DOI:
10.1039/D1FO00210D
(Paper)
Food Funct., 2021,
12, 5478-5487
Hydroxytyrosol alleviates oxidative stress and neuroinflammation and enhances hippocampal neurotrophic signaling to improve stress-induced depressive behaviors in mice
Received
21st January 2021
, Accepted 27th April 2021
First published on 28th April 2021
Abstract
Hydroxytyrosol (HT), the main phenolic compound in olives and olive products, has antioxidative, anti-inflammatory, neuroprotective, and other physiological functions. The effects of HT on depression are unclear. The aim of this study was to explore the effects of HT on chronic unpredictable mild stress (CUMS) induced depressive-like behaviors. Mice were exposed to CUMS for 9 weeks and then treated with HT beginning in the second week and continuing for 7 weeks. Behavioral, biochemical, and molecular tests were conducted at the end of the experiment. The sucrose preference was significantly decreased in the CUMS group versus the healthy control group. Also, immobility times in forced swimming and tail suspension tests were increased in CUMS-induced mice, but treatment with HT significantly reversed this change. HT ameliorated oxidative stress in CUMS-exposed mice by enhancing superoxide dismutase activity and reducing reactive oxygen species and malondialdehyde levels in the hippocampus. HT administration significantly suppressed microglia activation and inhibited the expression of tumor necrosis factor alpha and interleukin 1 beta in the hippocampus versus the untreated group. The expression level of glial fibrillary acidic protein (GFAP) and the number of GFAP-immunoreactive astrocytes in the hippocampus were significantly augmented by HT. Furthermore, HT treatment increased the expression of hippocampal brain-derived neurotrophic factor (BDNF), phosphorylated tropomyosin receptor kinase B (p-TrkB), and phosphorylated c-AMP response element binding protein (p-CREB) compared with the untreated CUMS group. Overall, HT improved CUMS-induced depressive-like behaviors in mice by alleviating oxidative stress and neuroinflammation and by enhancing the BDNF/TrkB/CREB signaling pathway.
1. Introduction
Depression is a common psychiatric disorder characterized by loss of pleasure or interest, feelings of worthlessness, disturbed sleep or appetite, and poor concentration.1 The World Health Organization estimates that more than 350 million people of all ages suffer from depression. It is predicted that, by 2030, depression will become the leading cause of disability, as measured in disability-adjusted life-years.2 The etiological mechanism of depression is complicated, and current treatments remain ineffective in a large subset of patients.3 It is estimated that only 50% of patients with depression respond to currently available antidepressants.4 Thus, finding new molecular targets and more effective therapeutic drugs to cure depression is urgent.
Accumulating data show that an inverse association exists between Mediterranean diet intervention and the risk of depression.5–7 The Mediterranean diet is a green and healthy diet that uses olive oil as the main edible oil and includes large amounts of whole grains, vegetables and fruits; appropriate amounts of seafood and nuts; and supplementation with appropriate amounts of dairy and dairy products, red wine, and small amounts of red meat.8 Olive oil contains many bioactive components, such as monounsaturated fatty acids, tocopherols, and polyphenols. Among the components of polyphenols, hydroxytyrosol [2-(3,4-di-hydroxyphenyl)ethanol, or HT] is one of the main phenolic compounds.9 Its chemical structure and molecular formula are shown in Fig. 1. Recent studies have shown that HT exhibits good pharmacological activities, such as antibacterial, anti-inflammatory, and antioxidant activities; prevents metabolic syndrome (obesity, dyslipidemia); and has neuroprotective properties.10–12 HT supplementation greatly improved the cognitive function of TgCRND8 mice and dramatically reduced Aβ42 and pE3-Aβ plaque areas and numbers in the cortex in a murine model of amyloid-β deposition.13 Treatment with HT ameliorated neuronal impairment via modulation of mitochondrial function, oxidative stress, and neuroinflammation, and HT improved cognitive dysfunction in a murine model of Alzheimer's disease.14 HT also improved prenatal, stress-induced cognitive impairment in rat offspring through neuroprotective effects.15
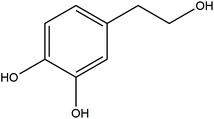 |
| Fig. 1 Chemical structure and formula of HT. | |
To date, no study to our knowledge has explored the antidepressant effects of HT in a model of stress-induced depression. In this study, we established a depression model by exposing mice to chronic unpredictable mild stress (CUMS) and tested the effects of HT on depressive-like behaviors. In addition, we examined the mechanisms underlying the effects of HT.
2. Materials and methods
2.1. Reagents
HT (S25176) was obtained from Shanghai Yuan Ye Biotechnology Co., Ltd (Shanghai, China). A reactive oxygen species (ROS) fluorescent probe, 2′,7′-dichlorofluorescindiacetate (DCFDA, C2938), was obtained from Molecular Probes (Carlsbad, CA, USA). Superoxide dismutase (SOD, A001-3) and malondialdehyde (MDA, A003-2) kits were purchased from Nanjing Jiancheng Biotechnology Co., Ltd (Nanjing, China). Interleukin 1 beta (IL-1β, BMS6002) and tumor necrosis factor alpha (TNF-α, 88732422) ELISA kits were purchased from Invitrogen (Carlsbad, CA, USA). Anti-Iba-1 (0805K02) antibody was obtained from Wako (Chuo-Ku, Japan). Anti-glial fibrillary acidic protein (GFAP, 7260) and anti-brain-derived neurotrophic factor (BDNF, 108319) antibodies were obtained from Abcam (Cambridge, MA, USA). Antibodies against tropomyosin receptor kinase B (TrkB, 4603), phosphorylated TrkB (p-TrkB, 4619), c-AMP response element binding protein (CREB, 9197) and phosphorylated CREB (p-CREB, 9198) were purchased from Cell Signaling Technology (Danvers, MA, USA).
2.2. Animals
C57BL/6 mice (male, 7–8 weeks old, 20–22 g) were purchased from Guangdong Medical Laboratory Animal Center (Guangzhou, China). The animals were housed, five per cage, with food and water ad libitum, and maintained on a 12 h light/dark cycle (light on from 8 AM to 8 PM) and at a controlled temperature of 21 °C ± 1 °C except when specified otherwise. All animal welfare and procedures were performed in accordance with the Guidelines for Care and Use of Laboratory Animals of Guangdong Ocean University and experiments were approved by the Animal Ethics Committee of Guangdong Ocean University (Approval No. 2018100905).
2.3. Drug treatment and CUMS procedure
The mice were randomly divided into six groups (n = 15 per group): the control group; the HT group (200 mg per kg body weight); the CUMS group; and three CUMS groups treated with HT at doses of 50, 100, or 200 mg per kg body weight. HT was dissolved in distilled water and administered by gastric gavage for 49 days. The experimental procedure is illustrated in Fig. 2A.
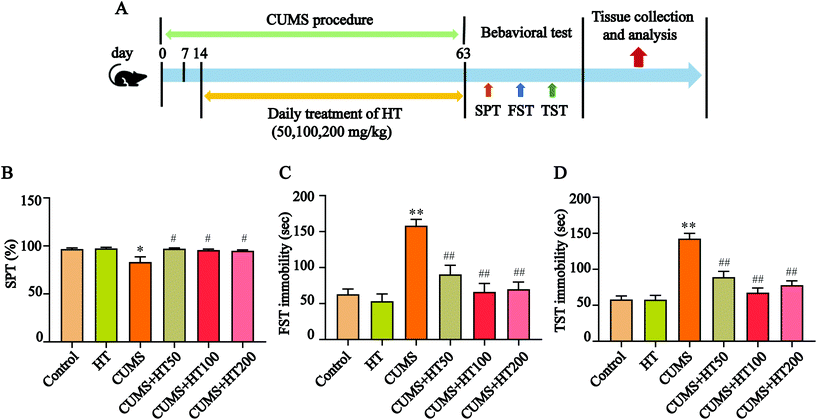 |
| Fig. 2 Experimental procedures and the results of behavioral tests. (A) Schematic illustration of the experimental process. (B) The treatment with HT obviously reversed the decreased sucrose preference observed in mice exposed to CUMS. (C and D) HT exposure significantly reduced the immobility time in the FST and TST compared with the results in the CUMS group. All data are expressed as mean ± SEM (n = 15). *P < 0.05, **P < 0.01 vs. control group; #P < 0.05, ##P < 0.01 vs. CUMS group. | |
The CUMS protocol was performed as described previously but with a slight modification.16 The mice in the control group and the HT group were housed separately throughout the experiment. The mice in the CUMS group and the CUMS + HT group were administered the following mild stressors randomly for 63 days: cold temperature (4–10 °C) for 1.5 h; restraint for 1.5 h; swimming in 18 °C cold water for 10 min; stroboscopic illumination; 45° cage tilting for 12 h; damp bedding for 12 h; food and water deprivation for 12 h; and shaking at 150 rpm for 2.5 h. All stressors were randomly applied, and the same stressor was not administered within 3 days.
2.4. Behavioral tests
2.4.1. Sucrose preference test (SPT).
Anhedonia behavior was assessed by the SPT according to the methods used in a previous report.17 Before testing, mice were supplied with one bottle of 1% sucrose water (w/v) and one bottle of purified water for 48 h. Then, the mice were deprived of food and water for 24 h. Then, at the beginning of the experiment, each mouse was again given two bottles of water: one bottle of 1% sucrose water and one bottle of purified water. After 4 h, the consumption of sucrose water and pure water was recorded, and the sucrose preference was calculated (sucrose preference = [sucrose water consumption/total water consumption] × 100%).
2.4.2. Forced swimming test (FST).
The FST was performed according to published protocols, with minor modifications.18 The experiment was carried out within 2 days. On the first day, the mice were placed in a cylindrical bucket (50 cm height, 20 cm diameter) filled with water (temperature 25 °C ± 1 °C) for 10 min. On the next day, the mice were placed in a cylindrical bucket for 5 min, and the immobility time was recorded from the second minute to the sixth minute by experimenters who were blinded to the design of the experiment.
2.4.3. Tail suspending test (TST).
The TST was performed according to published protocols, with minor modifications.19 After 9 weeks of CUMS, mice were suspended by the tail, by applying tape to the last third of the tail, at a height of 50 cm above the floor. The experiment was performed for a period of 5 min, and the duration of immobility was measured by an observer who was trained and blinded to the design of the experiment.
2.5. Measurement of ROS
The levels of ROS in the hippocampus were measured using DCFDA, as previously described, with minor modifications.20 Brain slides were incubated with DCFDA (20 μM) and dissolved with 0.1 mol L−1 phosphate buffer solution (PBS, pH 7.2) at 37 °C for 30 minutes in a dark environment. Fluorescent imaging was performed with a fluorescence microscope. Image-Pro Plus software, version 6.0, was used to analyze the optical density in each hippocampus.
2.6. Biochemical analysis for MDA and SOD in the hippocampus
Hippocampal tissue samples were homogenized (10% w/v) with PBS and centrifuged (12
000 rpm, 4 °C, 15 min). The supernatant was collected, and the protein concentration was determined with a bicinchoninic acid (BCA) assay kit. The activity of SOD and the content of MDA in the hippocampus were measured using commercial kits. All the experiment procedures followed the instructions of the commercial kits.
2.7. Measurement of inflammatory cytokines in the hippocampus
Hippocampal tissue samples were homogenized (10% w/v) with RIPA lysis buffer and centrifuged (12
000 rpm, 4 °C, 15 min). The supernatant was collected, and the protein concentration was measured with a BCA assay kit. The levels of TNF-α and IL-β in the hippocampus were determined by ELISA kits. All the experiment procedures followed the instructions of the ELISA kits.
2.8. Western blotting
Hippocampal samples were homogenized in ice-cold RIPA lysis buffer containing a mixture of a protease inhibitor and a phosphatase inhibitor and were centrifuged at 12
000 rpm for 15 min in 4 °C. Supernatants were collected and protein concentrations were determined with the BCA assay kit. Western blotting was performed as previously described, with minor modifications.21
Proteins were separated using 10% SDS-PAGE gels and transferred to polyvinylidene fluoride (PVDF) membranes. After blocking with 5% skimmed milk at room temperature for 1 h, the membranes were incubated at 4 °C overnight with primary antibodies (dilution: 1
:
1000). After washing with TBST five times, 8 min each time, the membranes were incubated with HRP-conjugated secondary antibodies (dilution: 1
:
2000) at room temperature for 1 h. Enhanced chemiluminescence (ECL) was applied on the membranes before the ChemiDoc™ XRS+ System (Bio-Rad, USA) was used. The intensity of the protein bands was normalized to β-actin.
2.9. Immunofluorescence
Mice were anesthetized with pentobarbital sodium (50 mg per kg body weight) and transcardially perfused with PBS followed by 4% paraformaldehyde. Brains were fixed overnight in 4% paraformaldehyde and then transferred to 30% sucrose for 24 h. Twenty-micrometer-thick floating sections were obtained using a microtome. Immunofluorescence was performed as previously described, with minor modifications.22 Tissue sections were washed three times in PBS, blocked in 10% normal horse serum with 0.3% Triton X-100 in PBS for 2 h at room temperature, and incubated overnight at 4 °C with the primary antibody diluted in 10% horse serum with 0.3% Triton X-100 in PBS. The sections were washed three times in PBS and then incubated for 2 h at room temperature with a fluorophore-conjugated secondary antibody and 4′,6-diamidino-2-phenylindole (DAPI) was used as the nuclear stain. After the last three washes in PBS, the sections were coverslipped with glycerol.
Images were acquired using a Leika DM2500 positive fluorescence microscope (Germany). Positive cell counting was performed using ImageJ software, version 1.8.0. Immune-positive cells were quantified in dentate gyrus per millimeter, as described.
2.10. Statistical analysis
Experimental data were expressed as the mean ± standard error of measurement (SEM) of at least three independent experiments. Statistical analyses were performed using one-way analysis of variance (Tukey procedure to compare all pairs of columns) and were conducted using GraphPad Prism Software, version 8.0 (GraphPad Software, Inc., La Jolla, CA). P < 0.05 was considered statistically significant.
3. Results
3.1. HT improved CUMS-induced depressive-like behaviors
To investigate the effects of HT on CUMS-induced depressive-like behaviors, mice were exposed to the CUMS protocol for 63 days and treated with HT for 49 days (Fig. 2A). At the end of the experiment, behavioral tests were performed. The results showed that, compared with the control mice, CUMS-induced mice showed a lower preference for sugar water in the SPT (P < 0.05, Fig. 2B) and a longer immobility time in the FST and TST (P < 0.01, Fig. 2C and D). These behavioral changes were significantly reversed by the administration of different doses of HT (50, 100, and 200 mg per kg of body weight) (P < 0.05, Fig. 2B–D).
3.2. HT alleviated oxidative stress in the hippocampus
To investigate the possible mechanisms of antidepressant effects of HT, the mice exposed to CUMS and treated with 100 mg kg−1 dose of HT were selected for an in-depth study. We first detected the changes in the oxidative stress levels in the hippocampus of mice. The results showed that ROS levels were markedly elevated in the hippocampus of CUMS mice, whereas HT treatment inhibited ROS production (P < 0.01, Fig. 3A and B). In addition, SOD activity was reduced and the MDA content was increased in the CUMS-induced group compared with those of the control group (P < 0.01, Fig. 3C and D). After treatment with HT, SOD activity increased and MDA levels decreased significantly (P < 0.01, Fig. 3C and D).
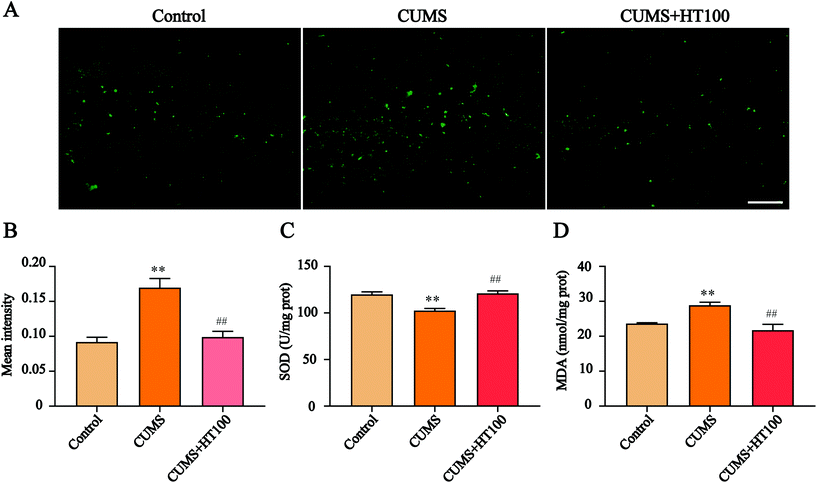 |
| Fig. 3 Effects of HT on oxidative stress in the hippocampus. (A) The distribution of ROS was observed by immunofluorescence. Scale bar = 100 μm. (B) ROS levels in the hippocampus were quantified by fluorescent analysis using Image-Pro Plus 6.0. (C and D) HT administration enhanced the activity of SOD and decreased the content of MDA in the hippocampus. All data are expressed as mean ± SEM (n = 3). **P < 0.01 vs. control group; ##P < 0.01 vs. CUMS group. | |
3.3. HT inhibited neuroinflammation in the hippocampus
The pathogenesis of depression is strongly related to neuroinflammatory processes in the hippocampus. To assess the effects of HT on neuroinflammation in the murine hippocampus, the expression of microglia labeled with Iba-1 was detected by immunofluorescence (Fig. 4A), and the levels of IL-1β and TNF-α were measured by ELISA. The number of Iba-1-labeled positive cells (P < 0.01, Fig. 4B) and the levels of IL-1β (P < 0.01, Fig. 4C) and TNF-α (P < 0.01, Fig. 4D) increased dramatically in the hippocampus of CUMS-exposed mice compared with that in the control mice. HT administration significantly decreased the number of microglia cells and the levels of IL-1β and TNF-α in the hippocampus compared to those in the CUMS-exposed mice (P < 0.01, Fig. 4B–D).
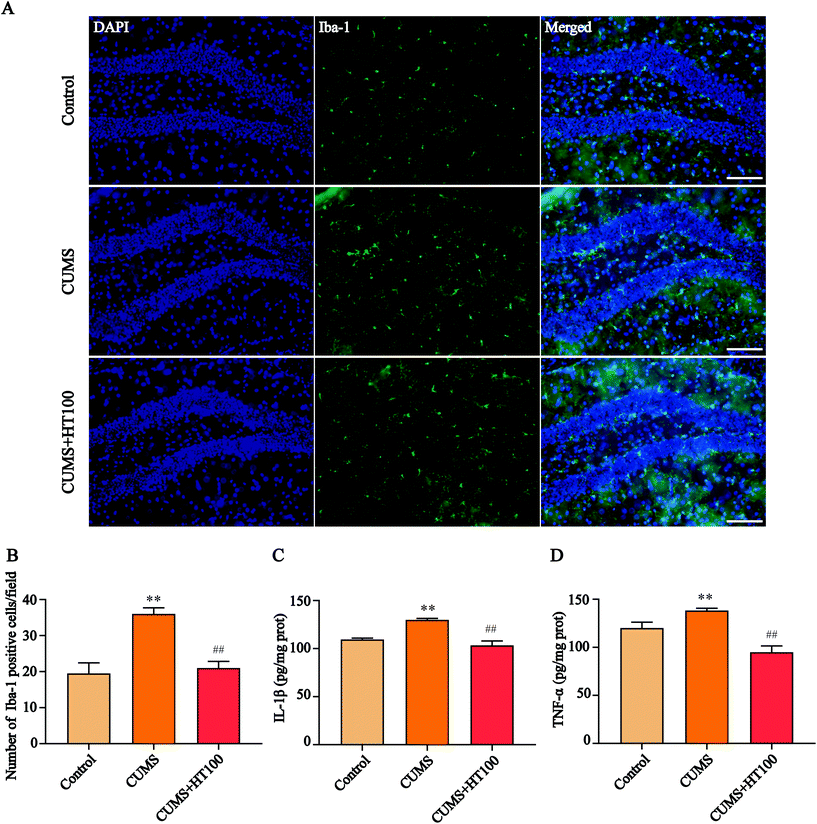 |
| Fig. 4 Effects of HT on neuroinflammation in the hippocampus. (A) Representative images of activated microglia. Tissue sections were stained using the anti-Iba-1 antibody for microglia (green). DAPI (blue) was used for nuclear staining. Scale bar = 100 μm. (B) Quantification of Iba-1-positive cells in the hippocampus. (C) HT administration significantly decreased the expression of IL-1β. (D) HT administration reduced the expression of TNF-α in the hippocampus. All data are expressed as mean ± SEM (n = 4). **P < 0.01 vs. control group; ##P < 0.01 vs. CUMS group. | |
3.4. HT increased the number of GFAP-positive cells and upregulated the level of GFAP in the hippocampus
To analyze the density of astrocytes in the murine hippocampus, the expression of the astrocyte marker GFAP in the hippocampus was measured by immunofluorescence (Fig. 5A) and western blotting (Fig. 6A). The number of GFAP-positive cells in the hippocampus observably decreased compared with those in the control group (P < 0.05, Fig. 5B). Treatment with HT significantly elevated the number of GFAP-positive cells (P < 0.05, Fig. 5B). Furthermore, western blotting results showed that HT enhanced GFAP expression (P < 0.05, Fig. 6A and B).
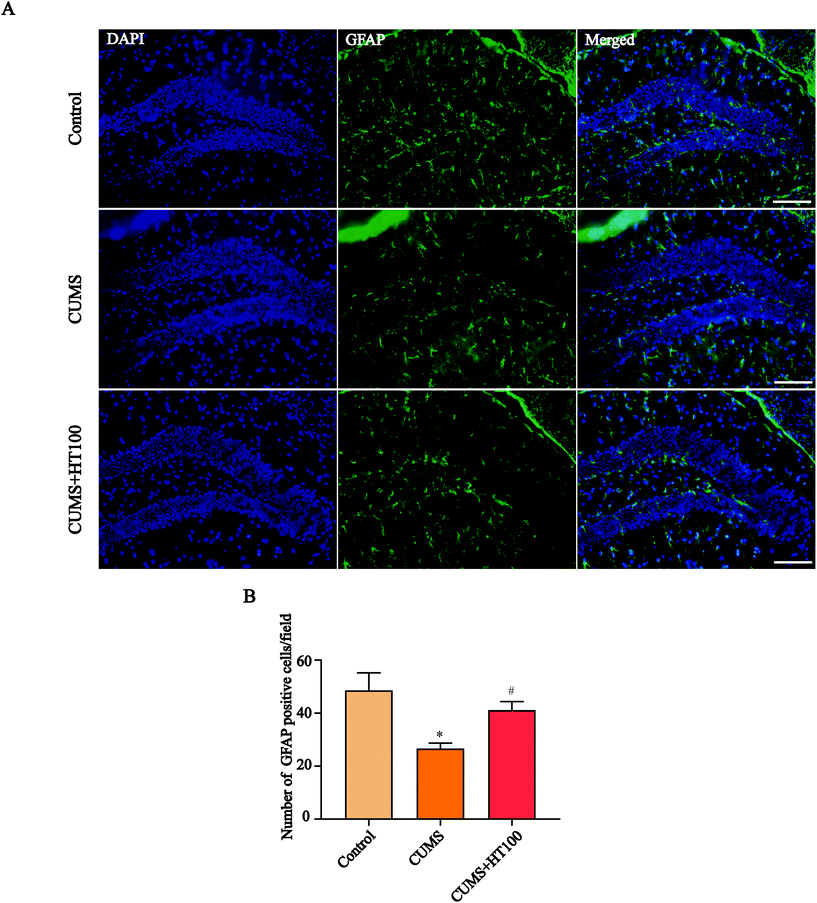 |
| Fig. 5 Effects of HT on the number of GFAP-positive cells in the hippocampus. (A) Representative images of astrocytes. Tissue sections were stained using the anti-GFAP antibody for astrocytes (green). DAPI (blue) was used for nuclear staining. Scale bar = 100 μm. (B) Quantification of GFAP-positive cells in the hippocampus. All data are expressed as mean ± SEM (n = 4). *P < 0.05 vs. control group; #P < 0.05 vs. CUMS group. | |
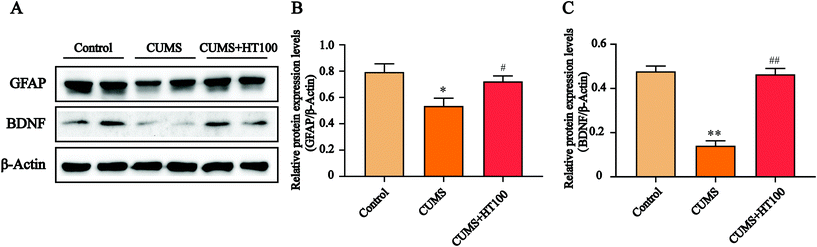 |
| Fig. 6 Effects of HT on the expression of GFAP and BDNF in the hippocampus. (A) Representative images of western blotting of GFAP and BDNF. (B and C) Quantitative analysis of GFAP and BDNF expression in the hippocampus. All data are expressed as mean ± SEM (n = 4). *P < 0.05, **P < 0.01 vs. control group; #P < 0.05, ##P < 0.01 vs. CUMS group. | |
3.5. HT enhanced the BDNF signaling pathway in the hippocampus
To better study the underlying mechanism of the effect of HT on CUMS-induced depressive-like behaviors, the levels of BDNF and related signaling proteins were measured by western blotting. The results revealed that CUMS dramatically suppressed BDNF (P < 0.01, Fig. 6A and C), p-TrkB (P < 0.01, Fig. 7A and B), and p-CREB (P < 0.01, Fig. 7A and C) in the hippocampus compared with those in the control group. HT administration reversed these changes significantly (P < 0.01, Fig. 6 and 7).
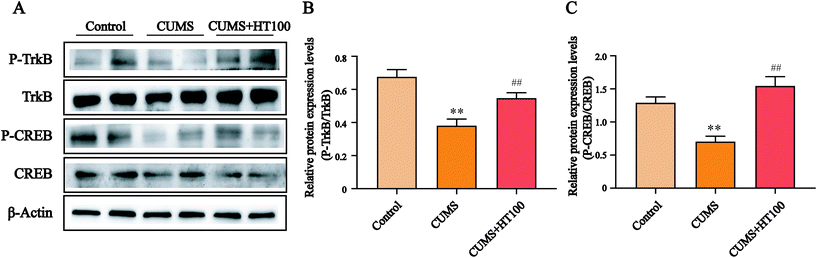 |
| Fig. 7 Effects of HT on the expression of p-TrkB and p-CREB in the hippocampus. (A) Representative images of western blotting results. (B and C) Quantification of the expression of p-TrkB and p-CREB in the hippocampus. All data are expressed as mean ± SEM (n = 4). **P < 0.01 vs. control group; ##P < 0.01 vs. CUMS group. | |
4. Discussion
The Mediterranean diet is recognized as one of the healthiest dietary patterns, conferring benefits against brain dysfunction, including neurodegenerative diseases and psychiatric disorders.23 Extra virgin olive oil is the main lipid source in the Mediterranean diet. A large body of evidence has demonstrated that the polyphenols of extra virgin olive oil can promote neurogenesis, improve learning and memory, and protect the nervous system.24,25 HT is the main polyphenol present in this oil, and HT is bioavailable for humans. It can cross the blood–brain barrier and exert neuroprotective effects.26 In this study, the potential for antidepressant effects of HT was investigated in stress-induced mice. Behavior tests showed that HT reversed depressive-like behaviors in mice exposed to CUMS, and the most effective dose of HT was 100 mg per kg of body weight. In this study, the mechanisms underlying the roles of HT in depression were studied using mice treated with 100 mg kg−1 doses of HT.
The hippocampus is a key hub within the limbic system of the brain, and its complicated structure and multiple functions ensure the biochemical integrity of the brain.27 The hippocampus is extremely sensitive to stress, and pathological changes to its structure and function form the basis of various mental disorders, including depression.28 Accumulating data show that oxidative stress, neuroinflammation, and glial cell dysfunction are involved in stress-induced hippocampal impairment and play pivotal roles in the onset and development of depression.29,30
The imbalance between ROS and antioxidants leads to oxidative stress, which will cause oxidative damage to lipids, proteins, and DNA; eventually, the damage will lead to cellular apoptosis.31,32 The brain, which consumes a lot of oxygen and is rich in lipids, is vulnerable to oxidative stress. Thus, damage to the brain caused by oxidative stress has a strong negative impact on the normal functions of the central nervous system (CNS).30 Studies have shown that oxidative stress is involved in psychiatric illnesses, including depression and anxiety disorders.33 Reduced activity of antioxidative enzymes and increased levels of oxidative stress products in the hippocampus have been reported in patients with depression.34,35 In this study, ROS and MDA levels were increased and SOD activity was decreased in the hippocampus of mice exposed to CUMS. These changes were reversed by HT treatment.
Neuroinflammation orchestrates the development of depression.36 Microglial cells are the resident immune cells of the CNS, and they are essential to maintain the homeostasis of healthy tissues and influence brain development by shaping neural circuits.37,38 Under pathological conditions, resting microglial cells convert into activated amoeba-like macrophages that release pro-inflammatory cytokines to stimulate self-proliferation, and peripheral immune cells are recruited to cause neuroinflammation, resulting in neuronal damage.39 To determine whether HT could inhibit neuroinflammation in CUMS-exposed mice, the number of hippocampal microglia cells were measured by immunofluorescence and the levels of inflammatory factors were analyzed. The total number of Iba-1-labeled positive microglia cells and the levels of IL-1β and TNF-α increased significantly in the hippocampal dentate gyrus of CUMS-exposed mice compared with those in the control group. HT treatment inhibited the activation of microglia cells in the hippocampus and downregulated the levels of IL-1β and TNF-α. This study demonstrated that treatment with HT can significantly attenuate neuroinflammation in the hippocampus, and this response was associated with the normalization of depressive-like behaviors.
Astrocytes are widely distributed in the CNS and peripheral nervous system, and they play critical roles in maintaining homeostasis within the CNS, maintaining the integrity of the brain–blood barrier, and supporting neurotrophic function.40 Previous research has shown that changes in the number, morphology, and function of astrocytes are involved in the development of depression.41 Here, we found that the number of astrocytes in the hippocampus of CUMS-exposed mice decreased significantly compared with the number in the control group. HT treatment significantly reversed this CUMS-induced decrease of astrocytes in the hippocampus.
Astrocytes can release BDNF, nerve growth factor (NGF), glial cell line-derived neurotrophic factor (GDNF), and other neurotrophins, all of which play a crucial role in neurogenesis, neuronal differentiation, neuronal survival, and synapse formation.42,43 A systematic review of clinical studies has suggested that BDNF is directly involved in the pathophysiology of depression and that the restoration of BDNF function may underlie the therapeutic efficacy of antidepressant treatment.44 Here, our results revealed that the BDNF expression level decreased in the hippocampus of CUMS-exposed mice compared with the level in the control group. HT treatment subsequently elevated the BDNF protein level. BDNF exerts anti-apoptotic and neuroprotective effects, in part via the BDNF/TrkB/CREB signaling pathway.45 BDNF binds with its receptor, TrkB, which leads to autophosphorylation of the tyrosine residues of TrkB; p-TrkB then phosphorylates CREB. CREB is a major transcription factor involved in neurogenesis, nerve survival, and brain function; it regulates the transcription of BDNF and influences cognitive function.21,46 To better explore the underlying mechanisms of the beneficial effects of HT, we measured the protein levels of p-TrkB and p-CREB in the hippocampus and found that CUMS can lead to downregulation of the BDNF/TrkB/CREB signaling pathway. Treatment with HT enhanced the expression of p-TrkB and p-CREB. Therefore, we conclude that the antidepressant effect of HT may be related to activation of the BDNF/TrkB/CREB pathway.
5. Conclusion
In summary, this study demonstrates that HT reverses CUMS-induced depressive-like behaviors. HT exerts antidepressant-like effects by reducing oxidative stress, suppressing neuroinflammation, and enhancing the function of neurotrophic factors (Fig. 8). HT might serve as a therapeutic agent in depression.
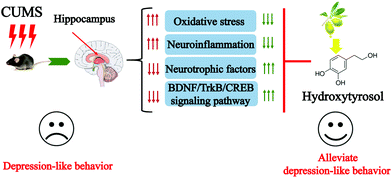 |
| Fig. 8 Mechanism underlying the antidepressant effect of HT. | |
Author contributions
Conceptualization: Yun-Tao Zhao. Data curation: Lulu Zhang and Ling Shen. Investigation: Lulu Zhang, Kun Zhang, Haowen Yin, Wenjing Zheng and Jian Zeng. Resources: Chuanyin Hu, You Liu and Yun-Tao Zhao. Preparation of the original draft: Yun-Tao Zhao and Lulu Zhang.
Conflicts of interest
The authors declare no conflict of interest.
Acknowledgements
The present study was supported by grants from the National Science Foundation of Guangdong Province (2017A030307001) and the National Key R&D Program of China (2019YFD0901800).
References
- Y. Zhang, L. Liu, Y. Z. Liu, X. L. Shen, T. Y. Wu, T. Zhang, W. Wang, Y. X. Wang and C. L. Jiang, NLRP3 Inflammasome Mediates Chronic Mild Stress-Induced Depression in Mice via Neuroinflammation, Int. J. Neuropsychopharmacol., 2015, 18, pyv006 CrossRef PubMed.
- G. S. Malhi and J. J. Mann, Depression, Lancet, 2018, 392, 2299–2312 CrossRef.
- C. Menard, G. E. Hodes and S. J. Russo, Pathogenesis of depression: Insights from human and rodent studies, Neuroscience, 2016, 321, 138–162 CrossRef CAS.
- M. S. Antunes, J. R. Ruff, D. de Oliveira Espinosa, M. B. Piegas, M. L. de Brito, K. A. Rocha, M. G. de Gomes, A. T. Goes, L. C. Souza, F. Donato, S. P. Boeira and C. R. Jesse, Neuropeptide Y administration reverses tricyclic antidepressant treatment-resistant depression induced by ACTH in mice, Horm. Behav., 2015, 73, 56–63 CrossRef CAS PubMed.
- F. Shafiei, A. Salari-Moghaddam, B. Larijani and A. Esmaillzadeh, Adherence to the Mediterranean diet and risk of depression: a systematic review and updated meta-analysis of observational studies, Nutr. Rev., 2019, 77, 230–239 CrossRef PubMed.
- A. Sanchez-Villegas, M. A. Martinez-Gonzalez, R. Estruch, J. Salas-Salvado, D. Corella, M. I. Covas, F. Aros, D. Romaguera, E. Gomez-Gracia, J. Lapetra, X. Pinto, J. A. Martinez, R. M. Lamuela-Raventos, E. Ros, A. Gea, J. Warnberg and L. Serra-Majem, Mediterranean dietary pattern and depression: the PREDIMED randomized trial, BMC Med., 2013, 11, 208 CrossRef PubMed.
- T. Psaltopoulou, T. N. Sergentanis, D. B. Panagiotakos, I. N. Sergentanis, R. Kosti and N. Scarmeas, Mediterranean diet, stroke, cognitive impairment, and depression: A meta-analysis, Ann. Neurol., 2013, 74, 580–591 CrossRef.
- C. Davis, J. Bryan, J. Hodgson and K. Murphy, Definition of the Mediterranean Diet; a Literature Review, Nutrients, 2015, 7, 9139–9153 CrossRef CAS PubMed.
- P. Ditano-Vázquez, J. D. Torres-Peña, F. Galeano-Valle, A. I. Pérez-Caballero, P. Demelo-Rodríguez, J. Lopez-Miranda, N. Katsiki, J. Delgado-Lista and L. A. Alvarez-Sala-Walther, The Fluid Aspect of the Mediterranean Diet in the Prevention and Management of Cardiovascular Disease and Diabetes: The Role of Polyphenol Content in Moderate Consumption of Wine and Olive Oil, Nutrients, 2019, 11, 2833 CrossRef PubMed.
- A. Lopez-Jimenez, E. Gallardo, J. L. Espartero, A. Madrona, A. R. Quesada and M. A. Medina, Comparison of the anti-angiogenic potential of hydroxytyrosol and five derivatives, Food Funct., 2018, 9, 4310–4316 RSC.
- M. Robles-Almazan, M. Pulido-Moran, J. Moreno-Fernandez, C. Ramirez-Tortosa, C. Rodriguez-Garcia, J. L. Quiles and M. Ramirez-Tortosa, Hydroxytyrosol: Bioavailability, toxicity, and clinical applications, Food Res. Int., 2018, 105, 654–667 CrossRef CAS PubMed.
- L. Zhang, J. Zhang, X. Jiang, L. Yang, Q. Zhang, B. Wang, L. Cui and X. Wang, Hydroxytyrosol Inhibits LPS-Induced Neuroinflammatory Responses via Suppression of TLR-4-Mediated NF-kappaB P65 Activation and ERK Signaling Pathway, Neuroscience, 2020, 426, 189–200 CrossRef CAS PubMed.
- P. Nardiello, D. Pantano, A. Lapucci, M. Stefani and F. Casamenti, Diet Supplementation with Hydroxytyrosol Ameliorates Brain Pathology and Restores Cognitive Functions in a Mouse Model of Amyloid-beta Deposition, J. Alzheimer's Dis., 2018, 63, 1161–1172 CAS.
- Y. Peng, C. Hou, Z. Yang, C. Li, L. Jia, J. Liu, Y. Tang, L. Shi, Y. Li, J. Long and J. Liu, Hydroxytyrosol mildly improve cognitive function independent of APP processing in APP/PS1 mice, Mol. Nutr. Food Res., 2016, 60, 2331–2342 CrossRef CAS PubMed.
- A. Zheng, H. Li, K. Cao, J. Xu, X. Zou, Y. Li, C. Chen, J. Liu and Z. Feng, Maternal hydroxytyrosol administration improves neurogenesis and cognitive function in prenatally stressed offspring, J. Nutr. Biochem., 2015, 26, 190–199 CrossRef CAS PubMed.
- A. Surget, A. Tanti, E. D. Leonardo, A. Laugeray, Q. Rainer, C. Touma, R. Palme, G. Griebel, Y. Ibarguen-Vargas, R. Hen and C. Belzung, Antidepressants recruit new neurons to improve stress response regulation, Mol. Psychiatry, 2011, 16, 1177–1188 CrossRef CAS PubMed.
- J. Geng, J. Liu, X. Yuan, W. Liu and W. Guo, Andrographolide triggers autophagy-mediated inflammation inhibition and attenuates chronic unpredictable mild stress (CUMS)-induced depressive-like behavior in mice, Toxicol. Appl. Pharmacol., 2019, 379, 114688 CrossRef CAS.
- R. Yankelevitch-Yahav, M. Franko, A. Huly and R. Doron, The forced swim test as a model of depressive-like behavior, J. Visualized Exp., 2015, 97, 52587 Search PubMed.
- J. F. Cryan, C. Mombereau and A. Vassout, The tail suspension test as a model for assessing antidepressant activity: review of pharmacological and genetic studies in mice, Neurosci. Biobehav. Rev., 2005, 29, 571–625 CrossRef CAS.
- W. Wang, L. Zheng, L. Xu, J. Tu and X. Gu, Pinocembrin mitigates depressive-like behaviors induced by chronic unpredictable mild stress through ameliorating neuroinflammation and apoptosis, Mol. Med., 2020, 26, 53 Search PubMed.
- C. Yin, Y. Deng, Y. Liu, J. Gao, L. Yan and Q. Gong, Icariside II Ameliorates Cognitive Impairments Induced by Chronic Cerebral Hypoperfusion by Inhibiting the Amyloidogenic Pathway: Involvement of BDNF/TrkB/CREB Signaling and Up-Regulation of PPARalpha and PPARgamma in Rats, Front. Pharmacol., 2018, 9, 1211 CrossRef CAS PubMed.
- R. X. Liu, J. Ma, B. Wang, T. Tian, N. Guo and S. J. Liu, No DCX-positive neurogenesis in the cerebral cortex of the adult primate, Neural Regener. Res., 2020, 15, 1290–1299 CrossRef PubMed.
- A. K. Kiani, G. A. D. Miggiano, B. Aquilanti, V. Velluti, G. Matera, L. Gagliardi and M. Bertelli, Food supplements based on palmitoylethanolamide plus hydroxytyrosol from olive tree or Bacopa monnieri extracts for neurological diseases, Acta Biomed., 2020, 91, e2020007 CAS.
- C. Angeloni, M. Malaguti, M. C. Barbalace and S. Hrelia, Bioactivity of Olive Oil Phenols in Neuroprotection, Int. J. Mol. Sci., 2017, 18, 2230 CrossRef PubMed.
- M. Leri, M. Scuto, M. L. Ontario, V. Calabrese, E. J. Calabrese, M. Bucciantini and M. Stefani, Healthy Effects of Plant Polyphenols: Molecular Mechanisms, Int. J. Mol. Sci., 2020, 21, 1250 CrossRef CAS PubMed.
- M. Gallardo-Fernandez, R. Hornedo-Ortega, A. B. Cerezo, A. M. Troncoso and M. C. Garcia-Parrilla, Melatonin, protocatechuic acid and hydroxytyrosol effects on vitagenes system against alpha-synuclein toxicity, Food Chem. Toxicol., 2019, 134, 110817 CrossRef CAS PubMed.
- N. V. Gulyaeva, Functional Neurochemistry of the Ventral and Dorsal Hippocampus: Stress, Depression, Dementia and Remote Hippocampal Damage, Neurochem. Res., 2019, 44, 1306–1322 CrossRef CAS PubMed.
- N. V. Gulyaeva, The Neurochemistry of Stress: the Chemistry of the Stress Response and Stress Vulnerability, Neurochem. J., 2018, 12, 117–120 CrossRef CAS.
- Q. Q. Yang and J. W. Zhou, Neuroinflammation in the central nervous system: Symphony of glial cells, Glia, 2019, 67, 1017–1035 CrossRef PubMed.
- S. Salim, Oxidative Stress and the Central Nervous System, J. Pharmacol. Exp. Ther., 2017, 360, 201–205 CrossRef CAS PubMed.
- A. Kandola, G. Ashdown-Franks, J. Hendrikse, C. M. Sabiston and B. Stubbs, Physical activity and depression: Towards understanding the antidepressant mechanisms of physical activity, Neurosci. Biobehav. Rev., 2019, 107, 525–539 CrossRef.
- Y. B. Joshi and D. Pratico, Lipid peroxidation in psychiatric illness: overview of clinical evidence, Oxid. Med. Cell. Longevity, 2014, 2014, 828702 Search PubMed.
- E. Bouvier, F. Brouillard, J. Molet, D. Claverie, J. H. Cabungcal, N. Cresto, N. Doligez, C. Rivat, K. Q. Do, C. Bernard, J. J. Benoliel and C. Becker, Nrf2-dependent persistent oxidative stress results in stress-induced vulnerability to depression, Mol. Psychiatry, 2017, 22, 1701–1713 CrossRef CAS.
- F. Salehpour, F. Farajdokht, P. Cassano, S. Sadigh-Eteghad, M. Erfani, M. R. Hamblin, M. M. Salimi, P. Karimi, S. H. Rasta and J. Mahmoudi, Near-infrared photobiomodulation combined with coenzyme Q10 for depression in a mouse model of restraint stress: reduction in oxidative stress, neuroinflammation, and apoptosis, Brain Res. Bull., 2019, 144, 213–222 CrossRef CAS.
- X. Li, T. Wu, Z. Yu, T. Li, J. Zhang, Z. Zhang, M. Cai, W. Zhang, J. Xiang and D. Cai, Apocynum venetum leaf extract reverses depressive-like behaviors in chronically stressed rats by inhibiting oxidative stress and apoptosis, Biomed. Pharmacother., 2018, 100, 394–406 CrossRef CAS PubMed.
- S. Najjar, D. M. Pearlman, K. Alper, A. Najjar and O. Devinsky, Neuroinflammation and psychiatric illness, J. Neuroinflammation, 2013, 10, 43 CAS.
- G. Z. Reus, G. R. Fries, L. Stertz, M. Badawy, I. C. Passos, T. Barichello, F. Kapczinski and J. Quevedo, The role of inflammation and microglial activation in the pathophysiology of psychiatric disorders, Neuroscience, 2015, 300, 141–154 CrossRef CAS.
- L. Janks, C. V. R. Sharma and T. M. Egan, A central role for P2X7 receptors in human microglia, J. Neuroinflammation, 2018, 15, 325 CrossRef CAS PubMed.
- R. Yirmiya, N. Rimmerman and R. Reshef, Depression as a microglial disease, Trends Neurosci., 2015, 38, 637–658 CrossRef CAS PubMed.
- L. Leng, K. Zhuang, Z. Liu, C. Huang, Y. Gao, G. Chen, H. Lin, Y. Hu, D. Wu, M. Shi, W. Xie, H. Sun, Z. Shao, H. Li, K. Zhang, W. Mo, T. Y. Huang, M. Xue, Z. Yuan, X. Zhang, G. Bu, H. Xu, Q. Xu and J. Zhang, Menin Deficiency Leads to Depressive-like Behaviors in Mice by Modulating Astrocyte-Mediated Neuroinflammation, Neuron, 2018, 100, 551–563 CrossRef CAS.
- I. P. Karve, J. M. Taylor and P. J. Crack, The contribution of astrocytes and microglia to traumatic brain injury, Br. J. Pharmacol., 2016, 173, 692–702 CrossRef CAS PubMed.
- E. Palasz, W. Niewiadomski, A. Gasiorowska, A. Mietelska-Porowska and G. Niewiadomska, Neuroplasticity and Neuroprotective Effect of Treadmill Training in the Chronic Mouse Model of Parkinson's Disease, Neural Plast., 2019, 2019, 8215017 Search PubMed.
- C. Cao, J. Xiao, M. Liu, Z. Ge, R. Huang, M. Qi, H. Zhu, Y. Zhu and J. A. Duan, Active components, derived from Kai-xin-san, a herbal formula, increase the expressions of neurotrophic factor NGF and BDNF on mouse astrocyte primary cultures via cAMP-dependent signaling pathway, J. Ethnopharmacol., 2018, 224, 554–562 CrossRef CAS.
- T. Rana, T. Behl, A. Sehgal, P. Srivastava and S. Bungau, Unfolding the Role of BDNF as a Biomarker for Treatment of Depression, J. Mol. Neurosci., 2020, 5, 1–14 Search PubMed.
- T. Odaira, O. Nakagawasai, K. Takahashi, W. Nemoto, W. Sakuma, J. R. Lin and K. Tan-No, Mechanisms underpinning AMP-activated protein kinase-related effects on behavior and hippocampal neurogenesis in an animal model of depression, Neuropharmacology, 2019, 150, 121–133 CrossRef CAS.
- Y. Shang, X. Wang, F. Li, T. Yin, J. Zhang and T. Zhang, rTMS Ameliorates Prenatal Stress-Induced Cognitive Deficits in Male-Offspring Rats Associated With BDNF/TrkB Signaling Pathway, Neurorehabilit. Neural Repair, 2019, 33, 271–283 CrossRef.
Footnote |
† These authors contributed equally to this work. |
|
This journal is © The Royal Society of Chemistry 2021 |
Click here to see how this site uses Cookies. View our privacy policy here.