DOI:
10.1039/D1FO00457C
(Paper)
Food Funct., 2021,
12, 7428-7439
Bioactive extracts from persimmon waste: influence of extraction conditions and ripeness†
Received
12th February 2021
, Accepted 1st June 2021
First published on 1st June 2021
Abstract
In this work, a bioactive persimmon extract was produced from discarded fruits. A central composite design was used to evaluate the effect of different extraction parameters and ripeness stages of persimmon fruits on the total phenolic content and antioxidant activity of the resulting extracts. Significantly greater phenolic contents were obtained from immature persimmon (IP) fruits. The optimum IP extract with the conditions set by the experimental design was industrially up-scaled and its composition and functional properties were evaluated and compared with those obtained under lab-scale conditions. Both extracts contained significant protein (>20%) and phenolic contents (∼11–27 mg GA/g dry extract) and displayed significant antiviral activity against murine norovirus and hepatitis A virus. Moreover, the extract showed no toxicity and significantly reduced the fat content and the cellular ageing of Caenorhabditis elegans (C. elegans) without affecting the worm development. These effects were mediated by down-regulation of fat-7, suggesting an anti-lipogenic activity of this extract.
1. Introduction
According to the Food and Agriculture Organization (FAO), around 14 percent of the food produced worldwide every year is lost from the post-harvest stage to the retail stage and, of this, fruits and vegetables have the second highest wastage rate of the different commodity groups after roots and tubers.1 The reduction of food loss and waste is an important target of the Sustainable Development Goals (SDGs), relating to food security, nutrition and environmental sustainability.
To date, most agro-industrial wastes have been extensively used as a source of fuel, animal feed or organic fertilisers.2 However, there is growing interest in the valorisation of agro-industrial by-products and wastes as abundant, cheap and renewable sources of high added value molecules with specific functional properties.3,4
Persimmon (Diospyros kaki Thunb.) fruits are rich in various nutrients and phytochemicals, including carbohydrates, vitamins, proanthocyanidins, flavonoid oligomers, tannins, phenolic acids, dietary fibre and carotenoids, which significantly contribute to their taste, colour, and nutritional and medicinal value.5 Although China is by far the larger producer, a significant amount of persimmon is also produced in Spain, being the main exporter of the fruit, particularly in Europe.6 Currently, the seasonality and overproduction, together with problems associated with storage, ripening processes, fruit disease and stringent standard demands in terms of fruit appearance, give rise to huge amounts of discarded fruits at different stages of ripeness, which are estimated to be around 5–20% of the fruit harvested.7 In this sense, and given the existing evidence related to the beneficial functional attributes derived from phytochemicals (and more specifically polyphenols) present in the persimmon fruits,6,8 a plausible strategy for their valorisation can be obtaining polyphenol-rich extracts from the discarded fruits. It is also well known that composition changes with fruit ripeness and, thus, exploring these functional attributes in different stages of development can also be helpful to determine the best ripening stage if aiming at this type of valorisation.
Although a few research studies have focused on obtaining purified tannins from persimmon pulp,9,10 from an application view point, obtaining polyphenol-rich extracts with simple, green and scalable processes may be desirable. Therefore, the main goals of this work were first to optimize the extraction protocol to obtain polyphenol-rich extracts from persimmon fruits at two different ripeness stages, selecting the optimal one in terms of polyphenol content and antioxidant capacity. Subsequently, the selected extract was industrially up-scaled to check on the validity of the extraction process and various functional properties of interest for its practical application as a food ingredient were evaluated, including antioxidant, antiviral, anti-obesity and anti-ageing capacity using both in vitro and in vivo (Caenorhabditis elegans) methods. Complete characterization of the selected extract was carried out, including protein content, monosaccharide analysis, polyphenolic profile and a preliminary genotoxicity screening assay through an SOS/umu-test. Overall, the results presented in this work will provide the basis for the production of a potential antioxidant extract produced by valorisation of persimmon fruits.
2. Materials and methods
2.1. Materials
Discarded persimmon (Diospyros kaki Thunb.) “Rojo brillante-Ribera del Xuquer” fruits of an astringent variety in two ripeness stages, immature (I) and mature (M) (see photos in Fig. S1 from the ESI†), were kindly supplied by Anecoop S. Coop. during the autumn season of 2019 (Spain). The unpeeled whole fruits, after removal of the calix and peduncle, were cut into pieces of around 0.5–2.5 cm and they were kept in sealed bags at – 20 °C and subsequently freeze-dried before further processing. Folin–Ciocalteu's reagent, Trolox (97% purity) and gallic acid (≥98.0% purity) were supplied by Sigma-Aldrich (Stenheim, Germany). Ethanol (96% v/v, USP grade) was purchased from Panreac Applichem (Darmstadt, Germany).
2.2. Experimental design for obtaining the persimmon extracts (PE)
A response surface methodology with central composite design (CCD) was carried out on a lab scale to investigate the effect of the solid
:
liquid ratio (mg mL−1) (X1), ethanol
:
water ratio (E/W %) (X2) and temperature (°C) (X3) on the extraction of polyphenolic compounds and antioxidant capacity of the extracts from persimmon fruits, at two different ripeness stages (I and M). The whole design consisted of 20 experimental points carried out in a random order, which included 8 factorial points, 6 centre points and 6 axial points (Table 1). Experimental data were fitted to a second-order polynomial equation. For the extractions, 5 g of freeze-dried samples were used in the various ethanol
:
water solutions, adjusting the volume and ratio of the solvent. Then, they were heated on a hotplate with magnetic stirring for 1 hour. After that, the different persimmon extracts obtained (IPE and MPE for the extracts obtained from the immature and mature fruits, respectively) were filtered with a muslin cloth, freeze-dried and stored in a desiccator with silica gel until subsequent characterization. After freeze-drying, the various extracts obtained were weighed to evaluate the yield.
Table 1 Full central composite design with the experimental data
Run |
Ratio (mg mL−1) |
E/W (%) |
Temp. (°C) |
IPE |
MPE |
TPC (mg GAE/g extract) |
TEAC (μmol TE/g extract) |
TPC (mg GAE/g extract) |
TEAC (μmol TE/g extract) |
1 |
12.50 |
75.0 |
60.0 |
26.68 |
119.36 |
3.44 |
40.18 |
2 |
12.50 |
75.0 |
60.0 |
26.00 |
122.26 |
3.61 |
35.53 |
3 |
12.50 |
100.0 |
60.0 |
2.41 |
44.49 |
1.92 |
35.99 |
4 |
5.00 |
75.0 |
60.0 |
15.33 |
129.37 |
1.78 |
35.27 |
5 |
8.04 |
60.1 |
77.8 |
5.69 |
49.29 |
9.34 |
81.71 |
6 |
20.00 |
75.0 |
60.0 |
20.54 |
167.57 |
3.87 |
40.04 |
7 |
12.50 |
75.0 |
90.0 |
20.79 |
160.64 |
15.19 |
114.72 |
8 |
8.04 |
89.8 |
42.1 |
8.59 |
97.67 |
2.91 |
39.59 |
9 |
16.96 |
60.1 |
42.1 |
23.55 |
119.87 |
4.53 |
35.80 |
10 |
12.50 |
50.0 |
60.0 |
15.66 |
160.50 |
8.59 |
46.44 |
11 |
12.50 |
75.0 |
60.0 |
26.47 |
130.82 |
2.93 |
37.27 |
12 |
8.04 |
89.8 |
77.8 |
17.36 |
134.68 |
3.69 |
49.48 |
13 |
12.50 |
75.0 |
60.0 |
27.14 |
137.96 |
2.98 |
39.41 |
14 |
16.96 |
60.1 |
77.8 |
19.72 |
141.72 |
4.19 |
67.44 |
15 |
12.50 |
75.0 |
60.0 |
28.94 |
162.43 |
4.42 |
37.83 |
16 |
12.50 |
75.0 |
30.0 |
20.19 |
142.19 |
4.39 |
45.83 |
17 |
16.96 |
89.8 |
42.1 |
4.73 |
51.31 |
3.04 |
48.59 |
18 |
16.96 |
89.8 |
77.8 |
11.41 |
116.88 |
3.14 |
62.20 |
19 |
8.04 |
60.1 |
42.1 |
16.08 |
132.26 |
3.74 |
34.00 |
20 |
12.50 |
75.0 |
60.0 |
27.10 |
122.38 |
3.17 |
37.57 |
Tables of the analysis of variance (ANOVA) were generated, and the effect and regression coefficients of individual linear, quadratic, and interactive terms were determined. Quality of fit (coefficient of determination (R2)), adjusted coefficient of determination (adj-R2) and lack of fit were obtained using the software Minitab version 15 (Minitab Inc., Philadelphia, U.S.A.). Differences were considered significant at p ≤ 0.05.
A principal component analysis (PCA) using Minitab 15 software was also performed for a better evaluation of the results.
Then, the optimum extraction conditions in terms of TPC and antioxidant activity were used for up-scaling.
2.3. Total phenolic content (TPC)
The TPC of the extracts was determined using a spectrophotometric method.11 Previously, the freeze-dried IPE and MPE were dissolved in PBS at 5 mg mL−1. The reaction mixture was prepared by mixing 0.2 mL of the extract solutions for each treatment, 1 mL of 10% Folin–Ciocalteu's reagent dissolved in distilled water and 0.8 mL 7.5% NaHCO3. The samples were thereafter incubated in a thermostat at 50 °C for 10 min and the mean absorbance of a triplicate analysis was determined at λmax = 750 nm. The same procedure was repeated for a standard solution of gallic acid and the content of phenolic compounds in the various extracts was expressed in terms of gallic acid equivalents (mg GAE/g extract).
2.4. Antioxidant activity
The Trolox Equivalent Antioxidant Capacity (TEAC) of the persimmon extracts was determined using a modification of the original TEAC method.12 Trolox (6-hydroxy-2,5,7,8-tetramethylchroman-2-carboxylic acid) was used as a standard for antioxidant capacity. Samples were solubilized in distilled water for 12 hours and analyzed for ABTS+˙ 2,2-azino-bis(3-ethylbenzothiazoline-6-sulfonic acid) radical scavenging activity. First, the ABTS+˙ solution with an initial absorbance at 734 nm of 0.70 ± 0.08 was prepared, then 20 μL of the various IPE and MPE were added to 230 μL of the ABTS+˙ solution and the absorbance was registered at 6 min. For calibration, Trolox standards of different concentrations were prepared, and the same procedure was followed. The TEAC of the persimmon extracts was determined by comparing the corresponding percentage of absorbance reduction at 6 min with the Trolox concentration–response curve. All the determinations were carried out, at least, six times using a spectrophotometer (CLARIOstar, BMG LABTECH, Germany) and water as blank.
2.5 Up-scaled extraction of the selected persimmon extract
The extraction process using the optimized conditions in terms of total polyphenolic content and antioxidant activity was industrially scaled up by the Kimitec Group (Vícar, Spain). In order to make the process industrially viable, ethanol was replaced by isopropanol and, instead of freeze drying, the extract was concentrated with a semi-industrial concentrator at mild temperatures. Briefly, 200 kg of fresh immature persimmon samples were washed and ground with an industrial mixer. The extraction was carried out by recirculation in a helical mill at 60 °C for 1 h using a solid
:
liquid ratio of 83.3 g L−1 and a mixture of 75
:
25 isopropanol
:
water solution. The extract was subsequently filtered with a vibrating sieve and concentrated using a semi-industrial concentrator with a capacity of 350 L h−1 and a rotary evaporator. An aliquot of the scaled up extract was freeze-dried to quantify its solid content, determine the yield (50 g concentrated extract /100 g dried sample) and use it for subsequent functional analysis.
2.6. Compositional characterization of the selected persimmon extract
2.6.1. Protein content.
Samples were analysed, in triplicate, for total nitrogen content using an Elemental Analyser Rapid N Exceed (Paralab S.L., Spain). Approximately 100 mg of dry extracts were pressed into pellets and then analyzed using the Dumas method, which is based on the combustion of the sample and subsequent detection of the released N2.13 The protein content was calculated from the nitrogen content multiplied by a factor of 6.25.
2.6.2 Carbohydrate composition.
The carbohydrate composition in both the lab-scale and up-scaled obtained samples was determined after acid methanolysis of the extracts. Briefly, the dried extracts were subjected to methanolysis, with acid methanol (HCl 2 M) for 5 h at 100 °C, followed by neutralization and drying with inert air. Subsequently, the methoxy group was hydrolyzed with trifluoroacetic acid (2 M, 1 h at 100 °C) and the volatile acid was eliminated with air flow. The samples were then re-suspended in bi-distilled water at suitable dilution and analysed by anionic chromatography with a pulsed amperometric detector (HPAEC-PAD) using a Dionex ICS-3000 equipped with a CarbopacPA1 column. Calibration was carried out with commercial standards of the different monosaccharides (fucose, arabinose, rhamnose, galactose, glucose, xylose, mannose, glucuronic acid and galacturonic acid). The experiments were carried out in triplicate.
2.6.3. Polyphenolic profile (UPLC-DAD-MS2 analysis).
The phenolic profile of the lab-scale and up-scaled persimmon extracts was determined in an UPLC-DAD-MS system equipped with a diode array (DAD) and triple quadrupole mass spectrometer (MS) detectors using an electrospray ionization interface (Waters, Milford, MA, USA). The freeze dried lab-scale extract (50 mg) was first suspended in 1 mL 85% methanol, sonicated for 5 min and centrifuged at 12
000 rpm for 10 minutes. The clear supernatant was dissolved (1
:
8.5) in 0.1% (v/v) formic acid, filtered through PTFE (0.2 μm) and injected into the chromatographic system. The concentrated liquid pilot plant persimmon extract (50 mg) was directly dissolved in 0.5 mL 0.1% (v/v) formic acid, filtered and injected into the chromatographic system. Separation was performed in a reversed-phase Acquity UPLC BEH Shield 1.7 μm 1.0 × 150 mm column (Waters, Milford, MA, USA), using a mobile phase composed of A (0.1% v/v formic acid) and B (acetonitrile), under isocratic elution (1% B) from 0 to 5 min and linear gradient (1%–40% B) from 5 to 25 min.
Parent molecular ions (m/z) were obtained by MS scan mode experiments using a cone voltage of 30 V. MS2 fragmentation patterns were obtained using a cone voltage of 30 V and a collision energy of 25 V. Phenolic compounds were quantified using the DAD signal and external calibration curves with pure standards: gallic acid, vanillic acid, hesperetin, quercetin, kaempferol and naringenin (Sigma-Aldrich, Madrid, Spain).
2.7. Functional activity of the selected persimmon extract
2.7.1. Antiviral capacity – virus propagation and cell lines.
Murine norovirus (MNV-1), used as a human norovirus surrogate, was propagated and assayed in RAW 264.7 cells, both kindly provided by Prof. H. W. Virgin (Washington University School of Medicine, USA). Hepatitis A virus (HAV, strain HM-175/18f) was purchased from ATCC (VR-1402) and was propagated and assayed in confluent FRhK-4 cells (kindly provided by Prof. A. Bosch, University of Barcelona, Spain). Semi-purified MNV and HAV viruses were harvested at 2 days and 12 days after infection, respectively, by three freeze–thaw cycles of infected cells followed by centrifugation at 660g for 30 min to remove cell debris. Infectious viruses were enumerated by determining the 50% tissue culture infectious dose (TCID50) with eight wells per dilution and 20 μL of inoculum per well using the Spearman–Karber method.14
Evaluation of antiviral activity of the persimmon extract.
Both the lab-scale and pilot plant scale obtained persimmon extracts were dissolved in PBS (pH 7.2) and mixed with an equal volume of HAV and MNV suspensions (ca. 5 log TCID50/mL) obtaining a final concentration of the extracts of 0.5 and 5 mg mL−1 and incubated overnight at 37 °C. The positive control was virus suspensions in PBS under the same experimental conditions. Each treatment was performed in triplicate. Confluent RAW 264.7 and FRhK-4 monolayers in 96-well plates were used to evaluate the effect of the extracts as described above. The antiviral activity of the persimmon extracts was estimated by comparing the number of infectious viruses on suspensions without the extracts and on the extract-treated virus suspensions. The decay of HAV and MNV titers was calculated as log10
(Nx/N0), where N0 is the infectious virus titer for untreated samples and Nx is the infectious virus titer for persimmon extract-treated samples.
2.7.2. Anti-obesity and anti-ageing capacity of the persimmon extract.
C. elegans culture and persimmon extract supplementation. C. elegans was cultured as previously described.15 Wild type N2 Bristol strain was obtained from the Caenorhabditis Genetics Center (CGC, University of Minnesota, MN) and was grown at 20 °C on Nematode Growth Medium (NGM) with Escherichia coli OP50 as the normal nematode diet. Experiments were performed in 6-well cell culture plates with 4 mL of NGM per well. Supplemented media were prepared as follows: Persimmon extract was dissolved in distilled water and mixed with the NGM at doses of 10, 100, 200 and 1000 μg mL−1. Each concentration was tested in four replicates, including the vehicle (distilled water) as the control. Orlistat supplemented plates (6 μg mL−1 Orlistat; Sigma-Aldrich, St. Louis, MO) were used as the fat reduction control.15 Once the treatments were added to the NGM, the plates were allowed to solidify and dry in a dark environment to protect them from light oxidation. Afterwards, 150 μL of an overnight culture of E. coli OP50 were seeded and the plates were incubated in darkness at room temperature until dry. For all experiments, age-synchronized L4 worms were obtained by standard hypochlorite treatment of gravid animals and eggs were left to hatch overnight in M9 medium. Then, about 1000–2000 L1 larvae were transferred onto plates and were grown for 46 h until they reached the L4 stage, in which worms were collected and experiments were performed.
Nile Red staining.
Nile Red (#N3013, Sigma-Aldrich, St. Louis, MO) staining, a dye for neutral lipids, was performed as previously described with minor modifications.15,16 Briefly, L4 worms were harvested in 1.5 mL tubes and washed twice with PBST (0.01% Triton X-100 in Phosphate Buffered Saline). Then, worms were kept on ice for 15 min to stop pharyngeal activity and fixed in 40% isopropanol for 3 min. The staining was performed by adding 150 μL of Nile Red solution (3 μg mL−1) to fixed worms followed by incubation (30 min) at 20 °C in the dark with gentle rocking. Finally, worms were washed in PBST and mounted on a 2% agarose pad for microscopy visualization.
C. elegans ageing visualization.
Synchronized 500 L1 larvae were transferred onto plates containing DMSO (control) or persimmon extract (200 μg mL−1) and were grown until the L4 stage of adulthood. Worms were collected, washed and mounted onto 2% agarose pads with 1% sodium azide. Lipofuscin pigment was determined by auto-fluorescence as the marker of ageing.17
Image acquisition, quantification and statistical analyses.
For all conditions tested, approximately 300 animals were fixed and stained. Fluorescent images of Nile Red stained worms were captured at 100x magnification on a Nikon SMZ18 research stereomicroscope equipped with an epi-fluorescence system and a DS-FI1C refrigerated colour digital camera (Nikon Instruments Inc., Tokyo, Japan). Images were taken under the same conditions and integration time under a GFP filter (Ex 480–500; DM 505; BA 535–550). The dihydroethidium (DHE)-labelled ROS formation and the lipofuscin auto-fluorescence were detected by measuring the fluorescence intensity using a Nikon Eclipse 80i epi-fluorescence microscope, equipped with a TRITC filter (Ex 540–625; DM 565; BA 605–655) and a DAPI filter (with excitation at 340–380 nm and emission at 435–485 nm), respectively (Nikon Instruments Inc., Tokyo, Japan). In all cases, the image analyses of the Nile Red, DHE and lipofuscin assays were quantified by determining the average pixel intensity per worm (mean value) using ImageJ software as previously described.15 Approximately 25–40 worms were examined in three independent experiments for each condition. C. elegans body-fat reduction (Nile Red) between treated and untreated control conditions (NGM group), together with oxidative stress (DHE), was evaluated by a hierarchical ANOVA test, where replicates were nested in treatments, followed by multiple comparison (Fisher's protected Least Significant Difference, LSD) tests. Lipofuscin determinations between treated (200 μg mL−1) and untreated control worms were statistically compared using Student's t test. All statistical tests were performed using StataSE v12 software (StataCorp LLC, College Station, TX).
Egg laying and worm length.
Egg laying was observed in young adult nematodes (day 3 of growth) grown on NGM agar plates supplemented or not with the persimmon extract (200 μg mL−1). The images were taken at 135 magnifications using a Nikon SMZ18 stereomicroscope equipped with a Nikon DS-Fi1C high-definition colour camera. The worm length (μm) was calculated with the Nikon NIS-ELEMENTS Software (Nikon Instruments Inc., Tokyo, Japan), and determinations between treated (200 μg mL−1) and untreated samples were statistically compared using Student's t test.
RNA extraction and quantitative PCR analysis.
Total RNA from the C. elegans N2 strain was extracted using the Trizol® RNA isolation reagent (Thermo Fisher Scientific, Paisley, UK) according to the manufacturer's instructions. Concentration and purity of RNA were determined at 260/280 nm using a NanoDrop ND-1000 spectrophotometer (Thermo Fisher Scientific, Wilmington, DE, USA). Then, 1000 ng of RNA were treated with DNAse (Ambion™ DNase I, RNase-free; Thermo Fisher Scientific Inc., Waltham, MA, USA) according to the manufacturer's protocol. For the quantitative gene expression analyses, DNA-free RNA was reverse-transcribed into cDNA following the protocol previously described (Navarro-Herrera et al., 2018).15 Gene expression analyses were performed by quantitative-real time PCR (qPCR) using the TaqMan Universal PCR master mix and specific probes (Table S1 in the ESI†) from Applied Biosystems Technologies (Thermo Fisher Scientific Inc., Waltham, MA, USA) and Integrated DNA Technologies Inc., (Coralville, Iowa, USA). All reactions were performed using a CFX384 Touch™ Real-Time PCR Detection System (BioRad, Hercules, CA, USA). The expression level of each gene was normalized compared to the expression of the pmp3 gene from Life Technologies (TaqMan Gene Expression Assays), which was used as the housekeeping gene control. Gene expression differences between treated and untreated worms were quantified using the relative quantification 2−ΔΔCt method.18 Real-time PCR data were statistically analysed using the Wilcoxon test comparing ERE and control data.
2.7.3. Genotoxicity evaluation of the persimmon extract (SOS/umu-test).
The SOS/umu-test was used to determine the DNA-damaging effect and was carried out according to the method of Reifferscheid et al.,19 with some modifications. The test strain S. typhimurium TA1535/pSK1002 (German Collection for microorganisms and Cell cultures (DSMZ)) from stock (−80 °C; in TGA medium containing 10% DMSO as cryoprotective agent) was thawed and 0.5 mL of bacteria were suspended in 100 mL TGA medium supplemented with ampicillin (50 μg mL−1). The tested strain suspension was incubated overnight at 37 °C with slight orbital shaking (155 rpm) until an optical density was reached (OD 600 between 0.5 and 1.5). Thereafter, the overnight culture was diluted with fresh (not supplemented with ampicillin) TGA medium and incubated for 2 h at 37 °C and 155 rpm in order to obtain log-phase bacteria exponential growth culture (OD 600 between 0.15 and 0.4).
The test was performed in the absence and presence of an external metabolic activation system (10% rat S9 mix, prepared from S9 SD rat liver Aroclor KCl frozen, Trinova, Germany) in order to also determine the possible genotoxic effects of any metabolite. In each test performed, negative and positive controls were included, water was used as the solvent control (negative control), and 4-nitroquinoline-N-oxide (4-NQO) (Sigma-Aldrich, China) and 2-aminoanthracene (2-AA) (Sigma-Aldrich, Germany) were used as positive controls in the absence and presence of S9 mix, respectively.
The test procedure was as follows: firstly, the extract was dissolved in water at 80 mg mL−1 (for a final concentration in the assay of 2 mg mL−1) and 11 serial ½ dilutions were prepared in a 96-well plate (plate A; final volume in each well was 10 μL). The highest concentrations in DMSO used for the positive controls were 100 μg mL−1 for 4-NQO (final concentration: 2.5 μg mL−1) and 0.5 mg mL−1 for 2-AA (final concentration: 0.0125 mg mL−1). Then, 70 μL water was added to each well. At this point, each well was checked in order to detect any precipitation of the compounds. In two other 96-well plates (plates B; one for the test with S9 and the other without S9), 10 μL S9 mix or 10 μL PBS, respectively, were added and afterwards, 25 μL of each concentration of previously prepared compound were added. Finally, 90 μL of exponentially growing bacteria were added to each well and both plates were incubated for 4 hours by shaking (500 rpm) at 37 °C. After the incubation period, absorbance at 600 nm was measured in order to evaluate the toxicity on S. typhimurium TA1535/pSK1002.
Genotoxicity is related to survival percentage, which has been calculated as follows:
Survival percentage ε = (A600 for each concentration tested/media A600 for negative control) × 100 |
Afterwards, for the determination of β-galactosidase activity, in two new 96-well plates (plates C), 150 μL ONPG solution (2-nitrophenyl-β-D-galactopyranoside, Sigma-Aldrich, Switzerland) (0.9 mg mL−1 in B-buffer prepared according to Reifferscheid et al.19) was added to each well and 30 μL of the content of each well of plates B was transferred to these plates C. Both plates C were incubated for 30 minutes by shaking (500 rpm) at 28 °C avoiding direct light exposure. After the incubation period, 120 μL stop reagent (Na2CO3, 1 M) was added to stop the reaction. Absorbance at 420 nm was then measured immediately, and β-galactosidase activity (relative units; RU) was calculated as follows:
β-galactosidase enzymatic units = A420 for each concentration tested/A600 for each concentration tested |
And finally, the induction factor (IF) was calculated as follows:
IF = β-galactosidase RU for each concentration tested/average β-galactosidase RU for the negative control. |
Where: average β-galactosidase RU for the negative control = average
A420 for the negative control/average
A600 for the negative control.
In the same way, β-galactosidase relative units were calculated for both positive controls and the test was only considered valid if the positive controls reached an IF ≥2 under the given test conditions.
An extract is considered genotoxic when under any of the conditions studied (with or without metabolic activation) the induction factor is ≥2 at non-toxic concentrations (bacteria survival percentage ≥80%). Any well where compound precipitation was observed was discarded from analysis.
3. Results and discussion
A central composite design based on the response surface methodology was used to determine the optimal parameters giving rise to persimmon (Diospyros kaki Thunb.) extracts with the greatest polyphenol content and antioxidant capacity (cf.Table 1), aiming to provide relevant information for further industrial up-scaling and evaluation of the bioactive properties. The extracts were obtained from persimmon fruits in two different ripeness stages (IPE and MPE) and the effects of the solid
:
liquid ratio (g mL−1), the ethanol/water solvent ratio E/W (%) and the extraction temperature (°C) on the total polyphenolic content (TPC) as well as on the antioxidant capacity of the extracts were evaluated.
The overall extraction yields for the different extraction conditions and raw materials (both immature and mature persimmons) ranged between 50 g/100 g dry weight and 75 g/100 g dry weight, indicating that the range of parameters selected for extraction was suitable, as excellent yields were obtained in all cases. The high yield obtained in this case can be attributed to the extractions of other components, such as free sugars (glucose and fructose) which constitute around 66–83% dry weight of the whole fruit (data not shown).
3.1. Selection of extraction conditions
All the results were statistically analyzed through an analysis of variance (ANOVA) and the results are compiled in Table S2 from the ESI.† The regression models were highly significant with satisfactory coefficients of determination for total phenolic content (TPC) (R2 = 0.93–0.96). It has to be highlighted that the method used for total phenolic content quantification can also reflect the presence of other reducing compounds like ascorbic acid present in the fruits and, thus, should only be considered as a rough estimation. The values of the adjusted determination coefficients (adj R2 = 0.87–0.92) also validated that the models were highly significant. Moreover, the lack-of-fit test, which measures the competence of the model, did not result in a significant P-value, highlighting that the models were sufficiently precise for predicting the phenolic content of the extracts. The ABTS response also exhibited a high model accuracy for the mature fruits, showing R2 of 94.61% for MPE, although it was lower for IPE (R2 68.92%), indicating a lower reliability of the developed model. The response surface plots were also generated (cf.Fig. 1) to better understand the effect of extraction conditions on both TPC and antioxidant potential.
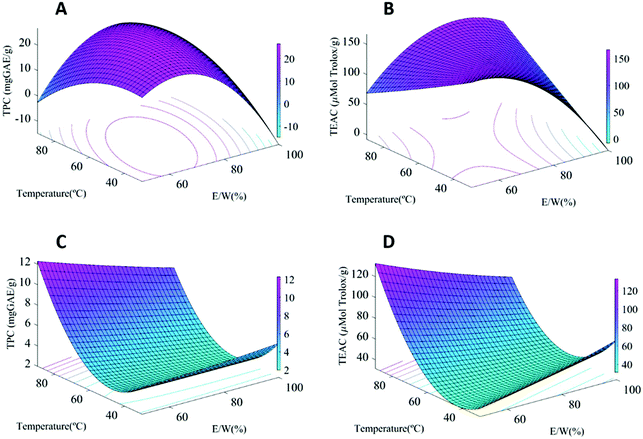 |
| Fig. 1 Response surfaces of the TPC and antioxidant activity of extracts from (a and b) IPE and (c and d) MPE as a function of temperature and E/W with a fixed solid : liquid ratio (12.5 mg mL−1). | |
Both the total phenolic content (TPC) and antioxidant capacity (using the ABTS assay) of the samples were taken into account to select the optimum extraction conditions for subsequent up-scale. The first remarkable result was that the TPC and antioxidant capacity showed a clear dependence on the fruit stage. As expected, the immature fruit samples showed significantly higher TPC (28.9 mg GAE/g) and antioxidant capacity values (167.6 μmol Trolox/g extract) than the mature ones (15.2 mg GAE/g and 114.7 μmol Trolox/g extract, respectively). This is explained by the ripeness stage, as less mature fruits have been reported to contain a greater amount of soluble tannins prone to complex formation, which are usually degraded or transformed into insoluble ones during ripening,5 having a positive impact on the astringency.20,21
Interestingly, remarkable differences were observed in the factors affecting the responses depending on the starting material. While for IPE, the E/W ratio had the highest effect on both response parameters, for MPE, the TPC and antioxidant capacity were mainly influenced by the extraction temperature (greater temperatures fostering phenolic extraction) (cf. Table S2†). This is clearly patent when looking at the response-surface plots for the TPC values (Fig. 1). For IPE, a combination of intermediate E/W ratios and high temperature is necessary to maximize polyphenol and antioxidant performance (Fig. 1a and b), while only high temperatures are most significantly affecting these for MPE (Fig. 1c and d). This is probably related to the different nature of the polyphenolic compounds present in the persimmon fruits depending on the ripeness stage. Whereas the intermediate E/W ratio favours the extraction of soluble tannins from the immature fruits, these temperatures were insufficient in the case of the mature persimmons, since higher temperatures are required in these cases to solubilize the complexes formed between insoluble tannins and other compounds, such as pectin, which have been associated with the ripening process.20 The TPC values obtained in this work were similar to the values reported for dried persimmon peel extract (2.30 to 18.31 mg GAE/g),22 and higher than that of other fruit extracts (3.09–9.44 mg GAE/g elderberry, blueberry 5.58 mg GAE/g, and blackberry 2.72 mg GAE/g DW),23,24 confirming the potential use of persimmon fruits as an important source of phenolic compounds for bioactive applications. A PCA was also carried out to evaluate the variance related to the extraction conditions and the results are included in the ESI (Fig. S2†).
In view of the results, immature fruits showed the highest antioxidant and TPC values and thus, this stage was selected as the raw material for the up-scaled process. The extraction conditions were set at 60 °C and an E/W ratio of 75%. The extract was up-scaled by the Kimitec Group, but in order to make the process more sustainable from an economical view point, ethanol was substituted with isopropanol. Both the optimized IPEs obtained at lab and industrial scales were characterized in terms of composition and functional properties.
3.2. Compositional characterization of the “Immature Persimmon Extract” (IPE)
3.2.1. Protein content.
The protein content of the lab-scale and up-scaled IP was 4.48 ± 0.13 g per 100 g dry weight and 1.35 ± 0.11 g per 100 g dry weight, respectively. The contents are higher than those reported in the literature for mature persimmon (Diospyros kaki L.) (1.14–1.56 g per 100 g dry weight),25 indicating that most of the proteins present in the fruits are enriched in the IPE under the applied extraction conditions. Ripeness, variety and nitrogen cultivation vastly affect the protein content of the fruits, as observed in a couple of studies in which higher protein contents were generally observed in earlier stages of maturation.26–28
3.2.2. Carbohydrate composition.
The carbohydrate composition of the persimmon extracts mainly consisted of glucose, arising from free glucose mainly, as well as from xyloglucan hemicelluloses, pectin components, such as galacturonic acid, galactose, rhamnose and arabinose, and xylose from xyloglucan (Table S3 in the ESI†), in agreement with the major soluble carbohydrate components found in persimmon.29 Although similar total carbohydrate contents were found in both lab- and up-scaled extracts (45–50%), glucose was much enriched in the up-scaled extract (45%) in comparison with the lab-scale IPE (20%), where significant quantities of xyloglucan and pectin were to be found. These differences may be ascribed to the different solvents used, as ethanol is more polar and thus able to extract low molecular weight pectin and xyloglucan components.
3.2.3. Polyphenolic profile.
To gain further insights into the composition of the selected extract obtained both at lab and industrial scales, their polyphenolic profile was also characterized and the results are compiled in Table 2. Details about the quantification and the obtained chromatograms can be found in the ESI (Table S4 and Fig. S3–S7†). Several phenolic compounds were identified in the persimmon extracts on the basis of their DAD, MS and MS2 information and comparison with pure standards and/or bibliographic data.30–32 The main phenolic compound in both extracts was the hydrolysable tannin gallic acid, which was more abundant in the up-scaled IPE than in the one produced under laboratory conditions. Other phenolic compounds such as naringin, hesperidin or naringenin were only present in the industrially up-scaled extract (see Table S4 in the ESI†). Again, the solvent combination used for extraction seems to play an important role in the solvation and extraction of specific phenolic molecules, a fact which also has an impact on the antioxidant properties of the extract, as commented on later and also observed in previous studies.33
Table 2 Content of the different phenolic compounds tentatively identified (mg kg−1) in the lab scale and up-scaled immature persimmon extracts (IPE) (mean ± standard deviation, n = 3). Nd: not detected
Peak |
Tentative identification |
Labscale IPE |
Up-scaled IPE |
1 |
Gallic acid |
728.5 ± 122.9 |
998.1 ± 37.2 |
2 |
Galloyl hexoside |
nd |
64.8 ± 1.3 |
3 |
Vanillic acid hexoside |
15.9 ± 1.6 |
28.2 ± 1.2 |
4 |
Naringin |
nd |
73.2 ± 3.0 |
5 |
Hesperidin |
nd |
277.8 ± 86.8 |
6 |
Quercetin hexoside I |
22.3 ± 3.3 |
13.4 ± 0.5 |
7 |
Quercetin hexoside II |
46.1 ± 8.9 |
28.3 ± 2.0 |
8 |
Kaempferol hexoside I |
19.0 ± 0.4 |
18.4 ± 2.2 |
9 |
Kaempferol hexoside II |
29.4 ± 0.6 |
16.7 ± 2.7 |
10 |
Kaempferol hexoside gallate I |
nd |
1.5 ± 0.3 |
11 |
Kaempferol hexoside gallate II |
nd |
3.4 ± 0.6 |
12 |
Naringenin |
nd |
27.0 ± 15.5 |
3.3. Functional activity of the “Immature Persimmon Extract” (IPE)
3.3.1. Antiviral capacity.
The antiviral activity of the lab-scale and up-scaled IPE was evaluated on HAV and MNV (a human surrogate), and the results are compiled in Table 3. Both extracts greatly decreased the titer of MNV detection limits at the greatest concentration tested (5 mg mL−1), although only the up-scaled extract produced a significant reduction at lower concentrations (0.5 mg mL−1). The up-scaled extract also showed a marked antiviral effect on HAV, with a titer reduction of 2.83 log. The antiviral capacity of persimmon extracts has been previously reported and has been correlated with the persimmon tannins present.8 The overall higher antiviral effectiveness of the up-scaled extract could thus be related to a higher polyphenol content. In fact, it was found that tannin concentrations greater than 0.11 mg mL−1 were needed to reduce the infectivity of a human norovirus surrogate by more than 2.5 log,8 which explains why only the greatest extract concentration tested was seen to be effective in the reduction of the viral load.
Table 3 Effect of the persimmon extracts on the infectivity of murine norovirus (MNV) and hepatitis A virus (HAV) after overnight incubation at 37 °C
Sample |
Concentration (mg ml−1) |
MNV |
HAV |
Log TCID50/mL |
R |
Log TCID50/mL |
R |
R: Reduction; UDL*: Under the detection limits (1.15). Within each column for each concentration, different letters denote significant differences between treatments (P < 0.05). |
Lab-scale IPE |
0 |
5.41 ± 0.29a |
|
5.78 ± 0.19a |
|
0.5 |
4.16 ± 0.19c |
1.25 |
5.37 ± 0.1a |
0.42 |
5 |
UDL*b |
>4.26 |
6.07 ± 0.37a |
−0.29 |
Up-scaled IPE |
0 |
4.03 ± 0.29d |
|
5.32 ± 0.22b |
|
0.5 |
5.53 ± 0.19d |
−0.50 |
5.16 ± 0.19b |
0.17 |
5 |
UDL*e |
>2.88 |
2.49 ± 0.07c |
2.83 |
3.3.2 Anti-obesity and anti-ageing capacity.
As has been previously described (see Table 2), the IPEs obtained contain a relevant content of polyphenols, including naringin, hesperidin and derivatives of quercetin and kaempferol, together with different phenolic acids, such as gallic and vanillic acids. All these phenolic compounds are considered bioactive compounds with reported antioxidant and anti-ageing activities.34,35 Therefore, the in vivo antioxidant activity was evaluated using the model C. elegans, and, its potential activity over other parameters associated with nematode health, such as development, ageing or energy homeostasis regulation, was also evaluated.
C. elegans has been demonstrated to be a powerful in vivo model for the screening of food ingredients and bioactive compounds with health-related benefits, such as antioxidant and anti-ageing activities.36,37 Besides, due to the highly conserved regulatory pathways of energy homeostasis between mammals and C. elegans, this nematode is considered a popular model for exploring the ability of different functional ingredients modulating fat storage, commonly determined by quantification of the triglyceride accumulation with lipid-specific stains such as Oil Red O or Nile Red.16 Thus, this model has been widely used to identify bioactive compounds with potential application in the prevention of obesity-related disorders.15,37
To determine the in vivo antioxidant capacity, synchronized L1 worms were grown in the presence of different concentrations of the IRE extract (100 and 200 μg mL−1) until the L4 stage, when nematodes were collected and stained with dihydroethidium (DHE) staining,38 to calculate the levels of intercellular reactive oxygen species (ROS). Surprisingly, despite the high content of antioxidant compounds, no differences were observed in the ROS levels of the IRE extract-treated and untreated worms.
However, it was observed that all tested doses (10, 100, 200 and 1000 μg mL−1) of the IRE extract were able to reduce the lipid accumulation of C. elegans, in comparison with untreated-control worms (Fig. 2A), as it was determined by a reduction in the Nile Red staining (Fig. 2B). As a result, the up-scaled IPE was able to modulate the C. elegans lipid homeostasis when treated from L1 to L4 stages.
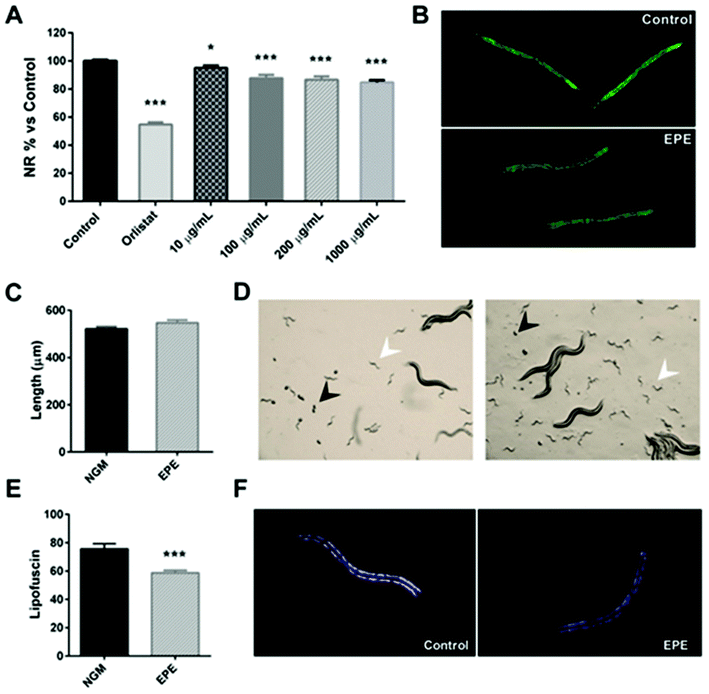 |
| Fig. 2 (A) C. elegans lipid quantification data (relative to NGM) obtained after treatment with different doses (10, 100, 200 and 1000 μg mL−1) of the persimmon enriched-polyphenolic extract (EPE) and measured by Nile Red staining. Orlistat (6 μg mL−1)-supplemented wells were used as the positive control of fat loss. All data correspond to the mean ± SEM. Significance refers to the effect of persimmon extract with respect to control worms (ANOVA followed by the LSD test, * p < 0.05; *** p < 0.001). (B) Nile Red staining of the control and EPE extract (200 μg mL−1)-treated worms. (C) Size (μm) of the treated- and un-treated worms on day 1 of adulthood (mean ± SEM). (D) Microscope observation of the presence of eggs (black arrows) and L1 larvae (white arrows) in both control (left) and EPE-supplemented (right) plates. (E) Quantification of lipofuscin aging-pigment of EPE-treated worms compared to the control (mean ± SEM). *** Shows the level of statistical significance of the differences (T test, p < 0.001). (F) Visualization of C. elegans lipofuscin pigment auto-fluorescence by microscopy of control and EPE-treated worms. | |
In order to determine if the reduction in the lipid content was related to an effect on the worm development, a qualitative analysis was performed by microscopy on day 1 of adulthood (day 3 of treatment). In this experiment (Fig. 2C), it was observed that both control and IPE-treated plates exhibited the presence of eggs (black arrows) and L1 larvae (white arrows), with no differences in the time of appearance of these eggs. The analysis of the worm size of the adults revealed no differences in length between control and treated-worms (Fig. 2D), indicating that the lipid-reducing effect of the persimmon extract was not related to differences in the worm development. Moreover, it was observed that persimmon extract-treated worms (200 μg mL−1) exhibited a significant reduction of the intestinal lipofuscin pigment, an auto-fluorescent molecule commonly used as a marker of the rate of ageing39 (Fig. 2E and F), in comparison with the untreated-control worms. Therefore, this result evidenced that the treatment with IPE during L1 to adulthood reduced the C. elegans fat content without having an effect on the worm development, and this activity was accompanied by a reduction in the cellular ageing of the nematode.
Finally, the plausible mechanisms underlying the lipid- and ageing-reducing activities of the persimmon extract were determined by evaluating the expression of genes involved in different processes related to lipid synthesis (fat-7 and fat-5), lipid oxidation (acox-1 and maoc-1), and oxidative stress (skn-1). As can be observed in Fig. 3, treatment with IPE induced a significant downregulation (Wilcoxon test p = 0.047) of fat-7. This gene encodes a Δ9 desaturase that catalyses the insertion of the first double bond into a saturated fatty acid (stearic acid, C18:0) at the C9 position, constituting a key enzyme in the synthesis of fatty acids.43 Inhibition of this gene is associated with a reduced total triacylglyceride content,40 so the down-regulation by the persimmon extract might indicate a reduction in the fat synthesis rate. No changes were observed in the expression of fat-5, encoding for a palmitic acid-specific desaturase,40 or in that of the maoc-1 and acox-1 genes, involved in the fatty acid β-oxidation. These results suggest that the fat-reducing activity of the persimmon enriched-polyphenolic extract might be attributed to a reduced lipogenesis rate through down-regulation of fat-7, not to increased lipolysis and subsequent β-oxidation. Finally, no changes were observed in the expression of skn-1, an ortholog of human Nrf/CNC proteins which are activated under oxidative stress conditions41 which might contribute to explaining the result obtained in the DHE staining. However, it should be mentioned that the experiments were performed without applying any type of chemical or thermal stress to the nematode, so further studies are needed to investigate the antioxidant activity of this persimmon extract in C. elegans.
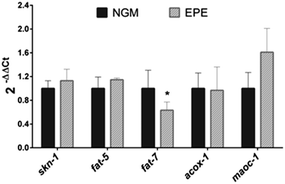 |
| Fig. 3 qPCR analysis performed in EPE-treated and untreated control worms. Results are expressed as the fold-difference expression levels of each gene in the EPE-treated group compared to the untreated-control group, calculated with the 2−ΔΔCt method. Significance refers to the effect of EPE with respect to control worms (student t test, * p < 0.05). | |
3.3.3. Genotoxicity screening assay.
Finally, the genotoxicity of the up-scaled IPE was also determined in order to evaluate its applicability in food related products. All the controls used for the SOS/umu-test were correct (IF < 2 for negative and IF > 2 for positive controls). The wells corresponding to the higher concentrations tested (final concentrations: 2, 1 and 0.5 mg mL−1) showed precipitation in the wells and were discarded from analysis. The well corresponding to a final concentration of 0.25 mg mL−1 showed some turbidity. The extract was considered non-genotoxic as the induction factor was always lower than 2 at non-toxic concentrations with or without metabolic activation (see Fig. S8 in the ESI†).
Even if a high degree of agreement between the SOS/umu-test and the standardized Ames test (OECD guideline 471) for mutagenicity testing has been found,42 it should be noted that the SOS/umu-test is used for screening purposes. For regulatory purposes, the negative results obtained with the SOS/umu-test should be further evaluated with the standardized Ames test.
5. Conclusions
In this work, optimization of the extraction of a polyphenol-rich extract from persimmon fruits was carried out using a central composite design. The ripeness degree of the fruits was seen to affect, not only the extract composition (greater in phenolic compounds in those obtained from immature fruits), but also the determining factors for extraction. Specifically, in the mature fruits, increased phenolic content and antioxidant capacity were seen upon a temperature increase, while in the case of the immature persimmons, the solvent composition was the main relevant factor in determining polyphenol extraction. The best extract in terms of phenolic content and antioxidant capacity (from immature persimmon fruits – IPE) was industrially up-scaled and compositionally characterized, being very rich in gallic acid and other phenolic compounds previously described to have diverse bioactive properties. Specifically, in this work, the IPE demonstrated a good antiviral capacity, with a significant reduction in the titers of hepatitis A virus and murine norovirus. Moreover, treatment of C. elegans with IPE during L1 to adulthood was able to reduce the fat content and the cellular ageing of the nematode without affecting the worm development. These effects were mediated by down-regulation of fat-7, suggesting an anti-lipogenic activity of this extract. Although further studies are needed to investigate the antioxidant activity of the extract in C. elegans, as well as to confirm the anti-obesity and anti-ageing activities of persimmon extract in superior mammalian models, these preliminary data would suggest the potential application of this persimmon extract in the prevention of obesity and ageing-related diseases.
Author contributions
Daniel Alexander Mendez: Methodology, investigation, formal analysis, and writing – original draft. María José Fabra: Conceptualization, formal analysis, and writing – review and editing. Irene Falco: Methodology and investigation. Gloria Sánchez: Writing – review and editing. Paula Aranaz: Methodology, formal analysis and writing. Ariane Vettorazzi: Methodology, formal analysis, and writing. Albert Ribas-Agustí: Methodology, investigation, and writing – review and editing. Carlos Javier González-Navarro: Methodology, formal analysis, and writing – review and editing. Massimo Castellari: Methodology and writing – review and editing. A. Martinez-Abad: Supervision, and writing – review and editing. Amparo López-Rubio: Conceptualization, methodology, funding acquisition, project administration, and writing – review and editing.
Conflicts of interest
There are no conflicts to declare.
Acknowledgements
This work was financially supported by the grant RTI-2018-094268-B-C22 (MCI/AEI/FEDER, EU). Mendez D. A. is supported by the Ministry of Science, Technology and Innovation (Minciencias) of the Colombian Government (783-2017). M. J. Fabra and A. Martinez-Abad are recipients of Ramon y Cajal (RYC-2014-158) and Juan de la Cierva (IJDC-2017-31255), respectively, from the Spanish Ministry of Economy, Industry and Competitiveness. We also appreciate the funding of the CERCA program (Centres de Recerca de Catalunya) of the Generalitat de Catalunya and from the CIEN project “BIOPRO” from CDTI. Nune Sahakyan is kindly acknowledged for experimental support.
References
- FAO, The State of Food and Agriculture 2019, Moving forward on food loss and waste reduction, Food and Agriculture Organization of the United Nations, Rome, 2019 Search PubMed.
- S. Venkata Mohan, G. N. Nikhil, P. Chiranjeevi, C. Nagendranatha Reddy, M. V. Rohit, A. N. Kumar and O. Sarkar, Waste biorefinery models towards sustainable circular bioeconomy: Critical review and future perspectives, Bioresour. Technol., 2016, 215, 2–12 CrossRef CAS PubMed.
- J. Müller-Maatsch, M. Bencivenni, A. Caligiani, T. Tedeschi, G. Bruggeman, M. Bosch, J. Petrusan, B. Van Droogenbroeck, K. Elst and S. Sforza, Pectin content and composition from different food waste streams, Food Chem., 2016, 201, 37–45 CrossRef PubMed.
- D. A. Méndez, M. J. Fabra, L. Gómez-Mascaraque, A. López-Rubio and A. Martinez-Abad, Modelling the Extraction of Pectin towards the Valorisation of Watermelon Rind Waste, Foods, 2021, 10, 738 CrossRef PubMed.
- S. K. Yoo, J. M. Kim, S. K. Park, J. Y. Kang, H. J. Han, H. W. Park, C. W. Kim, U. Lee and H. J. Heo, Chemical compositions of different cultivars of astringent persimmon (Diospyros kaki Thunb.) and the effects of maturity, Korean J. Food Sci. Technol., 2019, 51, 248–257 Search PubMed.
- L. Domínguez Díaz, E. Dorta, S. Maher, P. Morales, V. Fernández-Ruiz, M. Cámara and M.-C. Sánchez-Mata, Potential Nutrition and Health Claims in Deastringed Persimmon Fruits (Diospyros kaki L.), Variety ‘Rojo Brillante’, PDO ‘Ribera del Xúquer’, Nutrients, 2020, 12, 1397 CrossRef PubMed.
- S. Munera, N. Aleixos, C. Besada, J. Gómez-Sanchis, A. Salvador, S. Cubero, P. Talens and J. Blasco, Discrimination of astringent and deastringed hard ‘Rojo Brillante’ persimmon fruit using a sensory threshold by means of hyperspectral imaging, J. Food Eng., 2019, 263, 173–180 CrossRef CAS.
- M. Kamimoto, Y. Nakai, T. Tsuji, T. Shimamoto and T. Shimamoto, Antiviral Effects of Persimmon Extract on Human Norovirus and Its Surrogate, Bacteriophage MS2, J. Food Sci., 2014, 79, M941–M946 CrossRef CAS PubMed.
- H.-F. Gu, C.-M. Li, Y. Xu, W. Hu, M. Chen and Q. Wan, Structural features and antioxidant activity of tannin from persimmon pulp, Food Res. Int., 2008, 41, 208–217 CrossRef CAS.
- M. Liu, J. Wang, K. Yang, Y. Qi, J. Zhang, M. Fan and X. Wei, Optimization of ultrasonic-assisted extraction of antioxidant tannin from young astringent persimmon (Diospyros kaki L.) using response surface methodology, J. Food Process. Preserv., 2018, 42, e13657 CrossRef.
- V. L. Singleton, R. Orthofer and R. M. Lamuela-Raventós, Analysis of total phenols and other oxidation substrates and antioxidants by means of folin-ciocalteu reagent, Methods Enzymol., 1999, 299, 152–178 CAS.
- R. Re, N. Pellegrini, A. Proteggente, A. Pannala, M. Yang and C. Rice-Evans, Antioxidant activity applying an improved ABTS radical cation decolorization assay, Free Radicals Biol. Med., 1999, 26, 1231–1237 CrossRef CAS PubMed.
- P. G. Wiles, I. K. Gray, R. C. Kissling, C. Delahanty, J. Evers, K. Greenwood, K. Grimshaw, M. Hibbert, K. Kelly, H. Luckin, K. McGregor, A. Morris, M. Petersen, F. Ross and M. Valli, Routine Analysis of Proteins by Kjeldahl and Dumas Methods: Review and Interlaboratory Study Using Dairy Products, J. AOAC Int., 1998, 81, 620–632 CrossRef CAS PubMed.
- R. M. Pintó, J. M. Diez and A. Bosch, Use of the colonic carcinoma cell line CaCo-2 for in vivo amplification and detection of enteric viruses, J. Med. Virol., 1994, 44, 310–315 CrossRef PubMed.
- D. Navarro-Herrera, P. Aranaz, L. Eder-Azanza, M. Zabala, C. Hurtado, A. Romo-Hualde, J. A. Martínez, C. J. González-Navarro and J. L. Vizmanos, Dihomo-gamma-linolenic acid induces fat loss in: C. Elegans in an omega-3-independent manner by promoting peroxisomal fatty acid β-oxidation, Food and Funct., 2018, 9, 1621–1637 RSC.
- E. C. Pino, C. M. Webster, C. E. Carr and A. A. Soukas, Biochemical and high throughput microscopic assessment of fat mass in Caenorhabditis elegans, J. Visualized Exp., 2013, 73 DOI:10.3791/50180.
- M. P. Gardner, D. Gems and M. E. Viney, Aging in a very short-lived nematode, Exp. Gerontol., 2004, 39, 1267–1276 CrossRef PubMed.
- K. J. Livak and T. D. Schmittgen, Analysis of relative gene expression data using real-time quantitative PCR and the 2-ΔΔCT method, Methods, 2001, 25, 402–408 CrossRef CAS PubMed.
- G. Reifferscheid, J. Heil, Y. Oda and R. K. Zahn, A microplate version of the SOS/umu-test for rapid detection of genotoxins and genotoxic potentials of environmental samples, Mutat. Res., 1991, 253, 215–222 CAS.
- S. Taira, M. Ono and N. Matsumoto, Reduction of persimmon astringency by complex formation between pectin and tannins, Postharvest Biol. Technol., 1997, 12, 265–271 CrossRef CAS.
- T. Mamet, Z.-Z. Ge, Y. Zhang and C.-M. Li, Interactions between highly galloylated persimmon tannins and pectins, Int. J. Biol. Macromol., 2018, 106, 410–417 CrossRef CAS PubMed.
- I.-W. W. Hwang, M. C. Jeong and S. K. Chung, The Physicochemical Properties and the Antioxidant Activities of Persimmon Peel Powders with Different Particle Sizes, J. Korean Soc. Appl. Biol. Chem., 2011, 54, 442–446 CrossRef CAS.
- W. Huang, H. Zhang, W. Liu and C. Li, Survey of antioxidant capacity and phenolic composition of blueberry, blackberry, and strawberry in Nanjing, J. Zhejiang Univ., Sci., B, 2012, 13, 94–102 CrossRef CAS PubMed.
- R. Domínguez, L. Zhang, G. Rocchetti, L. Lucini, M. Pateiro, P. E. S. Munekata and J. M. Lorenzo, Elderberry (Sambucus nigra L.) as potential source of antioxidants. Characterization, optimization of extraction parameters and bioactive properties, Food Chem., 2020, 330, 127266 CrossRef PubMed.
- S. Karaman, O. S. Toker, M. Çam, M. Hayta, M. Doǧan and A. Kayacier, Bioactive and Physicochemical Properties of Persimmon as Affected by Drying Methods, Drying Technol., 2014, 32, 258–267 CrossRef CAS.
- S.-T. Choi, D.-S. Park and K.-P. Hong, Status of nitrogenous and carbohydrate compounds as affected by nitrogen fertigation rates in young persimmon trees, Sci. Hortic., 2011, 130, 354–356 CrossRef CAS.
- S. T. Choi, D. S. Park, S. M. Kang and S. K. Kang, Influence of leaf-fruit ratio and nitrogen rate on fruit characteristics, nitrogenous compounds, and nonstructural carbohydrates in young persimmon trees, HortScience, 2012, 47, 410–413 CAS.
- Y. W. Yoon, S. T. Choi, D. S. Park, C. W. Rho, D. H. Kim and S. M. Kang, Changes in organic and inorganic nutrients in terminal shoots of ‘Fuyu’ persimmon during spring growth, Korean J. Hortic. Sci. Technol., 2014, 32, 279–288 CrossRef CAS.
- A. Cutillas-Iturralde, M. J. Peña, I. Zarra and E. P. Lorences, A xyloglucan from persimmon fruit cell walls, Phytochemistry, 1998, 48, 607–610 CrossRef CAS.
- C. Jiménez-Sánchez, J. Lozano-Sánchez, N. Marti, D. Saura, M. Valero, A. Segura-Carretero and A. Fernández-Gutiérrez, Characterization of polyphenols, sugars, and other polar compounds in persimmon juices produced under different technologies and their assessment in terms of compositional variations, Food Chem., 2015, 182, 282–291 CrossRef PubMed.
- R. Martínez-Las Heras, P. Quifer-Rada, A. Andrés and R. Lamuela-Raventós, Polyphenolic profile of persimmon leaves by high resolution
mass spectrometry (LC-ESI-LTQ-Orbitrap-MS), J. Funct. Foods, 2016, 23, 370–377 CrossRef.
- E. Sentandreu, M. Cerdán-Calero, J. M. Halket and J. L. Navarro, Rapid screening of low-molecular-weight phenols from persimmon (Diospyros kaki) pulp using liquid chromatography/UV-visible/electrospray mass spectrometry analysis, J. Sci. Food Agric., 2015, 95, 1648–1654 CrossRef CAS PubMed.
- T. Venkatesan, Y. W. Choi and Y. K. Kim, Impact of Different Extraction Solvents on Phenolic Content and Antioxidant Potential of Pinus densiflora Bark Extract, BioMed Res. Int., 2019, 2019, 1–14 CrossRef PubMed.
- Y. Kawai, Understanding metabolic conversions and molecular actions of flavonoids in vivo: Toward new strategies for effective utilization of natural polyphenols in human health, Int. J. Med. Invest., 2018, 65, 162–165 CrossRef PubMed.
- C. Peng, X. Wang, J. Chen, R. Jiao, L. Wang, Y. M. Li, Y. Zuo, Y. Liu, L. Lei, K. Y. Ma, Y. Huang and Z. Y. Chen, Biology of ageing and role of dietary antioxidants, BioMed Res. Int., 2014, 2014 Search PubMed.
- N. Saul, K. Pietsch, S. R. Stürzenbaum, R. Menzel and C. E. W. Steinberg, Diversity of Polyphenol Action in Caenorhabditis elegans : Between Toxicity and Longevity, J. Nat. Prod., 2011, 74, 1713–1720 CrossRef CAS PubMed.
- P. Shen, Y. Yue and Y. Park, A living model for obesity and aging research: Caenorhabditis elegans, Crit. Rev. Food Sci. Nutr., 2018, 58, 741–754 CrossRef PubMed.
- Y. Wei and C. Kenyon, Roles for ROS and hydrogen sulfide in the longevity response to germline loss in Caenorhabditis elegans, Proc. Natl. Acad. Sci. U. S. A., 2016, 113, E2832–E2841 CrossRef CAS PubMed.
- V. H. C. Liao, Use of Caenorhabditis elegans to Study the Potential Bioactivity of Natural Compounds, J. Agric. Food Chem., 2018, 66, 1737–1742 CrossRef CAS PubMed.
- K. Ashrafi, Obesity and the regulation of fat metabolism, WormBook, 2007, 1–20 Search PubMed.
- J. H. An, K. Vranas, M. Lucke, H. Inoue, N. Hisamoto, K. Matsumoto and T. K. Blackwell, Regulation of the Caenorhabditis elegans oxidative stress defense protein SKN-1 by glycogen synthase kinase-3, Proc. Natl. Acad. Sci. U. S. A., 2005, 102, 16275–16280 CrossRef CAS PubMed.
- G. Reifferscheid and J. Heil, Validation of the SOS/umu test using test results of 486 chemicals and comparison with the Ames test and carcinogenicity data, Mutat. Res., Genet. Toxicol., 1996, 369, 129–145 CrossRef CAS.
- J. L. Watts, Fat synthesis and adiposity regulation in Caenorhabditis elegans, Trends Endocrinol Metab., 2009, 20(2), 58–65 CrossRef CAS PubMed.
Footnote |
† Electronic supplementary information (ESI) available. See DOI: 10.1039/d1fo00457c |
|
This journal is © The Royal Society of Chemistry 2021 |