chemistry.4,5 For the former, one or more solutions are usually incubated successively to allow the analyte to react with the reagents and produce a readable signal as a result.6 For the latter, all the reagent materials are usually fixed on a solid matrix, e.g. paper, nitrocellulose membrane and chip, so as to achieve ready-to-use detection of the target and to output generally a visual signal.7–10 Both of these two categories have advantages and disadvantages. Wet chemistry represented by enzyme-linked immunosorbent assay (ELISA), polymerase chain reaction (PCR), etc. is highly sensitive and has good performance of quantitative analysis.11,12 But due to the complicated operation, professional technicians are generally required. For example, Yang's group designed a series of bar chart chips for visual quantitative detection, but the fabrication of skillful bar chart chips required professional personnel.13,14 Dry chemistry represented by test strips eliminates the inconvenience of carrying and handling liquid substances, and is more friendly to operate especially for on-site testing and at-home testing.13 For example, Kumari's group fabricated coumarin coated fluorescent strips for visual detection of Cd2+.15 Ma's group developed a quantum-dot-based test strip for simultaneous determination of different biomarkers.16 But the performance for the accurate quantitative analysis of the target is often unsatisfactory.
How to balance the operability and performance of quantitative analysis is the long-term topic for scientific researchers, which is particularly important for on-site testing that requires accurate quantification.17 To address this issue, we propose an interesting concept here: semi-dry chemistry by seeking answers by using a hydrogel. As an intermediate state between liquid and solid, a hydrogel has the dual characteristics of both.18,19 It can maintain a certain shape under specific conditions just like solids, which provides the possibility of convenient liquid-free analysis. In parallel, it can also allow reagents to diffuse and react just like that in liquids, which provides the possibility of advanced quantitative analysis.20 There have been many reports on the use of hydrogels for biochemical analysis and biosensing. For instance, Tan's group developed aptamer-crosslinked hydrogels, which can be activated by different chemical stimuli of cocaine and ATP.21 Ju et al. designed an aptamer-functionalized hydrogel encapsulating gold nanoparticles for protein detection.22 These works have made useful attempts to solve the above problems of wet chemistry and dry chemistry, but there is still huge room for improvement in the detection performance and operability.23
In this work, we develop a semi-dry detection system based on a stimuli-responsive hydrogel and intelligent image recognition, which incorporates the merits of quantitative analysis and is ready-to-use. The semi-dry hydrogel is fabricated by embedding DNA probes and specific fluorescent dyes in an agarose hydrogel. When ions are injected into the microwells of the hydrogel, they can trigger the conformation changes of the DNA probes and thereafter output fluorescence signals, which can be imaged with a digital camera or a gel imaging system under ultraviolet irradiation. The obtained images can be subsequently analyzed by an intelligent image recognition program using a tablet computer. The system has the following characteristics: 1) in terms of operability, the DNA incorporating agarose hydrogel can be used as both a reaction container and a reaction substrate. No other reagents are needed. So, metal ions can be detected in a convenient liquid-free way. 2) In terms of quantitative detection, we use intelligent image recognition technology to intelligently analyze the detection results, so that we can directly present quantitative data. 3) For high-throughput detection, multiple microwells can be punched in a single piece of hydrogel, and each microwell can be adopted to perform an independent assay, thereby enabling high-throughput analysis. With these advantages, this semi-dry chemistry-based smart biosensing platform not only paves the way to on-site assay of metal ions, but also offers new possibilities to adapt the hydrogel for environmental monitoring, personalized healthcare and point-of-care diagnostics.
Materials and methods
Chemicals and reagents
All the oligonucleotides (K+-sensitive DNA probe: CGC GGG TTT TGG GTT TTG GGT TTT GGG G; Hg2+-sensitive DNA probe: TTG GTT TGT TTT CTT TCG TAT GCT TTG T-(BHQ1)-TT TCT TTC CTT AGT GTA ATC AAG GAA AGA AA-(FAM)) were synthesized and purified by TaKaRa Biotechnology Co. Ltd. Agarose was purchased from Saiguo Biotechnology Co. Ltd. (Guangzhou, China). Mercuric chloride (HgCl2) and N-methylmesoporphyrin (NMM) were obtained from J&K Technology Co. Ltd. (Beijing, China). Tris-HCl buffer (200 mM, pH 7.4) was used for dissolving DNA and preparing the hydrogel. Water used throughout all experiments was purified with a Milli-Q system (Branstead, USA) to a specific resistance of >18 MΩ cm−1.
Fluorescence spectra were recorded on a F4500 spectrofluorophotometer (HITACHI, Japan). Fluorescence images under ultraviolet (UV) irradiation were recorded with a digital camera or a gel imaging system (SYSTEM GelDoc XR+ 1
708
195, Bio-Rad, USA). Electrochemical analysis of ions was performed on a potentiometer (model 7.1 pH/ion meter, Metrohm, Switzerland) equipped with an ion-selective electrode (ISE) and an Ag/AgCl reference electrode.
Preparation of the DNA incorporating agarose hydrogel
The preparation process for the DNA incorporating agarose hydrogel for the detection of K+ is as follows (Fig. S1†). First, 1.6% agarose solution was obtained by adding agarose powder to Tris-HCl buffer (pH = 7.4) in a beaker. Then, 2 μM K+-sensitive DNA probe and 50 μM NMM were added to the above agarose solution. After thorough mixing, the mixture was heated in a microwave oven to form a hydrosol, which was further poured into a round glass dish with a diameter of 5.6 cm. Then, a 3D printed cylindrical plastic mould with the shape shown in Scheme 1A was inserted into the hydrosol. The height and diameter of the mould are 12 mm and 6 mm, respectively. After cooling down at room temperature and removing the mould, a DNA incorporating agarose hydrogel with uniform microwells was prepared.
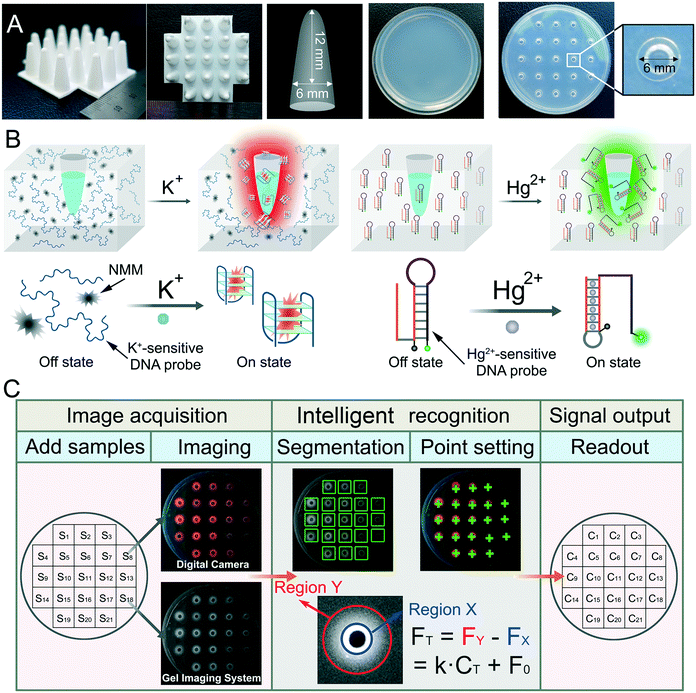 |
| Scheme 1 Principle of the DNA incorporating agarose hydrogel-based detection of metal ions. (A) Prepared DNA incorporating agarose hydrogel and the corresponding gel preparation mould. (B) Schematic illustration of the DNA incorporating agarose hydrogel-based detection of K+ and Hg2+. (C) Principle of intelligent analysis based on intelligent image recognition technology. | |
For the detection of Hg2+, agarose powder was firstly dissolved in 50 mM Tris-HCl (0.5 M NaCl, pH 7.5) to obtain a 1.6% (w/v) agarose solution. After heating in a microwave oven, the agarose solution was then slowly cooled to 50 °C. Next, 2 μM Hg2+-sensitive DNA probe was added and mixed with the agarose solution thoroughly. The mixture was further poured into a round glass dish, and the subsequent process is the same as that of the K+ detection above.
Detection of metal ions
For the detection of K+, 15 μL KCl solution with a concentration varying from 0.05 mM to 50 mM was injected into the microwell of the DNA incorporating agarose hydrogel at room temperature. After an incubation of 90 min, the sample-containing hydrogel was placed under UV light to obtain the fluorescence signals of the target potassium. Fluorescence images of the DNA incorporating agarose hydrogel were recorded with a digital camera or a gel imaging system.
For the detection of Hg2+, 50 μL HgCl2 solution with a concentration varying from 0.25 nM to 2.5 μM was injected into the microwell of the DNA incorporating agarose hydrogel at room temperature for 90 min. Next, the sample-containing hydrogel was placed under UV light to obtain the fluorescence signals. Fluorescence images of the DNA incorporating agarose hydrogel were recorded with a gel imaging system.
Intelligent image recognition
The images of the DNA incorporating agarose hydrogel were analyzed by either ImageJ, a common imaging analysis software, or a self-written intelligent image recognition program. For the former, the process is depicted in Fig. S2.† In detail, after opening the ImageJ software, select 8-bit as the image type and click process-subtract-background. Then, click “elliptical selection”. Next, a fixed circular area with a diameter of 0.06 inch containing the entire fluorescence signal (outer ring, Y) was selected by using the elliptical tool. Afterward, a fixed circular area with a diameter of 0.02 inch was selected as the inner ring (X). Then, click “analyze-measure” to calculate the integrated density (IntDen). The IntDen of X (FX) subtracted from the IntDen of Y (FY) was finally regarded as the fluorescence intensity signal (intensity) for the detection.
For the intelligent image recognition, a MATLAB-based GUI man–machine interface program was developed based on machine learning algorithm and image processing technology, denoted as GMIS. The fluorescence images were firstly transmitted and analyzed by the software GMIS. In less than 2 min, the results could be transmitted back and displayed on the visual interface of GMIS. The principle and operation process of GMIS are as follows: a linear equation and linear range of the concentration versus fluorescence intensity obtained from high-resolution images was first uploaded to GMIS. Subsequently, a high-resolution image of the target samples was uploaded and read by GMIS. GMIS segmented and classified different regions to identify microwells with fluorescence signals, then automatically positioned fluorescence measurement points (marked with green crosshairs) on the DNA incorporating agarose hydrogel. In this way, GMIS could analyze the fluorescence intensity of each microwell, and further automatically calculate the concentration according to the linear equation. When the calculated concentration is below the limit of detection, the output would be “<LOD”.
Detection of metal ions in real samples
Lake water samples were obtained from the campus lake in Shanghai University (SHU) and Shuanglong lake in Anqing Normal University (AQNU), respectively. Human serum samples were supplied by Navy Anqing Hospital.
The corresponding concentration of each sample was calculated though the calibration curve, and was compared with that obtained with an ISE.
Results and discussion
Principle of the DNA incorporating agarose hydrogel-based semi-dry chemistry
As shown in Scheme 1A, a semi-dry hydrogel was fabricated from an agarose–DNA mixture, and a corresponding loaded microwell was prepared through a 3D printed 21-well mould. Subsequently, the prepared DNA incorporating agarose hydrogel with microwells can serve as a sensing platform for the detection of metal ions. Here, K+ was selected as a model to test the performance of the sensing platform. As is known, K+ serves as an important element in the human body, and abnormal fluctuations of K+ is closely related to the abnormal functions of the heart, nervous system and cardiovascular system.15 For the detection of K+, a G-rich DNA probe and a specific fluorescent dye N-methylmesoporphyrin (NMM) are incorporated in the network of the agarose hydrogel (Scheme 1B). The G-rich DNA probe can chelate K+ to form a G-quadruplex structure,24 and once the G-quadruplex is formed, it can further combine with anionic porphyrin NMM to exhibit intensive red fluorescence under UV irradiation.25 In contrast, when K+ is absent, the G-quadruplex structure will not form with NMM incorporation and the fluorescence will not be generated. So, when a sample containing K+ is injected into the microwell, K+ will enter the hydrogel based on the free diffusion and hygroscopicity of the hydrogel, thereafter triggering the binding of NMM to the G-rich DNA probe, outputting fluorescence signals. Besides, Hg2+, a pollutant that is of great concern in environmental pollution, was selected as another model metal ion. The biosensing strategy developed according to the characteristics of Hg2+ is somewhat different from that of K+ detection. As illustrated in Scheme 1B, a Hg2+-sensitive DNA probe with a hairpin structure is designed to respond to Hg2+. There is a T-rich region at the 5-terminal of the DNA probe, which can bind another T-rich oligonucleotide that is blocked in the stem of the hairpin structure by forming T–Hg2+–T hybridization under the mediation of Hg2+. Once T–Hg2+–T is formed, reconfiguration of the DNA probe is launched, resulting in the departure of the quencher from the fluorophore and subsequently, the recovery of the fluorescence. Just like the K+ detection system, here the DNA probe is incorporated in the DNA incorporating agarose hydrogel. As for the quantification of metal ions, as shown in Scheme 1C, the fluorescence image of the semi-dry hydrogel is first recorded with a digital camera or a gel imaging system, and then intelligently analyzed using intelligent image recognition technology. Since that the intensity of the fluorescence should have a positive relationship with the concentration of the target metal ions, on-site and quantitative detection of metal ions without the requirement of any other reagents and devices is expected to be achieved. Notably, DNA incorporated agarose works as a substrate and reaction container, providing a convenient add-to-read manner for metal ion analysis by combining sample injection, reaction and indication together.
Feasibility of the fluorescence detection of potassium ions
The response of the G-rich DNA probe and NMM to K+ was first studied in a solution system. As shown in Fig. S3A,† a remarkable fluorescence peak at 620 nm can be observed in the presence of K+, whereas control groups NMM alone or NMM with the DNA probe show tiny fluorescence peaks. The difference can be easily distinguished even with the naked eye, suggesting the desirable recognition ability of the DNA probe for K+. Furthermore, some other common metal ions have not been found to induce the generation of fluorescence because they are not conducive to the formation of a DNA–NMM complex (Fig. S3B†), laying the foundation for selective analysis. There is also a positive correlation between the fluorescence intensity and the concentration of K+ (Fig. S3C†). As shown in Fig. S3D,† the fluorescence intensity shows good linear fitting (R2 = 0.99) with the concentration of K+ (0.025–2.5 mM), which lays the foundation for quantitative analysis. Finally, the study on the stability of the fluorescence signal indicates that the fluorescence remains stable for least 120 min (Fig. S4†), which enables delayed data processing and verification.
Optimization of the detection conditions in the DNA incorporating agarose hydrogel-based quantification
We next transferred the above fluorescence detection system to the DNA incorporating agarose hydrogel and optimized corresponding experimental conditions, including reaction time (Fig. S5†), the concentration of agar (Fig. S5†), buffer solution (Fig. S6†), pH value (Fig. S7†), and the concentration of the DNA probe (Fig. S8†). Optimized conditions were thus obtained with 1.6% agar, 90 min reaction time, 200 mM Tris-HCl, and 2.0 μM DNA probe. A maximum fluorescence peak could be obviously observed at pH 7.4, which was selected for the following experiments. Under optimized conditions, rough detection of standard K+ solution by using the DNA incorporating agarose hydrogel is achieved. As shown in Fig. 1A, B and E, clear fluorescent rings around the microwells can be observed in the presence of K+-containing samples, suggesting that K+ has diffused into the hydrogel and successfully triggered the formation of the G-quadruplex and DNA–NMM complexes. The formation of fluorescent rings is considered reasonable, because the relatively large DNA probes incorporated in the agarose hydrogel is difficult to leak out of the hydrogel for there is no driving forces, and at the same time, the hydrogel will suck the solution into the gel, resulting in no solution in the microwells. Owing to the formation of fluorescent rings, subsequent analysis can be carried out. After acquiring the fluorescence images, manual analysis with ImageJ (Fig. S2†) and intelligent analysis with GMIS are carried out (Fig. 1C, D, F and G).
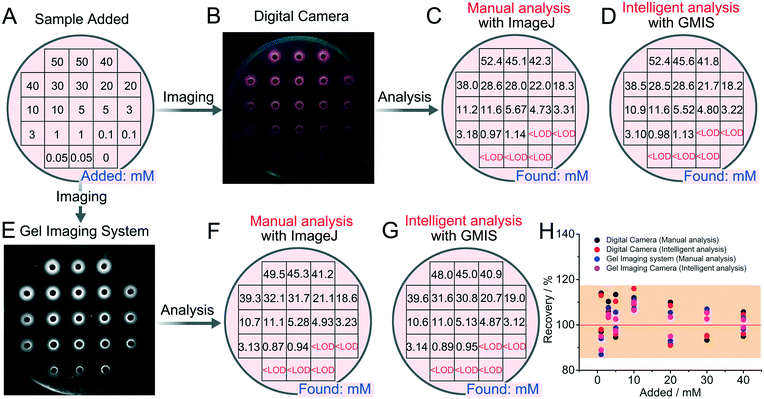 |
| Fig. 1 Optimization of the detection methods by using the DNA incorporating agarose hydrogel. (A) The concentration of K+ (mM) for each microwell. (B) Photographic image of the DNA incorporating agarose hydrogel obtained with a digital camera of a tablet computer. The recovery concentration K+ (mM) for each microwell obtained by (C) manual analysis and (D) intelligent analysis. (E) Photographic image of the DNA incorporating agarose hydrogel obtained with the gel imaging system. The recovery concentration of K+ (mM) for each microwell obtained by (F) manual analysis and (G) intelligent analysis. (H) Recovery rate analysis of C, D, F and G in the linear range of this method (1 mM to 50 mM). | |
As shown in Fig. 1C and D, the concentration of K+ intelligently calculated by ImageJ or GMIS is close to the concentration of K+ added (Fig. 1A), demonstrating the feasibility of the semi-dry chemistry-based smart biosensing platform for K+ detection. Through manual analysis, a good linear relationship between the concentration of K+ varying from 1 mM to 50 mM and fluorescence intensity is obtained both in the images acquired with the digital camera and gel imaging system (Fig. S9†). In terms of imaging methods, although the linear range is the same, the gel imaging system is more sensitive, manifesting a stronger fluorescence intensity and greater slope. Besides, the data obtained with the gel imaging system is more stable, manifesting a smaller deviation. The limit of detection (LOD) of this strategy is calculated to be 0.34 mM, which is even superior to some existing methods based on G-quadruplex (Table S1†). In terms of quantitative analysis, the results of intelligent analysis are consistent with those of manual analysis and the deviation is within 20% (Fig. 1H), indicating that manual analysis can be replaced by intelligent analysis to simplify the quantitative process. The above results demonstrate that the semi-dry hydrogel can be used for further intelligent quantification of K+. Importantly, under the assistance of intelligent image recognition technology, satisfactory quantitative results can be acquired without the assistance of huge instruments and professional workers.
Selectivity, anti-degradation and long-term storage of the DNA incorporating agarose hydrogel
The specificity for K+ assay by using the DNA incorporating agarose hydrogel was next studied (Fig. 2A–C). Some common ions in the biological matrix, including NH4+, Na+, Ca2+, Mg2+ and Fe3+, were adopted as controls. Their concentration was fixed at 250 mM, ten times the concentration of K+ (25 mM). The remarkable fluorescence change only appears in the presence of K+. In contrast, other control groups display almost negligible signal change on the DNA incorporated agarose plate, suggesting a good detection specificity. It is worth noting here that though Na+ can affect the detection in a liquid system to a certain extent (Fig. S10†), it barely affects the detection in our hydrogel-based system (Fig. 2A), which should be ascribed to the rapid diffusion of Na+ in the hydrogel and the confined position of the fluorescent probes. To further examine the selectivity of this method, the binary-based competitive system “K+ + metal ion” and a mixture of matrices “K+ + multi-metal ions” were investigated. As displayed in Fig. 2B and C, the interference of other metal ions could not show a strong influence on the fluorescence signal, suggesting a good selectivity of the detection system. Notably, nucleic acid-based biosensing systems always suffer from the problem of degradation by a nuclease. Protecting nucleic acids from enzymatic degradation is an important topic for practical applications.26 Since nucleic acids can be easily degraded by exonuclease I, the detection system cannot work anymore in solution. But in the case of the DNA incorporating agarose hydrogel, the detection is barely affected even if the hydrogel was immersed in a solution of exonuclease I for 1 day. The superior anti-degradation ability of the DNA incorporating agarose hydrogel is desirable and reasonable, because the pores of the hydrogel are not large enough to allow the free diffusion of enzymes with a large molecular weight. The detection stability of the DNA incorporating agarose hydrogel was further studied by conducting the detection after the storage of the DNA incorporating agarose hydrogel for a long time (30 days). Compared to the freshly prepared DNA incorporating agarose hydrogel, the signal intensity obtained from the DNA incorporating agarose hydrogel after long-term storage (30 day) remains at 92% (Fig. 2F and G), suggesting a good stability over time. Furthermore, as shown in Fig. 2G, the relative standard deviation (RSD) value of the signal intensity of the DNA incorporating agarose hydrogel slightly increased from 3.7% (0 day) to 5.3% (30 day), demonstrating a favorable accuracy for the analysis after 30 day storage.
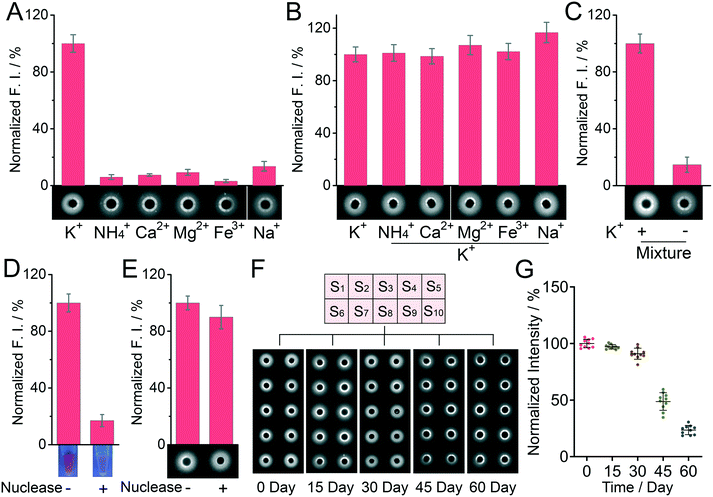 |
| Fig. 2 Verification of the characteristics of the DNA incorporating agarose hydrogel plate. (A) Selectivity investigation for K+ detection based on the DNA incorporating agarose hydrogel. The background signal is subtracted. K+ is at a concentration of 25 mM, other ions are at a concentration of 250 mM. The lower part: photographic images of the corresponding ions in the microwells. Selectivity investigation for K+ detection based on the DNA incorporating agarose hydrogel in a (B) binary-based competitive system “K+ + metal ion” and a (C) mixture of matrices “K+ + multi-metal ions”. Anti-degradation ability of the (D) solution system and the (E) DNA incorporating agarose hydrogel system under exonuclease I hydrolysis. 50 U mL−1 exonuclease I reacted 15 min in the solution and 1 day in the hydrogel, respectively. (F and G) Catalytic stability of the DNA incorporating agarose hydrogel during storage. Photographic images of the DNA incorporating agarose hydrogel were obtained with the gel imaging system and the fluorescence intensity of the microwells was acquired with ImageJ. | |
Detection of potassium ions in real samples
Lake water from Anqing Normal University (AQNU) and Shanghai University (SHU) were adopted as real samples, while an ion-selective electrode (ISE), a standard method for the detection of K+, was adopted for reference. Whether for the lake water from AQNU or SHU, it is shown that the detection results obtained by using the DNA incorporating agarose hydrogel were highly consistent with those obtained with the ISE (Fig. 3A and B, RSD < 9.2%). Parallel control results of the same sample in different microwells are also highly consistent with each other (Fig. 3C, RSD < 7.8%). It can be considered that the proposed DNA incorporating agarose hydrogel-based sensing platform can provide accurate detection results with reliability comparable to the standard method using an ISE. Besides, compared with the standard method, the proposed sensing platform provides a more portable and relatively high-throughput testing way in the detection of metal ions. The add-to-read manner is beneficial for the application of this sensing platform in harsh environments.
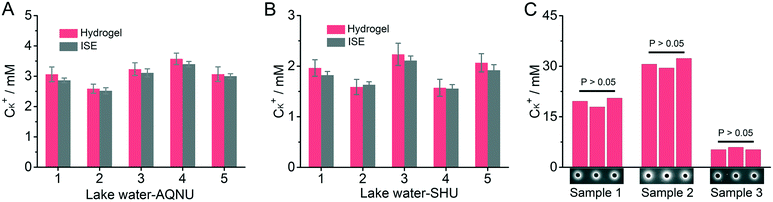 |
| Fig. 3 Demonstration of the potential capability for practical K+ detection based on the DNA incorporating agarose hydrogel plate in real samples, including (A) lake water from Shanghai University and (B) lake water from Anqing Normal University. The ion-selective electrode (ISE) was adopted for the reference for the detection of K+. (C) Parallel control results of the same sample in different microwells of the DNA incorporating agarose hydrogel. Inset: Images of the same sample in different microwells in the gel imaging system. | |
Detection of mercury ions by using the DNA incorporating agarose hydrogel
Finally, we studied if the DNA incorporating agarose hydrogel can be applied for the detection of other metal ions by altering the DNA probes. Hg2+ was adopted as the target. For the detection of Hg2+, a well-designed DNA probe with a hairpin structure is adopted and incorporated in the agarose hydrogel (Scheme 1B). Hg2+ can trigger the allosteric switch of the DNA probe, exposing the green fluorophores for further imaging. The principle of the DNA hydrogel for Hg2+ determination was firstly verified in solution. As illustrated in Fig. S11,† the significant increase of the green signal is obtained with the enhancement of the Hg2+ concentrations.
Results show that with the increase of the concentration of Hg2+, gradually increasing green fluorescence signals can be observed under UV light (Fig. 4A and B). Moreover, as shown in Fig. 4C, the quantitative results obtained using intelligent image recognition technology demonstrated the quantitative effects of the proposed method on a single sample, and the results show that this sensing system can directly output accurate results when the concentration of Hg2+ varies from 10 nM to 2.5 μM. Meanwhile, as shown in Fig. 4D and S12,† it is further proven that the linear detection range is 10 nM to 2.5 μM and the detection limit is 5.6 nM. The detection performance is comparable with some existing methods (Table S2†). It is worth noting that the detection effect of Hg2+ is much better than that of K+. According to the previous reports, this may be caused by the following two reasons: first, the fluorescence recovery effect of the NMM system is not as effective as the quencher system; second, the formation of the T–Hg2+–T structure is much easier because the affinity of Hg2+ in the T–Hg2+–T structure is higher than that of K+ in G-quadruplex.27,28 In the detection of the spiked samples, the recovery concentrations of our method are highly consistent with those of the standard method (Fig. 4E). Moreover, human serum, a complex biological matrix, was selected to demonstrate the application of the DNA hydrogel sensing system in real samples. Different concentrations of Hg2+ were spiked into the human serum and analyzed with the DNA hydrogel. Owing to the specificity between the T-rich region and Hg2+, the detection of Hg2+ in the human serum is also achieved (Fig. 4F). The recovery rate is ranging from 92% to 109%, and the deviation is within 9.9%, suggesting little interference from the non-target substances in real samples. The above results also demonstrate that the biosensing platform could be applied for the detection of other metal ions by only changing the sequences of metal ion-sensitive probes.
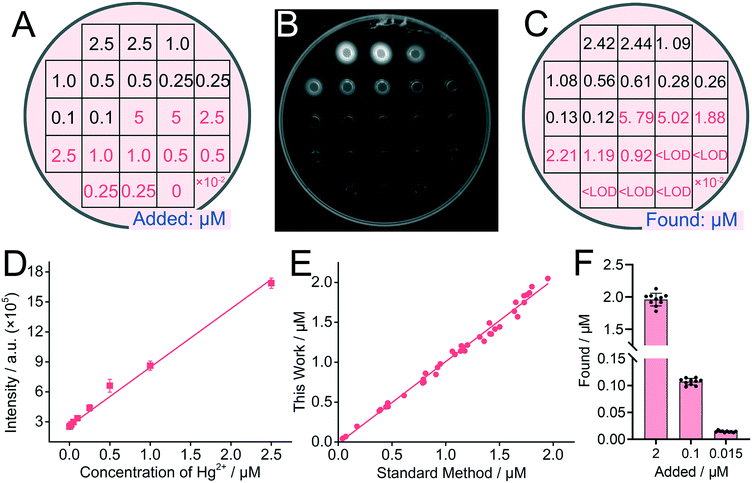 |
| Fig. 4 The universality of the proposed method. (A) The concentration of Hg2+ (μM) for each microwell. (B) The DNA incorporating agarose hydrogel plate for Hg2+ detection in water; the image was recorded with the gel imaging system. (C) The recovery concentration of Hg2+ (μM) for each microwell. (D) The linear relationship between the fluorescence intensity and Hg2+ concentration. (E) The consistency analysis of the standard method and this method in Hg2+ detection. (F) Detection of Hg2+ in spiked serum. | |
Conclusions
In summary, we have designed a functional DNA incorporating agarose hydrogel for quantitative detection of metal ions in a portable and easy-to-operate way. Potassium ions and mercury ions working as models have been successfully detected. The proposed DNA incorporating agarose hydrogel-based biosensing system offers several advantages: 1) the DNA incorporating agarose hydrogel provides a simple add-to-read manner for metal ion detection by integrating sample injection, reaction and indication together. 2) With the aid of intelligent image recognition technology, reliable quantitative results can be rapidly obtained without costly instruments. 3) The molecular sieve effect and the superior stability under nuclease degradation of the DNA incorporating agarose hydrogel enable the direct detection of metal ions in complex samples. 4) High-throughput testing can be achieved by punching multiple loaded microwells into the hydrogel. In addition to these advantages, the DNA incorporating agarose hydrogel also has the potential to be expanded for on-site detection of other metal ions by incorporating other DNA probes that are sensitive to specific metal ions (e.g. metal ion-dependent DNAzymes). Importantly, because of the advantages of the facile operability without the assistance of huge instruments and professional workers and the high-throughput testing ability of the portable semi-dry hydrogel, the biosensing platform holds great potential in POCT analysis for disease diagnosis and pollution monitoring.
Author contributions
X. M. and D. M. contributed equally in this work.
Conflicts of interest
There are no conflicts to declare.
Acknowledgements
This work was supported by the National Natural Science Foundation of China (Grant No. 21575088 and 31800670) and the Natural Science Foundation of Shanghai (19ZR1474200).
References
- P. Moitra, M. Alafeef, K. Dighe, M. B. Frieman and D. Pan, ACS Nano, 2020, 14, 7617–7627 CrossRef CAS.
- R. S. Alkasir, A. Rossner and S. Andreescu, Environ. Sci. Technol., 2015, 49, 9889–9897 CrossRef CAS.
- E. H. Lee, S. K. Lee, M. J. Kim and S. W. Lee, Food Chem., 2019, 287, 205–213 CrossRef CAS.
- Z. F. Gao, R. Liu, J. Wang, J. Dai, W. H. Huang, M. Liu, S. Wang, F. Xia, S. Zhang and L. Jiang, Nat. Protoc., 2020, 15, 316–337 CrossRef CAS.
- M. M. Calabretta, R. Alvarez-Diduk, E. Michelini, A. Roda and A. Merkoci, Biosens. Bioelectron., 2020, 150, 111902 CrossRef CAS.
- Z. Jin, X. Zhu, N. Wang, Y. Li, H. Ju and J. Lei, Angew. Chem., 2020, 59, 10446–10450 CrossRef CAS.
- X. Sun, Y. Jian, H. Wang, S. Ge, M. Yan and J. Yu, ACS Appl. Mater. Interfaces, 2019, 11, 16198–16206 CrossRef CAS.
- S. Cinti, D. Moscone and F. Arduini, Nat. Protoc., 2019, 14, 2437–2451 CrossRef CAS.
- Y. Gao, Z. Zhu, X. Xi, T. Cao, W. Wen, X. Zhang and S. Wang, Biosens. Bioelectron., 2019, 133, 177–182 CrossRef CAS.
- E. Vargas, H. Teymourian, F. Tehrani, E. Eksin, E. Sanchez-Tirado, P. Warren, A. Erdem, E. Dassau and J. Wang, Angew. Chem., Int. Ed., 2019, 58, 6376–6379 CrossRef CAS.
- N. Boehnke, S. Correa, L. Hao, W. Wang, J. P. Straehla, S. N. Bhatia and P. T. Hammond, Angew. Chem., 2020, 59, 2776–2783 CrossRef CAS.
- X. Gao, S. Li, F. Ding, H. Fan, L. Shi, L. Zhu, J. Li, J. Feng, X. Zhu and C. Zhang, Angew. Chem., Int. Ed., 2019, 58, 8719–8723 CrossRef CAS.
- Y. Li, Y. Ma, X. Jiao, T. Li, Z. Lv, C. Yang, X. Zhang and Y. Wen, Nat. Commun., 2019, 10, 1036–1038 CrossRef.
- R. Liu, Y. Huang, Y. Ma, S. Jia, M. Gao, J. Li, H. Zhang, D. Xu, M. Wu, Y. Chen, Z. Zhu and C. Yang, ACS Appl. Mater. Interfaces, 2015, 7, 6982–6990 CrossRef CAS.
- C. Kumari, D. Sain, A. Kumar, S. Debnath, P. Sahac and S. Dey, Dalton Trans., 2017, 46, 2524–2531 RSC.
- C. Wang, F. Hou and Y. Ma, Biosens. Bioelectron., 2015, 68, 156–162 CrossRef CAS.
- X. Yan, Y. Song, X. Wu, C. Zhu, X. Su, D. Du and Y. Lin, Nanoscale, 2017, 9, 2317–2323 RSC.
- J. Liu, L. Tian, Y. Qiao, S. Zhou, A. J. Patil, K. Wang, M. Li and S. Mann, Angew. Chem., 2020, 59, 6853–6859 CrossRef CAS.
- K. Chen, Y. Feng, Y. Zhang, L. Yu, X. Hao, F. Shao, Z. Dou, C. An, Z. Zhuang, Y. Luo, Y. Wang, J. Wu, P. Ji, T. Chen and H. Wang, ACS Appl. Mater. Interfaces, 2019, 11, 36458–36468 CrossRef CAS.
- Y. Sasaki, M. Oshikawa, P. Bharmoria, H. Kouno, A. Hayashi-Takagi, M. Sato, I. Ajioka, N. Yanai and N. Kimizuka, Angew. Chem., Int. Ed., 2019, 58, 17827–17833 CrossRef CAS.
- B. C. Yin, B. C. Ye, H. Wang, Z. Zhu and W. H. Tan, Chem. Commun., 2012, 48, 1248–1250 RSC.
- L. Zhang, J. P. Lei, L. Liu, C. F. Li and H. X. Ju, Anal. Chem., 2013, 85, 11077–11082 CrossRef CAS.
- S. Liu, W. Su, Y. Li, L. Zhang and X. Ding, Biosens. Bioelectron., 2018, 103, 1–5 CrossRef CAS.
-
G. Wu and H. Han, A DNA Polymerase Stop Assay for Characterization of G-Quadruplex Formation and Identification of G-Quadruplex-Interactive Compounds, Humana, New York, 2019 Search PubMed.
- R. Jin, D. Kong, X. Yan, X. Zhao, H. Li, F. Liu, P. Sun, Y. Lin and G. Lu, ACS Appl. Mater. Interfaces, 2019, 11, 27605–27614 CrossRef CAS.
- H. Qian, C. Y. Tay, M. I. Setyawati, S. L. Chia, D. S. Lee and D. T. Leong, Chem. Sci., 2017, 8, 1062–1067 RSC.
- Y. He and B. N. Jiao, RSC Adv., 2015, 5, 104116 RSC.
- A. C. Bhasikuttan and J. Mohanty, Chem. Commun., 2015, 51, 7581 RSC.
Footnotes |
† Electronic supplementary information (ESI) available: Fig. S1–S12 and Tables S1 and S2. See DOI: 10.1039/d0lc00855a |
‡ These authors contributed equally in this work. |
|
This journal is © The Royal Society of Chemistry 2021 |