DOI:
10.1039/D1GC01284C
(Paper)
Green Chem., 2021,
23, 4524-4530
Carbon dot/TiO2 nanocomposites as photocatalysts for metallaphotocatalytic carbon–heteroatom cross-couplings†
Received
13th April 2021
, Accepted 3rd June 2021
First published on 3rd June 2021
Abstract
Carbon dots have been previosly immobilized on titanium dioxide to generate photocatalysts for pollutant degradation and water splitting. Here we demonstrate that these nanocomposites are valuable photocatalysts for metallaphotocatalytic carbon–heteroatom cross-couplings. These sustainable materials show a large applicability, high photostability, excellent reusability, and broadly absorb across the visible-light spectrum.
Introduction
Carbon dots (CDs) are quasi-spherical fluorescent carbon-based materials with a size of typically less than 10 nm.1–5 CDs are easily prepared through top-down or bottom-up approaches from a variety of carbon sources that permit to adjust their chemical compositions and tune their photoluminescence (PL) properties.6,7 Their chemical inertness and biocompatibility has prompted applications in sensing, bioimaging, and nanomedicine.7–11 Moreover, the surface functional groups enabled applications as sustainable nano-organocatalysts for synthetic transformations. The superficial carboxylic acid, hydroxy, or amino functionalities were exploited in acid–base, hydrogen bond, or amine-catalysed reactions.12–15
CDs are also promising metal-free photocatalysts for pollutant degradation, H2 evolution and CO2 conversion, owing to their photostability, light-harvesting ability and electron-transfer efficiency.16–19 The high solubility of CDs in water makes them a suitable alternative to hydrophobic organic materials, such as carbon nitride and graphite.20 This feature permitted to use CDs in combination with nickel catalysis for H2 evolution in aqueous solution.21,22
However, due to their short PL lifetimes,23,24 examples of CDs as photocatalysts for selective organic synthesis are scarce14,15 when compared to common photocatalysts, such as ruthenium (Ru) and iridium (Ir) polypyridyl complexes that have long-lived triplet excited states.25–27 To overcome the problems associated with the short-lived excited states, CDs can be immobilized on heterogeneous semiconductors, such as titanium dioxide, to generate a composite material that absorbs visible-light and generates a long-lived charge-separated species.28–30 Still, the applications of such composites remained limited to water splitting, CO2 reduction, and pollutant degradation (Fig. 1A).31–33
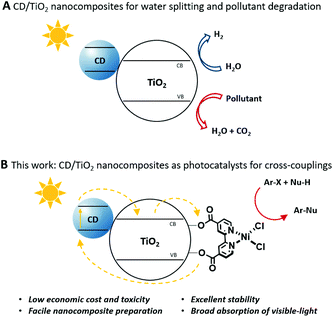 |
| Fig. 1 Schematic representation of CD/TiO2 nanocomposites as photocatalysts for water splitting, pollutant degradation (A) and metallaphotocatalytic carbon–heteroatom cross-couplings (B). | |
The combination of a photo- and a nickel catalyst (termed metallaphotocatalysis) triggers many important carbon–heteroatom and carbon–carbon cross-couplings using light as sustainable energy source.34 Suitable photocatalysts for these reactions range from ruthenium and iridium polypyridyl complexes and organic dyes to heterogeneous semiconductors.34 Moreover, nickel complexes and photocatalysts were combined in bifunctional heterogeneous materials, such as metal–organic frameworks,35,36 or organic polymers.37
Titanium dioxide can be sensitized with organic dyes to serve as a visible light photocatalyst for selective organic transformations.38,39 Recently, it was shown that the immobilization of a Ni(II) catalyst and an organic dye on the surface of metal oxides provides a heterogeneous catalytic system for metallaphotocatalytic carbon–carbon and carbon–heteroatom cross-couplings that overcomes the problems associated with short-lived singlet excited states of organic dyes.40 Following this seminal work, we show that CDs are a valuable alternative to organic dyes in such catalytic systems due to (i) their low economic cost and toxicity, (ii) their facile immobilization on semiconductors, (iii) their broad absorption across the visible-light spectrum, and (iv) their superior photo- and chemical stability (Fig. 1B).21,30
Results and discussion
Preparation of CD1/TiO2 nanocomposite
Carbohydrates are an attractive carbon source for CD synthesis owing to their low cost, high solubility in water, easy carbonisation at relatively low temperatures, and presence of heteroatoms.41 We therefore began our investigations by preparing CD1 from glucosamine hydrochloride (GlcN·HCl), following a microwave-based carbonisation method (Fig. 2A).42 Doping with β-alanine (β-Ala) ensured a high amount of surface carboxylic acid groups (Fig. S6 and S7†).42 The zeta potential in the range of −11.1 to +18.7 mV suggested the presence of several functional groups (carboxylic acids, alcohols, and amino groups) on the surface of CD1 (Fig. S8†).42,43
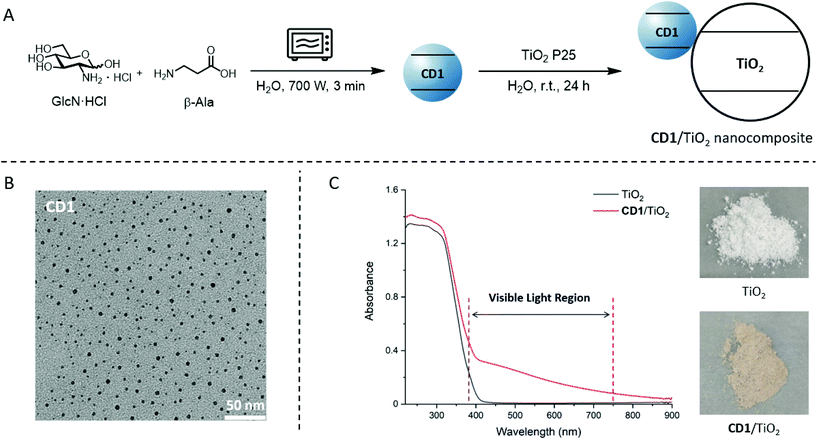 |
| Fig. 2 Schematic representation of the preparation of CD1 and CD1/TiO2 nanocomposite (A). TEM image of CD1 (B). UV-Vis absorption (solid state) and photographs of TiO2 and CD1/TiO2 nanocomposite (C). GlcN·HCl = Glucosamine hydrochloride. β-Ala = β-Alanine. | |
Transmission electron microscopy (TEM) confirmed a spherical shape of the CD nanoparticles with a diameter of about 4 nm (Fig. 2B and Fig. S2†). The X-ray diffraction (XRD) profile showed a single broad peak (2θ = 23°), indicating the amorphous structure of CD1 (Fig. S9†). A colloidal solution of CD1 in H2O emitted blue light under UV light irradiation (λex = 366 nm) (Fig. S3†). Spectroscopic analysis showed an absorption peak at 276 nm (Fig. S5†) and a PL emission maximum at ∼460 nm (λex = 360 nm, Fig. S4†). A PL lifetime of 4.45 ns was measured by fitting the PL decay curve of CD1 (Fig. S10†).
CD1 was immobilized on the surface of TiO2 P25 by stirring a mixture of the two components in water (mass ratio 1
:
1; Fig. 2A). The resulting brown powder (CD1/TiO2) was analysed by scanning electron microscopy (SEM) and energy dispersive X-ray spectroscopy (EDX) (Fig. S15†). The morphology and size of the nanocomposites remained similar to unfunctionalized TiO2. The increased carbon content confirmed the immobilization of CD1. UV-Vis spectroscopy of the resulting material confirmed its extended absorption in the visible-light region (Fig. 2C).
Applicability of CD1/TiO2 as photocatalyst
The applicability of CD1/TiO2 as photocatalyst for metallaphotocatalytic cross-couplings was tested for the C–O arylation of N-(tert-butoxycarbonyl)-L-proline (Boc-Pro-OH) with methyl 4-iodobenzoate using visible-light (Fig. 3A).40,44 A Ni(II) complex that contains carboxylic acid groups was employed to bind to the nanocomposite. The selective formation of 83% of the desired ester product (1) was observed when the reaction was irradiated with blue (440 nm) light for 24 h (entry 1). Control experiments confirmed the necessity of TiO2, CD1, and the carboxylic acid functionalized ligand 2,2′-bipyridine-4,4′-dicarboxylic acid (dcbpy) (entries 2–5).
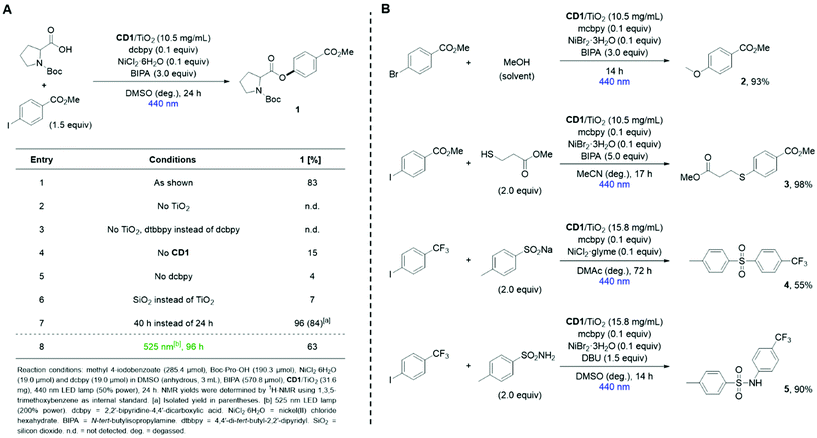 |
| Fig. 3 Optimized conditions and control experiments for the cross-coupling of Boc-Pro-OH with methyl 4-iodobenzoate using CD1/TiO2 nanocomposite (A). Application of CD1/TiO2 as photocatalyst for C–O, C–S, and C–N cross-couplings (B). | |
A previous report that used molecular dyes with short excited state lifetimes instead of CD1 showed that insulating materials, such as SiO2, can be used instead of TiO2 for the same reaction.40 In this case, it was proposed that the close proximity between dye molecules and the nickel complex is responsible for productive catalysis. Using a CD1/SiO2 nanocomposite, we only observed a modest yield of 7% of the desired product (entry 6), suggesting that an electronic communication between the excited CD and the immobilized nickel complex “through” a semiconducting material is crucial.
Using CD1/TiO2 an almost quantitative formation of 1 required 40 h (entry 7). The broad absorption of the nanocomposite also enabled cross-coupling at longer wavelengths (525 nm), albeit with longer reaction times (entry 8). It is worth noting that CD1/TiO2 is also highly active using very low loadings (Table S6†), and that the nanocomposite is bench-stable and does not lose its catalytic activity upon storage at room temperature for 26 weeks (Table S5†).
To our delight, CD1/TiO2 served as an active photocatalyst for a range of metallaphotocatalytic carbon–heteroatom cross-couplings.34 Moderate to excellent yields were obtained for the coupling of aryl halides with an alcohol, a thiol, a sodium sulfonate, and a sulfonamide using slightly adapted conditions (Fig. 3B).
Photostability and recyclability studies
Next, we sought to compare the photostability of the CD1/TiO2 nanocomposite with TiO2 that was functionalized with the organic dye fluorescein (Fluo/TiO2) (Fig. 4). The functionalized semiconductors were pre-irradiated with blue light for a defined amount of time and subsequently used as photocatalysts in the metallaphotocatalytic C–O arylation of Boc-Pro-OH. The photocatalytic performance of CD1/TiO2 remained unchanged even after 72 h exposure to light. In contrast, Fluo/TiO2 produced significantly lower yields after 6 h irradiation. The yield obtained with the Fluo/TiO2 photocatalyst did not decrease linearly with the irradiation time, but seemed to reach a plateau after 6–12 h pre-irradiation. We assume that the prolonged irradiation could promote the formation of fluorescein degradation products that still serve as a sensitizer.45,46
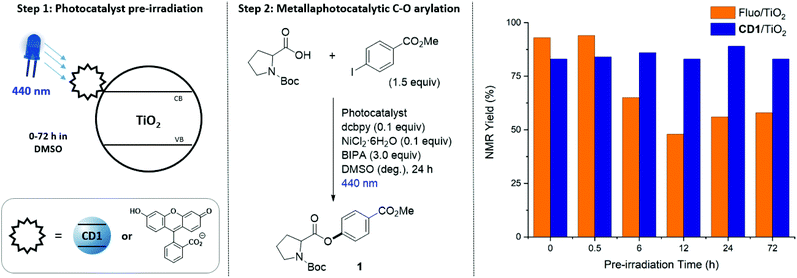 |
| Fig. 4 Photobleaching experiments to compare the photostability of CD1/TiO2 and Fluo/TiO2. The two photocatalysts were pre-irradiated with blue light and then used in the metallaphotocatalytic C–O arylation. Yields were determined via1H-NMR using 1,3,5-trimethoxybenzene as internal standard. Fluo = fluorescein. | |
The CD1/TiO2 nanocomposite was characterized before and after the catalytic reaction (Table S3†). Inductively coupled plasma - optical emission spectrometry (ICP-OES) revealed the presence of nickel in the CD1/TiO2 nanocomposite after the C–O cross-coupling (Fig. 3A, entry 7). This indicated that the nickel complex remained immobilized on CD1/TiO2, prompting us to explore the recyclability of the bifunctional heterogeneous catalyst (Fig. 5). Recyclability experiments were performed using the reaction conditions reported in Fig. 3A (entry 1). After each cycle, the heterogeneous material was separated, washed, and reused in the next C–O cross-coupling. Excellent catalytic performances were observed even after four recycling cycles. Importantly, the addition of nickel salt or nickel complex after each cross-coupling cycle, which was previously required in a related approach,40 was not only unnecessary, but significantly decreased the catalytic activity. This may be ascribed to Ni accumulation and formation of nickel-black upon irradiation by high-energy light (Fig. S28†).47
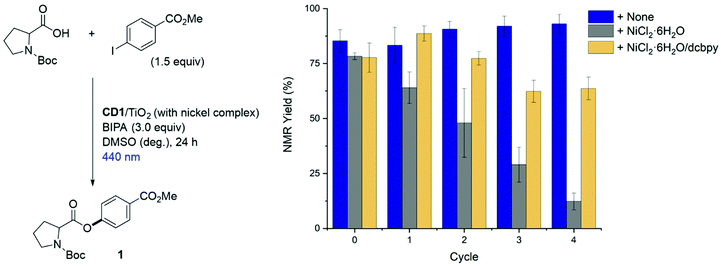 |
| Fig. 5 Reusability of CD1/TiO2 nanocomposite decorated with a nickel complex in the metallaphotocatalytic C–O arylation. | |
Overall, these results underscore the potential of CD1/TiO2 nanocomposites as a robust, cheap, and green photocatalyst for applications in organic chemistry.
Screening of different CD photosensitizers
Having demonstrated the potential of CD1 as photosensitizer for dual photoredox/Ni catalysis, we assessed the effect of different carbon sources and doping agents on the photocatalytic reaction (Fig. 6A). A first set of CDs was synthesized maintaining GlcN·HCl as the carbon source and screening different doping agents. 1,3-Diaminobenzene, l-cysteine (l-Cys), poly(ethylene glycol) (average Mn 400) (PEG), and glycine (Gly) were tested. Each compound was selected to introduce respectively aromatic groups,48 sulphur atoms, polymers to enhance surface passivation,49 or amino acid analogues of β-Ala. A second set of CDs was based on β-Ala as doping agent and different carbon sources. Three monosaccharides (glucose (Glc), N-acetyl-glucosamine (GlcNAc), galactose (Gal)), a disaccharide (D-lactose (Lac)) and a polysaccharide (pullulan) were tested to explore the influence of chain length and sugar structure on the photocatalytic performance. All CD precursors resulted in spherical nanoparticles with diameters smaller than 10 nm (Fig. S11†). Most CDs showed similar photophysical properties, with the exception of CD2 that emitted bright green light under UV light irradiation (λex = 366 nm) (Fig. S12 and S13†) and had an absorption maximum at 363 nm (Fig. S14†). All CDs were immobilized on TiO2 P25 to prepare nine CD/TiO2 nanocomposites able to absorb light in the visible region (Fig. 6B and Fig. S18†). While most UV-Vis spectra share a similar profile, CD2/TiO2 nanocomposites exhibit a strong absorption band with a maximum at 466 nm. The photocatalytic performances of all nanocomposites were compared (Fig. 6C). Despite the broad and intense absorption in the visible range, CD2/TiO2 resulted in low yields, whereas all other nanocomposites showed good to excellent results. For a fair comparison it should be noted that, even though all nanocomposites were prepared starting with an initial 1
:
1 mass ratio of CD
:
TiO2, differences in immobilization might play a role in the photocatalytic results.
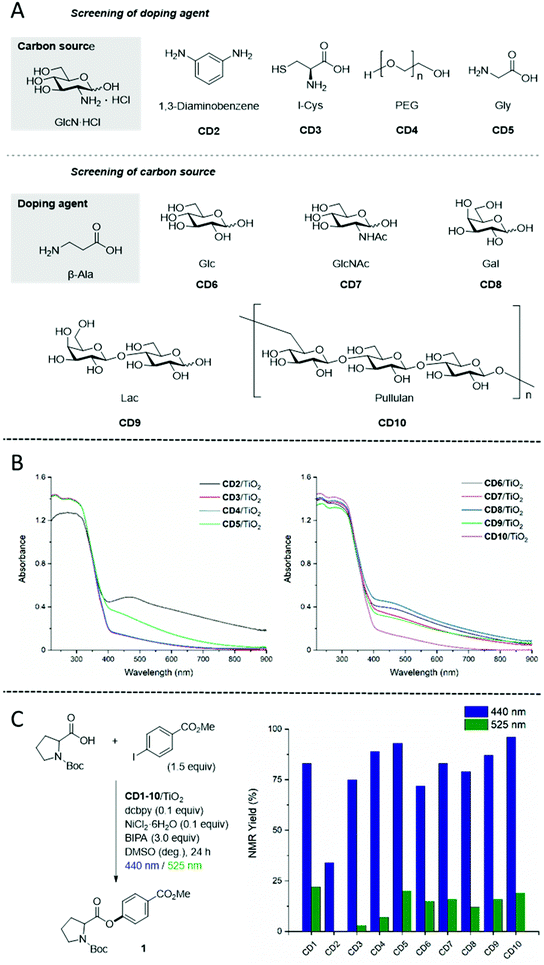 |
| Fig. 6 Chemical structures of carbon sources and doping agents used for CD synthesis (A). UV-Vis absorption spectra (solid state) of CD/TiO2 nanocomposites (B). Evaluation of different CD/TiO2 nanocomposites as photocatalyst for the metallaphotocatalytic C–O arylation of Boc-Pro-OH with methyl 4-iodobenzoate (C). | |
These results show that the system is highly flexible and that CD photosensitizers can be prepared from several starting materials, without affecting the catalytic performances. For example, excellent results were obtained for CD9 and CD10 prepared using lactose and pullulan as carbon source, respectively. These materials are highly abundant and cheap (lactose is a waste product of the dairy industry that is produced at >6 million ton scale every year) suggesting the possibility of turning naturally sourced polysaccharides from waste materials into valuable catalysts. Fine tuning of the elemental composition and its effect on the photocatalytic reaction will be explored in follow up studies.
Conclusions
Carbohydrate-based CDs were immobilized on TiO2 to prepare nanocomposites, offering a cheap and robust alternative to organic dyes. This approach allowed us to overcome the issues associated with the short excited state lifetime of CDs. We demonstrated that CDs are valuable photocatalysts for metallaphotocatalytic carbon–heteroatom cross-couplings. High conversions were observed under irradiation by either blue or green light. Photobleaching experiments confirmed that the catalytic performances of the CD/TiO2 nanocomposites are not affected by long-time light irradiation prior to their use in catalytic reactions. Recycling experiments showed the excellent reusability of this catalytic system in C–O cross-coupling reactions. CDs could be prepared from different carbohydrate sources and doping agents, without significant difference in the catalytic performance. This is particularly important in the perspective of using polysaccharide waste materials to generate valuable photocatalysts.
Conflicts of interest
There are no conflicts to declare.
Acknowledgements
We gratefully acknowledge the Max-Planck Society for generous financial support. M.D. and Z.Z. acknowledge financial support from the MPG-FhG Cooperation Project Glyco3Dysplay, the German Federal Ministry of Education and Research (BMBF, grant number 13XP5114) and the China Scholarship Council. S.R. and B.P. acknowledge financial support by a Liebig Fellowship of the German Chemical Industry Fund (Fonds der Chemischen Industrie, FCI). B.P. thanks the Deutsche Forschungsgemeinschaft (DFG, German Research Foundation) for financial support (PI 1635/2-1). Open Access funding provided by the Max Planck Society.
Notes and references
- X. Xu, R. Ray, Y. Gu, H. J. Ploehn, L. Gearheart, K. Raker and W. A. Scrivens, J. Am. Chem. Soc., 2004, 126, 12736 CrossRef CAS PubMed.
- G. A. M. Hutton, B. C. M. Martindale and E. Reisner, Chem. Soc. Rev., 2017, 46, 6111 RSC.
- Z. L. Wu, Z. X. Liu and Y. H. Yuan, J. Mater. Chem. B, 2017, 5, 3794 RSC.
- J. Zhan, B. Geng, K. Wu, G. Xu, L. Wang, R. Guo, B. Lei, F. Zheng, D. Pan and M. Wu, Carbon, 2018, 130, 153 CrossRef CAS.
- L. Vallan, E. P. Urriolabeitia, F. Ruiperez, J. M. Matxain, R. Canton-Vitoria, N. Tagmatarchis, A. M. Benito and W. K. Maser, J. Am. Chem. Soc., 2018, 140, 12862 CrossRef CAS PubMed.
- S. Y. Lim, W. Shen and Z. Gao, Chem. Soc. Rev., 2015, 44, 362 RSC.
- K. Jiang, S. Sun, L. Zhang, Y. Lu, A. Wu, C. Cai and H. Lin, Angew. Chem., Int. Ed., 2015, 54, 5360 CrossRef CAS PubMed.
- Z. Shen, C. Zhang, X. Yu, J. Li, Z. Wang, Z. Zhang and B. Liu, J. Mater. Chem. C, 2018, 6, 9636 RSC.
- B. Zhi, Y. Cui, S. Wang, B. P. Frank, D. N. Williams, R. P. Brown, E. S. Melby, R. J. Hamers, Z. Rosenzweig, D. H. Fairbrother, G. Orr and C. L. Haynes, ACS Nano, 2018, 12, 5741 CrossRef CAS PubMed.
- S. Sri, R. Kumar, A. K. Panda and P. R. Solanki, ACS Appl. Mater. Interfaces, 2018, 10, 37835 CrossRef CAS PubMed.
- H. Zhao, J. Duan, Y. Xiao, G. Tang, C. Wu, Y. Zhang, Z. Liu and W. Xue, Chem. Mater., 2018, 30, 3438 CrossRef CAS.
- B. Majumdar, S. Mandani, T. Bhattacharya, D. Sarma and T. K. Sarma, J. Org. Chem., 2017, 82, 2097 CrossRef CAS PubMed.
- X. Pei, D. Xiong, H. Wang, S. Gao, X. Zhang, S. Zhang and J. Wang, Angew. Chem., Int. Ed., 2018, 57, 3687 CrossRef CAS PubMed.
- Y. Han, H. Huang, H. Zhang, Y. Liu, X. Han, R. Liu, H. Li and Z. Kang, ACS Catal., 2014, 4, 781 CrossRef CAS.
- G. Filippini, F. Amato, C. Rosso, G. Ragazzon, A. Vega-Peñaloza, X. Companyó, L. Dell'Amico, M. Bonchio and M. Prato, Chem, 2020, 6, 3022 CAS.
- S. Hu, R. Tian, L. Wu, Q. Zhao, J. Yang, J. Liu and S. Cao, Chem. – Asian J., 2013, 8, 1035 CrossRef CAS PubMed.
- P. Yang, J. Zhao, J. Wang, H. Cui, L. Li and Z. Zhu, Chemphyschem, 2015, 16, 3058 CrossRef CAS PubMed.
- J. Wu, S. Ma, J. Sun, J. I. Gold, C. Tiwary, B. Kim, L. Zhu, N. Chopra, I. N. Odeh, R. Vajtai, A. Z. Yu, R. Luo, J. Lou, G. Ding, P. J. Kenis and P. M. Ajayan, Nat. Commun., 2016, 7, 13869 CrossRef CAS PubMed.
- L. Cao, S. Sahu, P. Anilkumar, C. E. Bunker, J. Xu, K. A. S. Fernando, P. Wang, E. A. Guliants, K. N. Tackett, II and Y. P. Sun, J. Am. Chem. Soc., 2011, 133, 4754 CrossRef CAS PubMed.
- H. Li, X. He, Z. Kang, H. Huang, Y. Liu, J. Liu, S. Lian, C. H. Tsang, X. Yang and S. T. Lee, Angew. Chem., Int. Ed., 2010, 49, 4430 CrossRef CAS PubMed.
- B. C. Martindale, G. A. Hutton, C. A. Caputo and E. Reisner, J. Am. Chem. Soc., 2015, 137, 6018 CrossRef CAS PubMed.
- Y. Liu, Y. Zhao, Q. Wu, X. Wang, H. Nie, Y. Zhou, H. Huang, M. Shao, Y. Liu and Z. Kang, Chem. Eng. J., 2021, 409, 128184 CrossRef CAS.
- Z. Zhou, P. Tian, X. Liu, S. Mei, D. Zhou, D. Li, P. Jing, W. Zhang, R. Guo, S. Qu and A. L. Rogach, Adv. Sci., 2018, 5, 1800369 CrossRef PubMed.
- S. Li, K. Ji, M. Zhang, C. He, J. Wang and Z. Li, Nanoscale, 2020, 12, 9533 RSC.
- D. M. Schultz and T. P. Yoon, Science, 2014, 343, 1239176 CrossRef PubMed.
- C. K. Prier, D. A. Rankic and D. W. MacMillan, Chem. Rev., 2013, 113, 5322 CrossRef CAS PubMed.
- L. Marzo, S. K. Pagire, O. Reiser and B. Konig, Angew. Chem., Int. Ed., 2018, 57, 10034 CrossRef CAS PubMed.
- Y. X. Weng, L. Li, Y. Liu, L. Wang and G. Z. Yang, J. Phys. Chem. B, 2003, 107, 4356 CrossRef CAS.
- S. Agrawal, N. J. English, K. R. Thampi and J. M. MacElroy, Phys. Chem. Chem. Phys., 2012, 14, 12044 RSC.
- L. Xu, X. Bai, L. Guo, S. Yang, P. Jin and L. Yang, Chem. Eng. J., 2019, 357, 473 CrossRef CAS.
- R. Shi, Z. Li, H. Yu, L. Shang, C. Zhou, G. I. N. Waterhouse, L. Z. Wu and T. Zhang, ChemSusChem, 2017, 10, 4650 CrossRef CAS PubMed.
- J. Zhang, X. Zhang, S. Dong, X. Zhou and S. Dong, J. Photochem. Photobiol., A, 2016, 325, 104 CrossRef CAS.
- M. Li, M. Wang, L. Zhu, Y. Li, Z. Yan, Z. Shen and X. Cao, Appl. Catal., B, 2018, 231, 269 CrossRef CAS.
- C. Zhu, H. Yue, J. Jia and M. Rueping, Angew. Chem., Int. Ed., 2021, 60, 2 CrossRef.
- G. Lan, Y. Quan, M. Wang, G. T. Nash, E. You, Y. Song, S. S. Veroneau, X. Jiang and W. Lin, J. Am. Chem. Soc., 2019, 141, 15767 CrossRef CAS PubMed.
- Y.-Y. Zhu, G. Lan, Y. Fan, S. S. Veroneau, Y. Song, D. Micheroni and W. Lin, Angew. Chem., Int. Ed., 2018, 57, 14090 CrossRef CAS PubMed.
- Y. Pan, N. Zhang, C.-H. Liu, S. Fan, S. Guo, Z.-M. Zhang and Y.-Y. Zhu, ACS Catal., 2020, 10, 11758 CrossRef CAS.
- D. Franchi and Z. Amara, ACS Sustainable Chem. Eng., 2020, 8, 15405 CrossRef CAS.
- S. Gisbertz and B. Pieber, ChemPhotoChem, 2020, 4, 456 CrossRef CAS.
- S. Reischauer, V. Strauss and B. Pieber, ACS Catal., 2020, 10, 13269 CrossRef CAS.
- S. Hill and M. C. Galan, Beilstein J. Org. Chem., 2017, 13, 675 CrossRef CAS PubMed.
- S. A. Hill, D. Benito-Alifonso, S. A. Davis, D. J. Morgan, M. Berry and M. C. Galan, Sci. Rep., 2018, 8, 12234 CrossRef PubMed.
- S. A. Hill, D. Benito-Alifonso, D. J. Morgan, S. A. Davis, M. Berry and M. C. Galan, Nanoscale, 2016, 8, 18630 RSC.
- B. Pieber, J. A. Malik, C. Cavedon, S. Gisbertz, A. Savateev, D. Cruz, T. Heil, G. Zhang and P. H. Seeberger, Angew. Chem., Int. Ed., 2019, 58, 9575 CrossRef CAS PubMed.
- U. Kriiger and R. Memming, Ber. Bunsenges. Phys. Chem., 1974, 78, 670 Search PubMed.
- Q. Zheng and L. D. Lavis, Curr. Opin. Chem. Biol., 2017, 39, 32 CrossRef CAS PubMed.
- S. Gisbertz, S. Reischauer and B. Pieber, Nat. Catal., 2020, 3, 611 CrossRef CAS.
- S. A. Hill, S. Sheikh, Q. Zhang, L. Sueiro Ballesteros, A. Herman, S. A. Davis, D. J. Morgan, M. Berry, D. Benito-Alifonso and M. C. Galan, Nanoscale Adv., 2019, 1, 2840 RSC.
- L. Li and T. Dong, J. Mater. Chem. C, 2018, 6, 7944 RSC.
Footnote |
† Electronic supplementary information (ESI) available. See DOI: 10.1039/d1gc01284c |
|
This journal is © The Royal Society of Chemistry 2021 |