DOI:
10.1039/D0GC03063E
(Paper)
Green Chem., 2021,
23, 561-574
Low carbon hydrogen production from a waste-based biorefinery system and environmental sustainability assessment†
Received
9th September 2020
, Accepted 17th November 2020
First published on 18th November 2020
Abstract
Production of low carbon biofuels and biochemicals from renewable feedstocks using a biological process is considered a sustainable alternative to the fossil-fuel-based linear economy. This study demonstrated the pilot-scale (10 m3) production of biohydrogen (bio-H2) and volatile fatty acids/carboxylic acids (VFA) through acidogenic fermentation (AF) using food waste (FW) as renewable feedstock. Bio-H2 production of 54
288 L (155.10 LH2 per kg COD) along with 25.77 g L−1 of VFA composed of acetic (HAc: 15.2 ± 1.98 g L−1), propionic (HPr: 4.89 ± 1.26 g L−1) and butyric (HBu 5.67 ± 0.96 g L−1) acids was achieved within 48 h of the fermentation period. The further acidogenic process was integrated with a biorefinery platform (methanogenesis + photosynthesis) in a circular loop strategy which helped derive multiple biobased products (CH4, algal biomass, O2 and treated water for reuse) from fatty acid-rich acidogenic effluent and untreated COD of AF. The whole bio-manufacturing unit (acidogenesis + methanogenesis + photosynthesis) converted the renewable feedstock (waste/wastewater) into fuels and platform chemicals, in analogy to a conventional oil refinery with a maximized resource recovery. The life cycle assessment (LCA) tool was employed to study the environmental impact of both the bio-H2 (standalone, ST) and waste biorefinery (WB) processes, and the results depicted that the WB approach offered a relatively low impact (approx. 3.5 fold less than ST). The approach helped determine the flow of carbon and its conversion to products; this aided the reduction of carbon emissions as well as minimized the burden on natural resources with the biosynthesis of green H2 and other value-added products, addressing carbon neutrality with bioeconomy.
1. Introduction
Greater demand for energy/materials due to the finite availability of the fossil reserves associated with environmental and societal issues creates the need for the development of new and sustainable strategies that can result in renewable and carbon-neutral energy/materials.1 A shift from conventional fossil-based pathways to ecologically sustainable energy systems and production methods is an essential prerequisite for the anticipated global mission of climate neutrality.2,3 Hydrogen (H2) is the most promising clean energy carrier with an energy density (LHV, 120 MJ kg−1) higher than the liquefied natural gas (54.4 MJ kg−1), ethanol (29.6 MJ kg−1) and methane (50 MJ kg−1).4–6 Hydrogen is being considered as a future energy carrier and transition to the ‘Hydrogen Economy’ is marching rapidly around the global energy portfolio. At present, H2 is mostly produced from a wide source of feedstock, such as natural gas, coal, biomass, and solar energy (thermal and photovoltaic).7–11 Currently, fossil-based H2 production is achieved through various physicochemical processes, such as steam reforming of natural gas followed by cracking oil products, coal gasification, and water electrolysis.12,13 Requirement for non-renewable fossil resources, excessive energy inputs for production, and significant emissions of greenhouse gases (GHGs) during the production life cycle are some of the inherent limitations of traditional H2 production, irrespective of the conversion technology used and the energy source employed.
In the sustainability context, H2 production should be renewable in nature with low embodied carbon by adopting a biological process for its synthesis. Biohydrogen (bio-H2) production through a dark-fermentation route (acidogenic fermentation) using biogenic waste/wastewater as a feedstock is a promising pathway.14,15 Along with bio-H2, the acidogenic process concomitantly produces short-chain fatty acids/volatile fatty acids (VFA) as main by-products.15,16 One mole of glucose can produce 4 moles of bio-H2 along with 2 moles of acetic acid through dark-fermentation (eqn (3)). When the process proceeds by the butyric acid pathway, two moles of bio-H2 are produced (eqn (4)). In contrast, the propionic acid (eqn 5) and lactic acid pathways consume two and one moles of bio-H2, respectively.
VFAs are platform chemicals that are normally used as building blocks for the production of polymers, acidulants, preservatives and flavouring agents or as precursors for the synthesis of chemicals.16–18 Waste is a global issue with significant availability; therefore, bio-H2 production from renewable waste as feedstock reduces the burden on the environment through remediation and avoids emissions from direct and indirect use of fossil-based feedstock.3,19 In recent years, the biorefinery concept has emerged as an alternative to address the fossil-based resource depletion and associated environmental impacts in addition to valorising a multitude of biobased products.14,20–22 A biorefinery, when designed systematically, may address the great prevailing challenges to environmental sustainability through efficient utilization of resources with lower emissions and switching to a circular economy model from linear economy models. These biobased products (low carbon) positively minimize the dependency on fossil-derived resources and therefore reduce the respective environmental issues to establish low carbon economy and systems.23 Due to its renewable and carbon neutral nature, bio-H2 is gaining attention in the framework of sustainability.24 Over the last two decades, researchers have performed numerous studies in order to improve bio-H2 productivity from different feedstocks on lab and semi-pilot scales.25–30 This communication presents and discusses bio-H2 production at the pilot scale (10 m3) integrated with a waste biorefinery facility to maximize resource recovery in a circular loop. The impact of waste-derived bio-H2 on the environment was studied using life cycle analysis (LCA; ISO 14040:2006) tools considering all the inputs and outputs in the form of materials and energy to and from the system to assess its relative environmental sustainability.31
2. Materials and methods
2.1. Food waste
Composite food waste (FW) was collected from the institute canteen. The collected FW was composed of cooked rice (47 ± 4%), boiled vegetables (16 ± 2%), vegetable peels (18 ± 3%), spoiled fruits and vegetables (6 ± 2%), and egg/meat waste (13 ± 1%). The FW was masticated using a blender and then filtered through a stainless-steel mesh (1.1 mm) to remove the coarse materials. Oil was separated using a gravity separating system. The homogenized FW was characterized and found to have 230 ± 4 g L−1 COD, 0.7 ± 0.2 g L−1 volatile fatty acids (VFA), 37 ± 1.2 mg L−1 nitrates, 13 ± 1.6 mg L−1 sulphates and 19 ± 2.4 mg L−1 phosphates. The oil-free filtrate was then used as a substrate by adjusting the organic load of feed to 50 g COD per L.
2.2. Scaling up of the hydrogen production process – pilot plant (10 m3)
2.2.1. Standalone acidogenic process.
The acidogenic bioreactor (total/working volume 10/8 m3; L/D ratio 2) was fabricated with SS316L to operate in up-flow mode. The major unit operations of the pilot plant system consisted of a waste shredder, waste/wastewater feeder, multi-utility buffer tank/pre-fermentation tank (2 m3), inoculum preparation tank (0.2 m3), acid–base holding tank, H2 gas collector (0.2 m3), automated H2 flare and water storage tank. All individual unit operations of the pilot plant are shown in Fig. 1. Accessories, namely an air compressor, online gas flow meter, moisture trap and online sensors (pH and temperature), were also integrated into the processes at appropriate places. The reactor liquid volume was filled with moving bed media (void fraction ∼0.18) to support the growth of acidogenic consortia. The pilot plant was designed to have control and process flexibility. Every unit in the plant was systematically placed to minimize energy input.
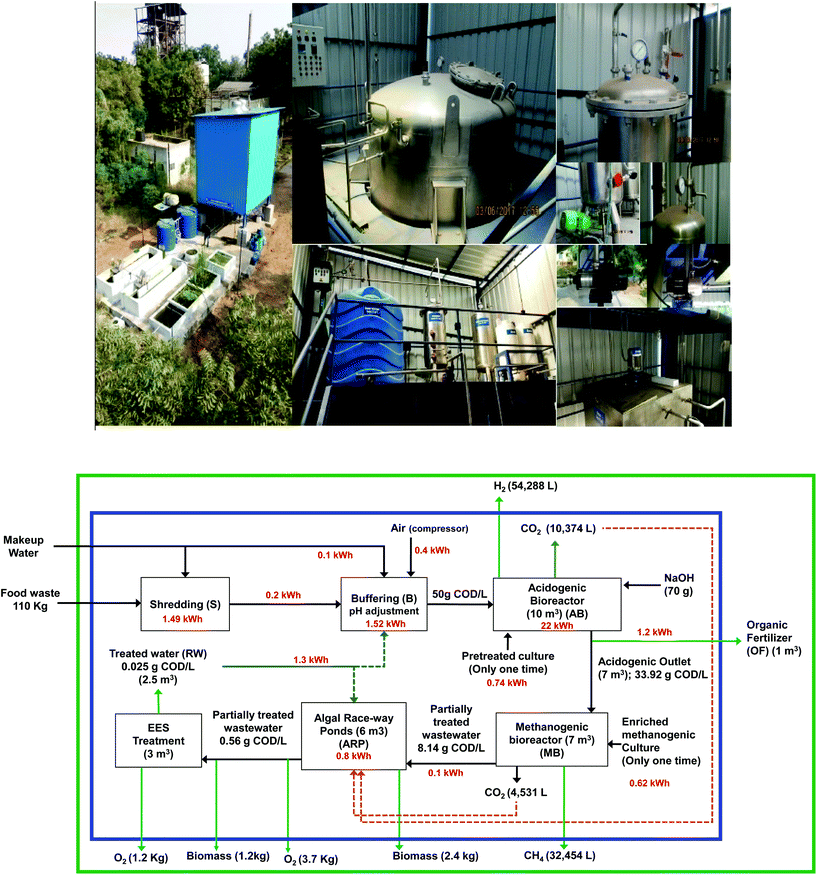 |
| Fig. 1 Aerial view (photographs) of pilot plant (10 m3) with its internal components integrated with a waste biorefinery installed at CSIR-IICT (above). Process flow diagram of acidogenic bio-H2 production integrated with methanogenesis and photosynthesis in a closed loop biorefinery to produce multiple biobased products from biogenic waste (below). | |
2.2.2. Waste biorefinery-integrated process.
To make the process sustainable, acidogenic bio-H2 (AB) production was integrated with a waste biorefinery facility to harness multiple biobased products from fatty acid-rich acidogenic outlet (AO). In addition to converting AO to multiple biobased products, this integrated process also treats wastewater simultaneously. The first bioprocess in the integrated biorefinery is methanogenesis (MB; total volume/working volume of 4.0/3.5 m3 × 2 no), which converts the AO to biomethane at pH 7. The methanogenic outlet was then transferred to algal raceway ponds (ARP; 3.0 m3 × 2) for the mixotrophic cultivation of microalgal biomass along with the utilization of CO2. The photosynthesis unit reduces the residual carbon load in the wastewater and then finally passes through an advanced ecological engineered system (EES; 1 m3 × 3). EES was designed to mimic the natural cleansing functions of wetlands to achieve wastewater treatment. The EES system consisted of three tanks with diverse biota, viz., aquatic macrophytes, submerged plants, emergent plants and filter feeders, connected in series. The treated water discharged from EES was re-circulated to the water storage tank to be used in the process.
2.3. Experimental details
Anaerobic sludge from STP-HWSSB, Hyderabad, was used as a parent culture after filtering with stainless steel mesh. The thick filtered inoculum was subjected to heat-shock pretreatment for 120 min at 80 °C–90 °C to suppress the methanogens in the mixed inoculums.30,32 Before inoculation, the inoculum was enriched with designed synthetic media providing 3 g COD per L (glucose) for 48 h at pH 6. A heat-shock pretreated enriched consortium from the inoculum tank was transferred to the acidogenic bioreactor (ABT) before starting the reactor. Initially, 110 kg of food waste (FW) was masticated (stage-I) using a blender. The masticated FW was then transferred to the buffer tank (BT) (stage-II) and mixed with water (left for 12 h under anaerobic conditions). A top mixer was used for homogenous mixing of the content. Periodically, air was sparged to the BT to suppress methanogens. The partially degraded FW in the BT was then transferred to the main ABT (stage-III) using a 1 HP motor. Fluid recirculation in the bioreactor was driven by a 1 HP non-flammable motor with a capacity of 5 m3 h−1. NaOH was dosed into the ABT from the acid/base tank to adjust the pH to 6.5 using a pH sensor. A recirculation pump (1 HP) in an up-flow mode was used for homogenization of the reactor content during the operation.
2.4. Analysis
The performance of the pilot plant system was assessed by analysing the chemical oxygen demand (COD; closed reflux titrimetric method), total volatile fatty acid (VFA), and pH profile. Quantification of the H2 gas produced during the fermentation of FW was carried out by withdrawing 1 mL of gas from the headspace with a gas-tight syringe (Hamilton) followed by injection into a calibrated gas chromatograph (GC: Agilent7890) equipped with a thermal conductivity detector (TCD; OD:1/8, ID: 2 mm, SS Heysep Q column) and argon as a carrier gas (4 mL min−1). The injector and detector were both maintained at 80 °C, and the oven was operated at 100 °C isothermally. The volatile fatty acid profile (acetate, propionate, and butyrate) was analyzed using HPLC (Shimadzu LC20A) employing an RI detector (RID20A; Shimadzu) and a Rezex monosaccharide column (Phenomenex India) by injecting a filtered sample of 20 μl. For elution, a flow rate of 0.5 mL min−1 was used in an isocratic method with filtered ultrapure water as the mobile phase, and the column temperature was maintained constantly at 60 °C in a column oven (RA20A). Dehydrogenase (DH) enzyme activity was estimated by a colorimetric procedure based on the reduction of 2,3,5-triphenyl tetrazolium chloride (TTC).25 Buffering capacity (βmol) was estimated based on acid–base titrations employing an auto-titrator (Mettler Toledo DL50). β was calculated using eqn (1), where C is the concentration of acid or base (N), Vs is the volume of the sample (mL), and m is the slope of the tangent of the curve. |  | (1) |
The hydrogen conversion efficiency (HCE) was calculated by considering the cumulative hydrogen production (CHP, L), organic loading of the substrate (OL, g COD per L), substrate conversion efficiency (CODC, %) and substrate feeding volume (V, L), as depicted in eqn (2).
| 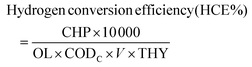 | (2) |
THY is the theoretical H2 yield (466 L H2 per kg COD) based on the acetate pathway from glucose (C6H12O6 + 2H2O → 4H2 + 2CH3COOH + 2CO2; 667.24 g COD CH3COOH per kg COD + 332.29 g COD H2 per kg COD; 466 L H2 per kg COD).
2.5. Metagenomic analysis
Microbial diversity analysis of parent inoculum (untreated) and acidogenic consortia (heat-shock pretreated) at the end of the experiment was studied. Genomic DNA (gDNA) extraction was performed by using a NucleoSpin® Soil kit (Macherey-Nagel GmbH & Co, Germany). Agarose gel electrophoresis (1%) was used to check the presence of gDNA. Universal primers [forward primer, 341FGC, 5′ – AGG CCT AAC ACA TGC AAG TC – 3′; reverse primer 517R, 5′ – ATT ACC GCG GCT GCT GG – 3′; GC clamp was added to the forward primer related to the conserved region (V3) of the 16S rRNA gene] were used for PCR amplification using a thermal cycler (Eppendorf, Germany). Further amplified PCR products were checked for concentration using Nanodrop (Thermo) and purified by a PCR purification kit (QIAquick, Qiagen, USA). Denaturing gradient gel electrophoresis (DGGE) with SYBR stain was used with polyacrylamide gel. Each DGGE band was considered as an OTU for analysis. The amplified bands were partially sequenced and compared with the NCBI GenBank database using the BLAST server (NCBI BLAST).
2.6. Life cycle analysis – environmental sustainability
Life cycle analysis (LCA; ISO 14040:2006) was employed to study the environmental impact of both the standalone and integrated biorefinery processes separately with defined system boundaries (Fig. 2) using SimaPro9.0.0.49 software. The primary data, i.e., the data pertaining to the raw materials, chemicals, water, and energy, were used as direct input, whereas the secondary data of processes such as production of reactants, nutrients and water were available in the Ecoinvent 3.4 database provided with the SimaPro software.33 The influence of both processes was evaluated on the major environmental impact categories such as global warming potential, ozone depletion, health factors, resource depletion, and ecotoxicity, along with other impacts. A gate-to-gate LCA approach was considered in this study to evaluate the respective environmental impacts.23,36 The analysis followed the avoided-products approach, where acceptable allocations were granted to the by-products of both processes. This approach facilitates the sharing of impact burdens among the product and the by-products (H2 and VFA, respectively). The allocations and the inventory data employed for conducting LCA are provided in Table 1. The functional unit for the study was set as one kilogram (kg) of H2 production. The lifecycle impact assessment (LCIA) was performed using the Impact 2002 + method considering various mid-point impact categories and endpoint impact categories.35 Uncertainty analysis was performed to compare the actual and anticipated results, which ultimately is crucial in accounting the LCA results. Monte Carlo simulation was employed in the uncertainty analysis with SimaPro software. A thousand runs were carried out to obtain the analysis at a 98% confidence interval by using the IMPACT 2002 + V2.15/IMPACT 2002 + method for both the processes (standalone and integrated approaches). Furthermore, a comparative study was conducted for standalone and integrated biorefinery approaches with established hydrogen production technologies such as ammonia cracking (M1), steam reforming of methane (M2), the sodium amalgam (mercury cell electrolysis; decomposer cell) method (M3), and diaphragm technologies (M4). The comparative analysis was performed in the confines of the obtainable data of processes M1 to M4 and features available in the Simapro LCA software.
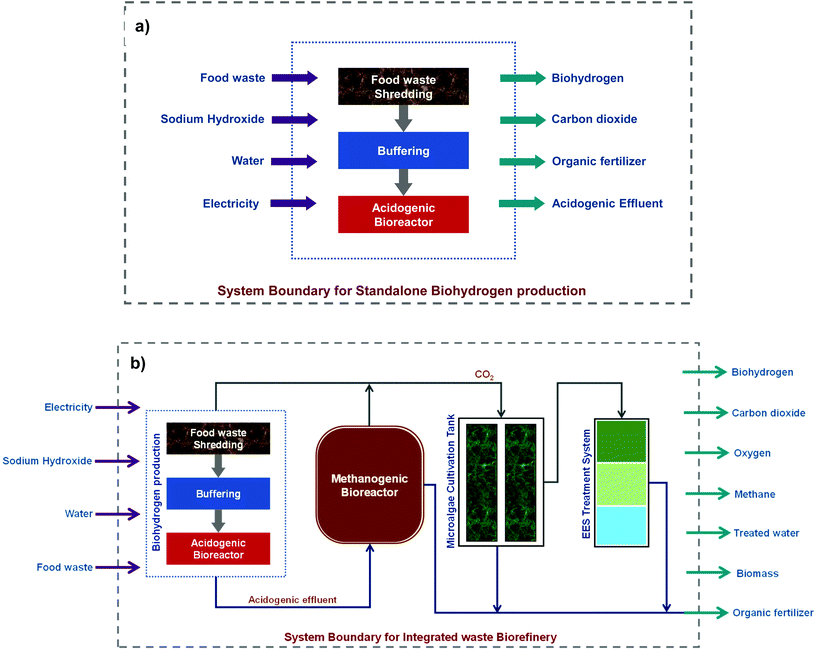 |
| Fig. 2 (a) System boundary for standalone bio-H2 and (b) the integrated biorefinery consisting of multiple unit operations for biobased product recovery and wastewater treatment. | |
Table 1 Inventory data employed for performing LCA
Input to the system |
Standalone approach |
Integrated biorefinery approach |
Food waste |
30 kg |
30 kg |
Sodium Hydroxide |
0.07 kg |
0.12 kg |
Tap Water |
1500 L |
1500 L |
Electricity |
4 Kwh |
7 Kwh |
Anaerobic Sludge |
25 kg |
25 kg |
Acetic acid |
— |
15.47 kg |
Butyric acid |
— |
11.41 kg |
Propionic acid |
— |
9.94 kg |
CO2 |
— |
3.6 kg |
Output from the system
|
|
|
Allocation (%) |
|
Allocation (%) |
Biohydrogen |
1 kg |
45 |
1 kg |
45 |
CO2 |
3.6 kg |
14 |
— |
— |
Acetic acid |
15.47 kg |
24 |
— |
— |
Butyric acid |
11.41 kg |
12 |
— |
— |
Propionic acid |
9.94 kg |
5 |
— |
— |
Algal biomass |
— |
— |
1 kg |
13 |
Treated water |
— |
— |
700 L |
7 |
O2 |
— |
— |
1 kg |
35 |
3. Results and discussion
3.1. Pilot plant (10 m3) performance
The pilot plant was initially operated at a lower organic load (FW: 5 g COD per L) for adaptation of the biocatalyst and for biofilm formation. Subsequently, the system was operated at a higher organic load (50 g COD per L). A cumulative bio-H2 production (CHP) of 54
288 L (H2: 4.5 kg) with a retention time of 48 h was observed with an organic load of 50 g COD per L, accounting for an H2 composition of 61% along with 39% CO2 co-generation (Fig. 3). In this study, CH4 evolution was not observed, confirming the suppression of methanogenic activity. Pretreatment of inoculum is crucial prior to startup of the acidogenic process to suppress the methanogens in the mixed culture.18,32 Heat-shock pretreatment was employed for terminating H2 consumers in the mixed culture. However, a complete suppression of methanogenic activity is difficult despite pretreatment because acetoclastic and methanoclastic methanogenic bacteria (MB) survive on weak acids/H2. Thus air was bubbled into the reactor for a period of 5 minutes (once in 12 h) in order to inactivate the functional activity of the MB.30
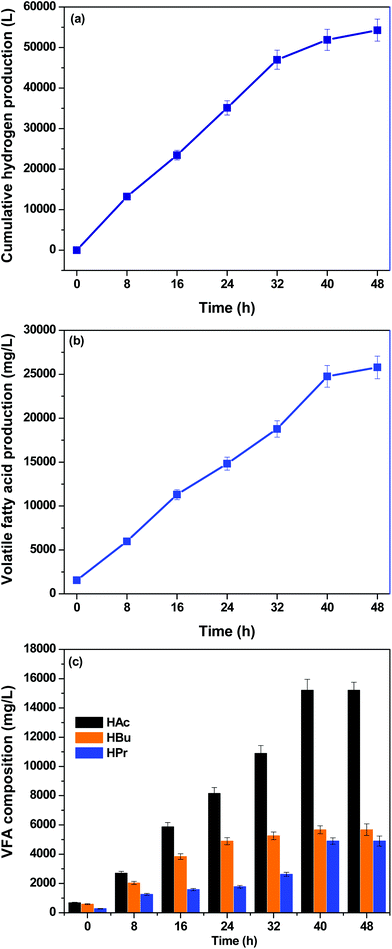 |
| Fig. 3 (a) Biohydrogen production during acidogenic fermentation of food waste at an organic load of 50 g COD per L; (b) total volatile fatty acid production; (c) composition of individual fatty acids in the total VFA derived from food waste at 50 g COD per L along with biohydrogen. | |
Short-chain carboxylic acid production accounted for 271.33 kg COD by the end of the cycle operation, along with bio-H2 production (Fig. 3). Compositional analysis revealed the presence of acetic (C2H4O2; HAc), propionic (C3H6O2; HPr) and butyric (C4H8O2; HBu) acids. HAc and HBu acid fermentation produced 4 mol H2 per mol glucose and 2 mol H2 per mol glucose, respectively; these are the two main metabolic routes by which molecular H2 is generated in dark fermentation (eqn 3 and 4). Production of HAc and HBu was higher compared to HPr at both 24 h and 48 h of the cycle period. Production of HAc increased to 8.15 g L−1 (24 h) from 0.68 g L−1 (0 h), which was further maximized to 15.21 g L−1 (48th h). Accumulation of HBu was higher during 8–24 h of operation, with the highest production of 4.89 g L−1 (24 h) and 5.6 g L−1 (48 h). In contrast, biosynthesis of HPr (1.5 g L−1) was low up to 24 h of the cycle period compared to the other two fatty acids. The HPr concentration was doubled in the next 24 h of fermentation (3.12 g L−1), with a total accumulation of 4.89 g L−1.
|  | (3) |
|  | (4) |
| C6H12O6 + 2H2 → 2CH3CH2COO− + 2H2O + 2H+ | (5) |
H2 production showed a good correlation with VFA production;16 interestingly, no consumption of VFA was seen during pilot plant operation. The efficiency of a system towards bioconversion of the substrate to VFAs was expressed in terms of acidification potential (AP). The AP was derived based on the COD equivalents of the individual fatty acids (C2H4O2: 1.07 g COD per g; C3H6O2: 1.51 g COD per g; C4H8O2: 1.82 g COD per g).15,16 With the production of 16.27 g COD per L of HAc followed by 10.26 g COD per L and 7.4 g COD per L of HBu and HPr, respectively, the acidification potential reached 67.91%. With the distribution of fatty acids, HAc alone contributed to 32.56% of the acidification, followed by 20.54% and 14.88% by HBu and HPr, respectively. The pilot-scale operation showed >60% AP, which is important in terms of production of renewable chemicals (HAc, HBu and HPr).
The pH of the influent was adjusted to 6.5 prior to feeding. Initial mild acidic pH favors acidogenic function by suppressing the methanogenic activity.32 The bio-H2 production correlated well with the pH changes during acidogenic fermentation (Fig. 4a). Controlled pH can be adopted throughout the process; however, it soon becomes economically unfeasible due to the large consumption of chemicals. Further dehydrogenase enzyme (DH) activity during the operation was monitored at every 8 h of operation. DH is fundamental to the biological redox reactions supported by redox mediators (NAD+, FAD+). At the 8th h, the DH activity was 1.44 μg mL−1, which further increased to 2.88 μg mL−1 (24 h) and 4.68 μg mL−1 (32 h). The dynamics of the fermentation process were further assessed in terms of substrate conversion/degradation, which showed a significant increment with fermentation time (Fig. 4b). The biohydrogen conversion efficiency (HCE) was calculated to assess the process efficiency, which specifically represented the amount of bio-H2 being evolved from the substrate degraded. The HCE based on waste-derived H2 was 57.38% by the end of the fermentation.
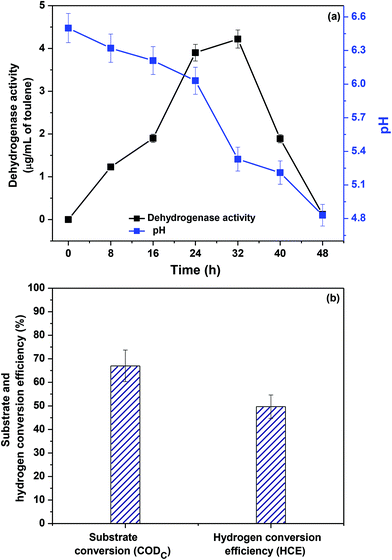 |
| Fig. 4 (a) Dehydrogenase activity and pH change recorded with respect to time during food waste fermentation; (b) substrate conversion (CODC) and biohydrogen conversion efficiency (HCE) recorded during acidogenic fermentation of food waste. | |
3.2. Biorefinery system
The acidogenic process left 271.33 kg of COD unutilized in the acidogenic outlet (AO), which is fatty acid-rich (Table 2). Although the H2 produced in this study is of biological origin from renewable feedstock, sole recovery and utilization of bio-H2 leaving behind unutilized carbon as waste will be unsustainable. There is a possibility of recovering fatty acids as platform chemicals from AO, which will have an influence on economics as well as on environmental sustainability.17 In this study, fatty acids were not recovered; hence, disposal of the AO as waste creates a substantially negative impact on the whole process, and this scenario compelled us to give value addition to AO by refining it in a waste biorefinery unit analogous to a petro-refinery.3 A biorefinery facility integrated with bio-H2 was designed with an aim to utilize the fatty acid-rich/unutilized carbon in AO and CO2 for the production of multiple biobased compounds in a carbon-neutral approach. Waste-based biorefineries (WB) have been evolving into more resource-oriented platforms.3,18,24,36–40 The concept of biorefinery was successfully documented in this study experimentally by integration of various bioprocesses which accounted for efficient residual carbon conversion (more than 95%) with resource recovery in the form of methane (methanogenesis: MB), algal biomass and oxygen (algal raceway pond: ARP) and treated water (ecological engineering wetland systems: EES). The first integrated methanogenesis was intended to facilitate the conversion of organics to biomethane. The produced methane during methanogenesis was 32
454 L, which can compensate the majority of energy consumption of the pilot plant. The methane yield from AO was due to the presence of high amounts of end products (mostly HAc), which are simpler to break down by methanogens compared to complex organic waste.41–43 However, simpler substrate availability is an added advantage, as bacteria skips a few steps (hydrolysis and acidogenesis/acetogenesis) and proceeds to the subsequent step, directly resulting in product formation (eqn (6)).44 | CH3COO− + H2O → CH4 + HCO3−; ΔG°′ = −31 kJ per reaction | (6) |
Table 2 Consolidated performance data pertaining to standalone and integrated biorefinery system
Bioprocess |
RT (h) |
Bioreactor (m3) |
H2 (L) |
H2 (%) |
VFA |
CODC (%) |
HCE (%) |
DH (μg mL−1 of toluene) |
In situ buffering capacity (βmol) |
Biogenic CO2 (L) |
Production (kg COD) |
Composition (kg COD) |
Initial |
Final |
HAc |
HBu |
HPr |
RT = retention time; HAc = acetic acid; HBu = butyric acid; HPr = propionic acid; OL = organic load; CODC = substrate conversion efficiency; HCE = hydrogen conversion efficiency; DH = dehydrogenase; MCE = methane conversion efficiency; VFA = volatile fatty acid. |
Acidogenesis (AB) |
48 |
10 |
54 288 |
61 |
0.002 |
271.33 |
129.68 |
82.40 |
59.25 |
58 |
57.38 |
4.68 |
0.26 |
10 374 |
Methanogenesis (MB) |
60 |
8 |
CH
4
(L)
|
CH
4
(%)
|
Initial
|
Final
|
Consumption (kg COD)
|
CODC (%)
|
MCE (%)
|
Biogenic CO
2
(L)
|
32 454 |
87 |
237.41 |
56.98 |
24.51 |
15.57 |
11.20 |
76 |
66.41 |
|
|
4531.8 |
Photosynthesis (ARP) |
120 |
6 |
Biomass (kg)
|
O
2
(evolution, kg)
|
Initial
|
Final
|
Consumption (kg COD)
|
CODC (%)
|
CODC (after treatment, kg COD)
|
Utilization of Biogenic CO
2
(L)
|
2.4 |
3.7 |
56.98 |
3.42 |
1.57 |
1.70 |
1.02 |
93 |
4.28 |
|
|
10 200 |
Ecological Engineered System (EES) |
24 |
3 |
1.2 |
1.2 |
3.42 |
0.074 |
0.032 |
0.020 |
0.014 |
95.7 |
0.074 |
|
|
2980 |
The higher CH4 fraction in the total biogas can be attributed to the direct conversion of VFA to CH4. Compared to bio-H2 production by the acidogenesis processes, methanogenesis is more efficient towards substrate conversion (>70%; final COD of 56.98 kg COD) during this stage of operation. The residual carbon of the methanogenic bioreactor is fed to raceway ponds for the cultivation of microalgae. Microalgae in mixotrophic mode convert both organic and inorganic carbon in the presence of sunlight.45 Microalgae have a fast growth rate and biomass productivity compared to other energy crops, as this biomass is doubled in 24–30 h.46 The microalgae using the residual carbon present in the methanogenic effluent produced 2–3 ± 1.2 kg biomass. Earlier studies showed that fatty acid-rich acidogenic effluent from bio-H2 production can be a potential feedstock for the valorisation of algal biomass.47 A study on the utilization of acidogenic effluent as the main substrate for mixotrophic algal cultivation showed carbon recoveries of more than 60%. Algae have the potential to capture and convert atmospheric CO2 into edible biomass.48,49 The production of 1 kg of algal biomass requires about 1.83 kg of CO2.50 CO2 utilization by algae indicates its potential towards carbon neutrality. After harvesting the algal biomass, the effluent is then passed through an ecological engineered system (EES) designed to mimic the natural cleansing functions of wetlands to enable wastewater treatment.51–53 Three tanks of the EES system consisted diverse biota, viz., aquatic macrophytes, submerged plants, emergent plants and filter feeders connected in series. The waste biorefinery unit finally reduced the COD concentration of the water in the range of 0.074 kg COD (Table 2). The treated water from the EES is again re-circulated to the water storage tank to use in the process. The waste biorefinery has been a potential solution to address energy, chemicals, materials and clean water needs in a sustainable way, preserving the ecosystem and natural capital.3,18
3.3. Metagenomic profile
At the end of the pilot plant operation, culture was collected from the main outlet of the ABT to understand the diversity of the microbiome in comparison with the parent culture. The relative abundance of bacterial diversity at the phylum and genus levels is depicted in Fig. 5. Among the total number of 18 operational taxonomic units (OTUs) observed, 15 were present in the parent inoculum and 13 were present in the bioreactor outlet. From the number of OTUs, it can be inferred that parent inoculum showed higher diversity. Both the samples shared 10 OTUs in common. The parent inoculum had 5 unique OTUs compared to 3 OTUs of the ABT. The unique OTUs of the parent inoculums are related to non-acidogenic groups, whereas the unique OTUs of ABT are related to acidogenic bacteria; this indicates the effectiveness of the heat-shock treatment and reactor operation. The ABT diversity showed the presence of firmicutes (54.55%) as the dominant bacterial phylum, followed by Proteobacterium (25%), Actinobacterium (9.1%), Bacteroides (6.2%), etc. as significant. An abundance of firmicutes favours acidogenesis.32 The enriched firmicutes are mostly acidogens, such as Clostridium acetobutylicum, Clostridium propionicum, Clostridium beijerinckii, Clostridium aciditolerans, Bacillus amyloliquefaciens, and Lactobacillus sp., which are well known for their ability to produce fatty acids.54,55 Production and accumulation of high concentrations of short-chain fatty acids can be positively correlated with the dominance of Clostridium sps.. Clostridia were previously reported to be a major part of the microbial consortium, producing butyrate, acetate and propionate along with bio-H2 under anaerobic conditions to support their enrichment.56,57 The evolution of efficient acidogenic communities in the ABT demonstrated that the operating conditions also have a strong selective and reproducible effect on the structure and function of the microbial community. On the other side, Bacteroidetes play an important role in the hydrolysis and fermentation of macromolecular organic compounds (e.g. proteins, carbohydrates).58 Production of propionic acid can be directly related to the presence of Propionibacterium, which is a Gram-positive bacterial group in the subdivision of Actinomycetes. This anaerobic, rod-shaped bacterium is known for its unique metabolic ability to synthesize propionic acid using transcarboxylase enzyme.59
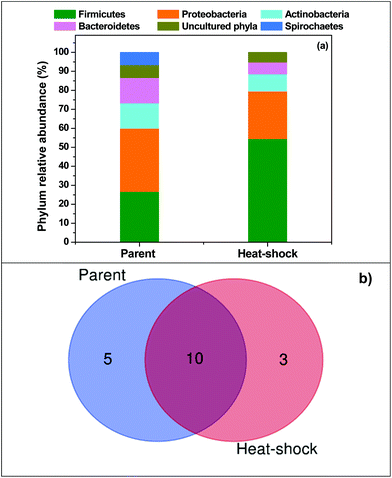 |
| Fig. 5 (a) Relative abundance of various OTUs in the heat-shock pretreated inoculum in the 10 m3 pilot plant and the parent inoculum; (b) Venn diagram illustrating the unique and shared OTUs within the heat-shock pretreated and parent inoculum. | |
3.4. Life cycle analysis
3.4.1. Standalone process.
Life cycle analysis (LCA) tools will help quantify the impact of a process/product on the environment throughout its life cycle; hence, it provides awareness to help inventors to take action to mitigate those impacts by adopting environmentally sustainable processes.21Table 3 depicts the quantified impact of input materials (food waste, NaOH, tap water and electricity) towards various environmental categories for the production of 1 kg of biohydrogen in both standalone and integrated approaches. Among all the inputs of the standalone approach, electricity showed the greatest contribution (around 95% of all inputs), mostly towards impact categories such as aquatic ecotoxicity: 19.32 (kg TEG water) > non-renewable energy: 4.84 (MJ primary) > terrestrial ecotoxicity: 4.42 (kg TEG soil) > global warming: 0.39 kg CO2 eq. (Table 3). The electricity was supplied from the grid (Southern India), which is mainly produced from thermal power stations with non-renewable feedstocks. The electricity production in India is categorized by the highest share of fossil fuel-derived power generation, with 73% coal followed by 4.5% natural gas and 0.5% diesel oil. In addition, nuclear, hydroelectric and other renewable energy sources contribute 3%, 10.5% and 8.5%, respectively.60 Combustion emissions play a crucial role in affecting air quality, environmental balance, climate change, and human health by the concentration of anthropogenic carbon dioxide. However, using food waste as the feedstock reduced the overall impacts of non-renewable energy (−67.2 MJ primary) and global warming (−4.21 kg CO2 eq.) by avoiding the utilization of conventional fossil-based feedstock. This also contributed positively to the mineral extraction category due to the utilization of a renewable resource as a feedstock (instead of non-renewable sources).
Table 3 Impacts of processes (standalone; ST and integrated waste biorefinery; WB) on various environmental impact categories (IMPACT 2002+ LCIA method)
Impact category |
Unit |
Food waste |
NaOH |
Tap water |
Electricity, (low voltage) |
Total |
ST (A1) |
WB (B1) |
ST (A2) |
WB (B2) |
ST (A3) |
WB (B3) |
ST (A4) |
WB (B4) |
ST (A1 + A2 + A3 + A4) |
WB (B1 + B2 + B3 + B4) |
Carcinogens |
kg C2H3Cl eq. |
0 |
0 |
2.31 × 10−6 |
6.60 × 10−7 |
4.26 × 10−5 |
1.22 × 10−5 |
0.00185 |
0.00056 |
0.0019 |
0.00054 |
Non-carcinogens |
kg C2H3Cl eq. |
0.00044 |
0.00013 |
9.49 × 10−5 |
2.71 × 10−5 |
6.80 × 10−5 |
1.94 × 10−5 |
0.00802 |
0.0022 |
0.0086 |
0.00246 |
Respiratory inorganics |
kg PM2.5 eq. |
0.00047 |
0.000135 |
4.38 × 10−6 |
1.25 × 10−6 |
4.16 × 10−6 |
1.19 × 10−6 |
0.00079 |
0.00022 |
0.0012 |
0.00036 |
Ionizing radiation |
Bq C-14 eq. |
0 |
0 |
0 |
0 |
1.0299985 |
0.294 |
1.462 |
0.4179 |
2.49271 |
0.7122 |
Ozone layer depletion |
kg CFC-11 eq. |
0 |
0 |
6.84 × 10−10 |
1.96 × 10−10 |
1.61 × 10−9 |
4.61 × 10−10 |
4.08 × 10−9 |
1.16 × 10−9 |
6.37 × 10−9 |
1.82 × 10−9 |
Respiratory organics |
kg C2H4 eq. |
−0.000347 |
−9.92 × 10−5 |
9.52 × 10−7 |
2.72 × 10−7 |
8.07 × 10−7 |
2.31 × 10−7 |
3.34 × 10−5 |
9.55 × 10−6 |
−0.000312 |
−8.91 × 10−5 |
Aquatic ecotoxicity |
kg TEG water |
0.034 |
0.0097 |
0.5601 |
0.160 |
0.5149 |
0.147 |
19.328 |
5.5223 |
20.437 |
5.8392 |
Terrestrial ecotoxicity |
kg TEG soil |
0.085 |
0.025 |
0.0022 |
0.00065 |
0.1091 |
0.0311 |
4.4203 |
1.262 |
4.6170 |
1.3191 |
Terrestrial acid/nutri |
kg SO2 eq. |
0.109 |
0.031 |
9.88 × 10−5 |
2.82 × 10−5 |
0.00013 |
4.32 × 10−5 |
0.0053 |
0.0015 |
0.1155 |
0.0330 |
Land occupation |
m2 org.arable |
0 |
0 |
0 |
0 |
0.00015 |
4.40 × 10−5 |
0.00356 |
0.00101 |
0.0037 |
0.0010 |
Aquatic acidification |
kg SO2 eq. |
0.012 |
0.00344 |
4.65 × 10−5 |
1.33 × 10−5 |
2.63 × 10−5 |
7.52 × 10−6 |
0.00162 |
0.0005 |
0.0137 |
0.0039 |
Aquatic eutrophication |
kg PO4P-lim |
0 |
0 |
2.92 × 10−8 |
8.34 × 10−9 |
1.75 × 10−6 |
4.99 × 10−7 |
8.86 × 10−5 |
2.53 × 10−5 |
9.04 × 10−5 |
2.58 × 10−5 |
Global warming |
kg CO2 eq. |
−4.61 |
−1.32 |
0.0050 |
0.00144 |
0.00449 |
0.0012 |
0.39813 |
0.1137 |
−4.2110 |
−1.203 |
Non-renewable energy |
MJ primary |
−72.42 |
−20.69 |
0.0831 |
0.023 |
0.2157 |
0.0616 |
4.848 |
1.3852 |
−67.281 |
−19.22 |
Mineral extraction |
MJ surplus |
−0.0059 |
−0.0012 |
0 |
0 |
0.000246 |
7.03 × 10−5 |
0.00413 |
0.0011 |
−0.0016 |
−0.0004 |
The influence on impact categories, namely respiratory organics, mineral extraction, non-renewable energy and global warming, was represented with a negative sign, which indicates the comprehensive and environmentally positive impact of the process with respect to those impact categories. However, the impact on aquatic ecotoxicity (20.43 kg TEG water) and terrestrial ecotoxicity (4.61 kg TEG soil) was still higher, possibly due to the substantial production of volatile fatty acids (in the effluent) in the form of acetic, propionic and butyric acids. These weak acids can be extracted from the fermentation liquid and have their own application in the market, thus lowering the impact on aquatic ecotoxicity and terrestrial ecotoxicity. Despite that, the VFA mixture was used as feedstock for the production of other biobased products (methane, algal biomass, O2 and treated water) in the integrated biorefinery approach.
3.4.2. Integrated biorefinery process.
As discussed, the standalone approach was upgraded to an integrated waste biorefinery process and was compared with the standalone approach and other existing hydrogen production technologies. The system boundary included various unit operations such as bio-methanation, algal cultivation and an ecological engineered system (EES) to utilize maximum resources available in the system and to improve the process sustainability. Inputs such as VFA and CO2 in the integrated process were not considered as an additional input to the system, as they were produced in the acidogenesis step and consumed in methanogenesis and algal cultivation steps, respectively (produced and consumed within the system). The electricity showed the highest influence on impact categories, even in the integrated process. The integrated approach resulted in −1.203 kg CO2 eq. of global warming potential (GWP), which is higher than that of the standalone approach. Because the integrated waste biorefinery approach includes additional unit operations, where more energy is required, the net GWP was greater. However, the influence on the remaining impact categories was considerably reduced. Owing to the utilization of CO2 and VFA generated in acidogenesis for the subsequent bioprocesses, the impacts on various categories were reduced markedly. The influence on non-carcinogens, terrestrial ecotoxicity, aquatic eutrophication and aquatic ecotoxicity was reduced by 3.5 fold, which may be due to maximal resource utilization with limited emissions. As an integrated approach, bio-sequestration of CO2 by algae as a unit operation also had an influence in reducing the net GWP. Integration of bioprocesses favored a significant reduction in global warming, dependency on non-renewable energy and mineral extraction. Based on the results, it could be stated that implementing bio-H2 production with a biorefinery have effectively reduced the load on the environment along with multiple bio-based products.
3.4.3. Uncertainty analysis.
Further, sensitivity analyses (uncertainty analyses) were individually performed for the standalone and integrated approaches. The uncertainty graphs with respect to the GWP category are depicted in Fig. 6. The uncertainty of the data was quantified according to the guidelines of IPCC as the ratio of the standard deviation and the mean value for an environmental impact category.34 The quantified uncertainty values of the aquatic acidification, aquatic ecotoxicity, global warming, land occupation, mineral extraction, non-renewable energy, ozone layer depletion, respiratory inorganics, respiratory organics, terrestrial acidification/nitrification and terrestrial ecotoxicity impact categories of both the standalone and integrated approaches were observed to be less than or equal to 0.3; this demonstrates the quality of the provided experimental data with good probability, which assists the repeatability as well as the reproducibility of the results.
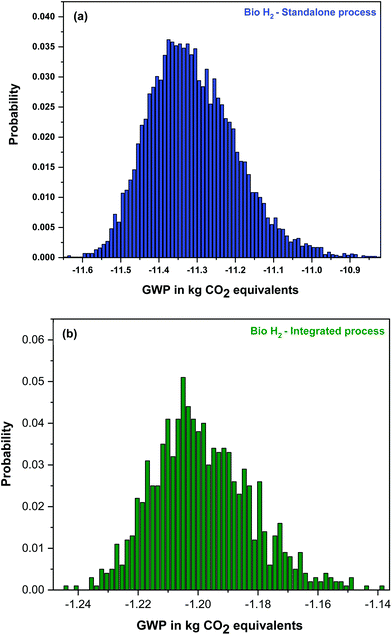 |
| Fig. 6 Uncertainty analysis for the GWP impact categories of (a) bio-H2 standalone and (b) an integrated (waste biorefinery) process using the IMPACT 2002 + V2.15/IMPACT 2002 + method at confidence interval of 98%. | |
3.4.4. Comparative impact analysis on the damage/endpoint categories.
Further, the comparative analysis of standalone and integrated biorefinery approaches with the existing conventional H2 technologies has shown interesting and supporting results for biobased and low carbon products. The integrated approach has shown the least influence on respiratory organics, global warming, non-renewable energy and mineral extraction; however, it showed a marginally higher impact on the remaining midpoint categories (Table 4). Specifically, aquatic acidification, aquatic eutrophication, terrestrial ecotoxicity, and respiratory inorganics showed higher impact, which is mainly caused by the power utility in various unit operations. Relative analysis depicted that the method M2 has the highest effect on all the impact categories, except the carcinogens and terrestrial ecotoxicity categories. For producing 1 kg of H2, reformer technology (5.31 kg CO2 eq.) contributed the most towards global warming. The M1 and M2 methods showed no impact on ozone depletion and ionizing radiation. When the bio-H2 standalone process was compared with the M1 and M2 methods, it showed a higher effect on impact categories such as non-carcinogens, terrestrial ecotoxicity and aquatic eutrophication, where the utilization of chemicals, electricity and water was greater. The GWP was relatively low with the standalone process operation compared to all other production processes. The M4 method exhibited much less impact on aquatic ecotoxicity, where the utilization/exploitation of water was minimal.
Table 4 Comparative LCA results of the standalone bio-H2 (ST), integrated biorefinery (WB), and conventional H2 production methods (IMPACT 2002+ LCIA method)
Impact category |
Unit |
Bio-H2 |
Method 1 (M1) |
Method 2 (M2) |
Method 3 (M3) |
Method 4 (M4) |
ST |
WB |
Carcinogens |
kg C2H3Cl eq. |
0.0019 |
0.00055 |
0.026 |
0.00085 |
0.00024 |
0.00029 |
Non-carcinogens |
kg C2H3Cl eq. |
0.0086 |
0.0024 |
2.57 × 10−5 |
7.91 × 10−5 |
0.00178 |
0.0012 |
Respiratory inorganics |
kg PM2.5 eq. |
0.0012 |
0.00036 |
0.00067 |
0.00570 |
0.00102 |
0.0008 |
Ionizing radiation |
Bq C-14 eq. |
2.4927 |
0.7122 |
0 |
0 |
26.447 |
22.09 |
Ozone layer depletion |
kg CFC-11 eq. |
6.37 × 10−9 |
1.82 × 10−9 |
0 |
0 |
2.40 × 10−7 |
2.01 × 10−7 |
Respiratory organics |
kg C2H4 eq. |
−0.00031 |
−8.91 × 10−5 |
0.00013 |
0.00085 |
0.00011 |
0.0001 |
Aquatic ecotoxicity |
kg TEG water |
20.43 |
5.8392 |
5.5511 |
6.5603 |
3.26 |
0.3600 |
Terrestrial ecotoxicity |
kg TEG soil |
4.617 |
1.3191 |
0.0087 |
0.01087 |
2.81 |
0.6435 |
Terrestrial acid/nutri |
kg SO2 eq. |
0.115 |
0.0330 |
0.0136 |
0.1539 |
0.0166 |
0.01427 |
Land occupation |
m2 org.arable |
0.0037 |
0.0011 |
0 |
0 |
0 |
0 |
Aquatic acidification |
kg SO2 eq. |
0.014 |
0.0039 |
0.0035 |
0.02567 |
0.00721 |
0.0061 |
Aquatic eutrophication |
kg PO4P-lim |
9.04 × 10−5 |
2.58 × 10−5 |
3.75 × 10−6 |
4.19 × 10−7 |
1.34 × 10−5 |
1.21 × 10−5 |
Global warming |
kg CO2 eq. |
−4.211 |
−1.203 |
1.3797 |
5.3181 |
1.0307 |
0.8830 |
Non-renewable energy |
MJ primary |
−67.28 |
−19.22 |
73.786 |
89.530 |
19.094 |
16.212 |
Mineral extraction |
MJ surplus |
−0.0016 |
−0.0004 |
2.15 × 10−5 |
0.00072 |
0.00011 |
9.49 × 10−5 |
In the impact 2002 + Lifecycle impact assessment method, the related mid-point categories were consolidated as endpoint or damage categories. The comparative impact analysis of standalone and integrated biorefinery approaches on the damage/endpoint categories is depicted in Fig. 7. Towards the damaging categories, the standalone process showed a higher impact on human health (9.21 × 10−7 DALY) and the ecosystem (0.1617 PDF m2 year) owing to the utilization of chemicals for buffering, electricity generation and the wastewater generated in the process (Fig. 7). However, there was a positive influence on climate change (−4.21 kg CO2 eq.) and resources (−67.28 MJ) owing to the use of food waste as renewable feedstock. In comparison to the standalone process, the effect on the human health-damaging category was significantly reduced (2.63 × 10−7 DALY) in the integrated biorefinery approach, which is the lowest effect compared with the existing methods. The ecosystem quality of the integrated biorefinery approach was slightly higher than that of all the existing methods except M2; this may be due to the higher impacts in the corresponding midpoint categories. Overall, the method M2 showed higher impacts on all the damage categories. Among the conventional H2 production technologies, the M4 method depicted lower impacts on environmental factors. The present study specifies that the production of H2 through biological routes enables low embodied carbon, and eventually the integrated systems facilitate sustainable platforms towards a circular bioeconomy.61
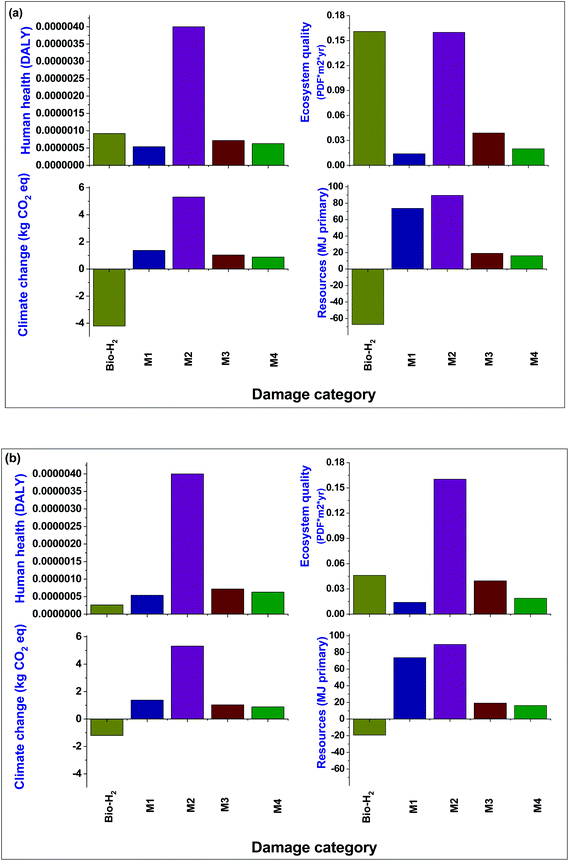 |
| Fig. 7 Comparative lifecycle impact assessment results of (a) the standalone biohydrogen process and (b) integrated waste biorefinery model with respect to the endpoint (damage) categories. | |
4. Conclusions
Upscaling of the acidogenic process from biogenic waste as feedstock was documented for bioenergy and renewable chemicals production, which in turn makes the whole process environmentally sustainable when adopting the biorefinery format. The 10 m3 pilot-scale operation showed an excellent cumulative bio-H2 production of 54.2 m3 along with a total VFA of 271.33 kg COD within 48 h of food waste fermentation. Metagenomic data revealed that the enrichment of firmicutes (55.4%) in the heat-shock pretreated mixed culture significantly enhanced the biosynthesis of short-chain carboxylic acids. Integration of the bioprocess in a circular loop assisted the derivation of additional products in form of bio-CH4 (32
454 L), algal biomass (2.4 kg), oxygen (4.9 kg), and finally treated water (3 m3), with significant utilization of both organic and inorganic carbon produced during acidogenic and methanogenic fermentation. As waste was consumed and valorized during the process, LCA analysis showed a lower impact with respect to global warming, non-renewable energy resources, and mineral extraction. The biorefinery approach facilitated maximum recovery with concomitant reduction in environmental burdens. More significantly, the bio-H2 production process projected a lower cumulative impact for damage category in terms of human health, ecosystem quality, climate change and resources than the traditional H2 production technologies.
Conflicts of interest
There are no conflicts to declare.
Acknowledgements
The authors acknowledge Ministry of New and Renewable Energy (MNRE), Government of India for funding project (103/131/2008-NT) for ‘Biohydrogen pilot plant’ and Council of Scientific and Industrial Research (CSIR), Government of India for funding ‘Waste biorefinery pilot plant’ as part of SETCA Project (CSC-0113; 12th Five Year Plan Project; Chemical Sciences Cluster). LCA part of the work was supported by CSIR-INPROTICS Project (HCP-0011; MLP-0033). OS And RK duly acknowledge the CSIR and UGC for providing fellowship, respectively. The authors wish to thank Director, CSIR-IICT (IICT/Pubs/2019/409) for supporting the research.
References
- T. M. Gür, Energy Environ. Sci., 2018, 11, 2696–2767 RSC.
- A. Sgobbi, W. Nijs and R. De Miglio, Int. J. Hydrogen Energy, 2015, 41, 1–17 Search PubMed.
- S. Venkata Mohan, G. N. Nikhil, P. Chiranjeevi, C. Nagendranatha Reddy, M. V. Rohit, A. N. Kumar and O. Sarkar, Bioresour. Technol., 2016, 215, 2–12 CrossRef CAS.
- DOE, 2018, https://www.nrel.gov/docs/fy19osti/73353.pdf (Accessed on 02 October, 2020).
- DST, 2020, https://dst.gov.in/sites/default/files/Country%20status%20report%20final%20Hydrogen.pdf (Accessed on 04 October, 2020).
- EU, 2020, https://op.europa.eu/en/publication-detail/-/publication/5602f358-c136-11ea-b3a4-01aa75ed71a1/language-en (Accessed on 18 October, 2020).
- I. Dincer, Int. J. Hydrogen Energy, 2012, 37(2), 1954–1971 CrossRef CAS.
- H. Tian, J. Li, M. Yan, Y. W. Tong, C. Wang and X. Wang, Appl. Energy, 2019, 256, 113961 CrossRef CAS.
- T. Weide, R. E. H. Regalado, E. Brügging, M. Wichern and C. Wetter, Chem. Eng. Technol., 2020, 43(8), 1578–1587 CrossRef CAS.
- C. Y. Lin, T. M. L. Nguyen, C. Y. Chu, H. J. Leu and C. H. Lay, Renewable Sustainable Energy Rev., 2018, 82, 4215–4220 CrossRef CAS.
- A. Fasolini, R. Cucciniello, E. Paone, F. Mauriello and T. Tabanelli, Catalysts, 2019, 9(11), 917 CrossRef CAS.
- P. Nikolaidis and A. Poullikkas, Renewable Sustainable Energy Rev., 2017, 67, 597–611 CrossRef CAS.
- J. F. Soares, T. C. Confortin, I. Todero, F. D. Mayer and M. A. Mazutti, Renewable Sustainable Energy Rev., 2020, 117, 109484 CrossRef CAS.
- J. Baeyens, H. Zhang, J. Nie, L. Appels, R. Dewil, R. Ansart and Y. Deng, Renewable Sustainable Energy Rev., 2020, 131, 110023 CrossRef CAS.
- R. Moscoviz, E. Trably, N. Bernet and H. Carrère, Green Chem., 2018, 20(14), 3159–3179 RSC.
- S. Dahiya, O. Sarkar, Y. V. Swamy and S. Venkata Mohan, Bioresour. Technol., 2015, 182, 103–113 CrossRef CAS.
- J. Iglesias, I. Martínez-Salazar, P. Maireles-Torres, D. Martin Alonso, R. Mariscal and M. López Granados, Chem. Soc. Rev., 2020, 49, 5704–5771 RSC.
- G. A. Martinez, S. Rebecchi, D. Decorti, J. M. Domingos, A. Natolino, D. Del Rio, L. Bertin, C. Da Porto and F. Fava, Green Chem., 2016, 18(1), 261–270 RSC.
- S. J. Burnley, Detritus, 2019, 5, 150–162 Search PubMed.
- A. A. Koutinas, A. Vlysidis, D. Pleissner, N. Kopsahelis, I. Lopez Garcia, I. K. Kookos, S. Papanikolaou, T. H. Kwan and C. K. Lin, Chem. Soc. Rev., 2014, 43, 2587 RSC.
- R. Katakojwala and S. Venkata Mohan, Curr. Opin. Green Sustainable Chem., 2020, 100392 Search PubMed.
- X. Lim, ACS Cent. Sci., 2019, 5(2), 203–205 CrossRef CAS.
- S. Dahiya, R. Katakojwala, S. Ramakrishna and S. Venkata Mohan, Mater. Circ. Econ., 2020, 2(1), 1–28 Search PubMed.
- M. K. Awasthi, S. Sarsaiya, A. Patel, A. Juneja, R. P. Singh, B. Yan, S. K. Awasthi, A. Jain, T. Liu, Y. Duan, A. Pandey, Z. Zhang and M. J. Taherzadeh, Renewable Sustainable Energy Rev., 2020, 127, 109876 CrossRef.
- G. Mohanakrishna, R. K. Goud, S. Venkata Mohan and P. N. Sarma, Int. J. Hydrogen Energy, 2010, 35(2), 533–541 CrossRef CAS.
- K. Y. Show, Z. P. Zhang, J. H. Tay, D. T. Liang, D. J. Lee, N. Ren and A. Wang, Int. J. Hydrogen Energy, 2010, 35(24), 13350–13355 CrossRef CAS.
- S. Venkata Mohan, P. Chiranjeevi and G. Mohanakrishna, Int. J. Hydrogen Energy, 2011, 37(4), 3130–3141 CrossRef.
- L. Zhang, Y. W. Lee and D. Jahng, Bioresour. Technol., 2011, 102(8), 5048–5059 CrossRef CAS.
- P. Mishra, S. Roy and D. Das, Bioresour. Technol., 2015, 198, 593–602 CrossRef CAS.
- O. Sarkar and S. Venkata Mohan, Bioresour. Technol., 2017, 242, 68–76 CrossRef CAS.
- K. Lokesh, A. S. Matharu, I. K. Kookos, D. Ladakis, A. Koutinas, P. Morone and J. Clark, Green Chem., 2020, 22(3), 803–813 RSC.
- S. Venkata Mohan, V. Lalit Babu and P. N. Sarma, Bioresour. Technol., 2008, 99(1), 59–67 CrossRef CAS.
- Ecoinvent database v3.4, 2017, https://www.ecoinvent.org/database/older-versions/ecoinvent-34/ecoinvent-34.html (Accessed from SimaPro LCA tool in January 2020).
- R. Katakojwala and S. Venkata Mohan, J. Cleaner Prod., 2020, 249, 119342 CrossRef CAS.
- O. Jolliet, M. Margni, R. Charles, S. Humbert, J. Payet, G. Rebitzer and R. Rosenbaum, Int. J. Life Cycle Assess., 2003, 8(6), 324 CrossRef.
- Y. Jiang, H. D. May, L. Lu, P. Liang, X. Huang and Z. J. Ren, Water Res., 2019, 149, 42–55 CrossRef CAS.
- J. J. Bozell and G. R. Petersen, Green Chem., 2010, 12, 539–554 RSC.
- F. Cherubini, Energy Convers. Manage., 2010, 51, 1412–1421 CrossRef CAS.
- A. A. Rosatella, S. P. Simeonov, R. F. M. Frade and C. A. M. Afonso, Green Chem., 2011, 13, 754–793 RSC.
- M. Lucarini, A. Durazzo, A. Romani, M. Campo, G. Lombardi-Boccia and F. Cecchini, Molecules, 2018, 23, 1888 CrossRef.
- F. A. M. De Bok, C. M. Plugge and A. J. M. Stams, Water Res., 2004, 38(6), 1368–1375 CrossRef CAS.
- S. G. Shin, G. Han, J. Lim, C. Lee and S. Hwang, Water Res., 2010, 44(17), 4838–4849 CrossRef CAS.
- G. Mohanakrishna and S. Venkata Mohan, Appl. Energy, 2013, 107, 244–254 CrossRef CAS.
- P. Chiranjeevi and S. Venkata Mohan, Bioresour. Technol., 2017, 242, 191–196 CrossRef CAS.
- B. Gilbert-López, J. A. Mendiola, J. Fontecha, L. A. M. van den Broek, L. Sijtsma, A. Cifuentes, M. Herrero and E. Ibáñez, Green Chem., 2015, 17, 4599–4609 RSC.
- F. B. Metting, J. Ind. Microbiol. Biotechnol., 1996, 17, 477–489 CrossRef CAS.
- S. Venkata Mohan and M. Prathima Devi, Bioresour. Technol., 2012, 123, 627–635 CrossRef CAS.
- S. Lage, Z. Gojkovic, C. Funk and F. G. Gentili, Energies, 2018, 11, 664 CrossRef.
- Y. Yang, H. Zhong, R. He, X. Wang, J. Cheng, G. Yao and F. Jin, Green Chem., 2019, 21, 1247–1252 RSC.
- R. Slade and A. Bauen, Biomass Bioenergy, 2013, 53, 29–38 CrossRef.
- J. C. Weissman, R. P. Goebel and J. R. Benemann, Biotechnol. Bioeng., 1988, 31(4), 336–344 CrossRef CAS.
- G. Mohanakrishna, P. Chiranjeevi, P. Dinakar and P. N. Sarma, Bioresour. Technol., 2010, 101, 3363–3370 CrossRef.
- D. K. Yeruva, P. Ranadheer, A. Kiran Kumar and S. Venkata Mohan, npj Clean Water, 2019, 2, 13 CrossRef.
- J. Moestedt, M. Westerholm, S. Isaksson and A. Schnürer, Bioengineering, 2020, 7(1), 3 CrossRef CAS.
- S. Venkata Mohan, L. Agarwal, G. Mohanakrishna, S. Srikanth, A. Kapley, H. J. Purohit and P. N. Sarma, Int. J. Hydrogen Energy, 2011, 36, 8234–8242 CrossRef CAS.
- R. K. Goud, O. Sarkar and S. Venkata Mohan, Int. J. Hydrogen Energy, 2014, 39(14), 7572–7586 CrossRef.
- O. Sarkar, A. N. Kumar, S. Dahiya, K. V. Krishna, D. K. Yeruva and S. Venkata Mohan, RSC Adv., 2016, 6(22), 18641–18653 RSC.
- J. Hu, J. Zhao, D. Wang, X. Li, D. Zhang, Q. Xu, L. Peng, Q. Yang and G. Zeng, Bioresour. Technol., 2018, 254, 7–15 CrossRef CAS.
- S. Dahiya, S. Lakshminarayanan and S. Venkata Mohan, Chem. Eng. J., 2020, 379, 122135 CrossRef CAS.
- Low carbon power, 2020, https://lowcarbonpower.org/region/India (Accessed on 02 October, 2020).
- S. Venkata Mohan, S. Dahiya, K. Amulya, R. Katakojwala and T. K. Vanitha, Bioresour. Technol. Rep., 2019, 7, 100277 CrossRef.
Footnote |
† Electronic supplementary information (ESI) available. See DOI: 10.1039/d0gc03063e |
|
This journal is © The Royal Society of Chemistry 2021 |
Click here to see how this site uses Cookies. View our privacy policy here.