DOI:
10.1039/D0FO02581J
(Paper)
Food Funct., 2021,
12, 1529-1546
Fermentation of Pleurotus ostreatus and Ganoderma lucidum mushrooms and their extracts by the gut microbiota of healthy and osteopenic women: potential prebiotic effect and impact of mushroom fermentation products on human osteoblasts†
Received
1st October 2020
, Accepted 5th January 2021
First published on 5th January 2021
Abstract
Recent data have highlighted the role of the gut microbiota and its several metabolites in maintaining bone health. Thus, gut microbiota manipulation, e.g., by prebiotics, might offer a plausible target in the fight against bone degenerative diseases. This study aimed (a) to investigate the in vitro prebiotic potential of Ganoderma lucidum and Pleurotus ostreatus mushrooms in healthy and osteopenic women and (b) to explore the impact of mushroom fermentation products on human osteoblasts. G. lucidum LGAM 9720 and P. ostreatus IK 1123 lyophilized mushroom-powders (2% w/v) and their hot-water extracts (1% w/v) were fermented in a 24 h static batch culture model by using faecal inocula from healthy (n = 3) or osteopenic (n = 3) donors. Gut microbiota analysis (qPCR) and measurement of short chain fatty acids (SCFAs) were performed during fermentation, and 24 h-prebiotic indexes were calculated. Evaluation of the effects of fermentation products on bone metabolism parameters (OPG: osteoprotegerin; and RANKL: receptor activator of nuclear factor kappa B ligand) in osteoblast cultures was also performed. Our data suggest that the origin of the gut microbiota inoculum plays a major role in the viability of osteoblasts. The treatments using P. ostreatus mushroom-powder and G. lucidum mushroom-extract had positive effects based on gut microbiota and SCFA analyses. Both mushrooms exhibited lower RANKL levels compared to controls, whereas their extracts tended to enhance the osteoblastic activity. In conclusion, mushrooms that are rich in beta-glucans may exert beneficial in vitro effects on bone physiology by alterations in the gut microbiota and/or SCFA production.
1 Introduction
The human body is the natural habitat of a large number and variety of microorganisms including bacteria, eukaryotes, archaea and viruses, widely known as “gut microbiota”. While the composition of an individual's gut microbiota is unique, it usually remains relatively stable during adult life and it may be altered by various factors including host immune status, gender-specific hormones, age, diet and use of antibiotics.1,2 The most dominant phyla detected in the gut microbiota are Firmicutes and Bacteroidetes, whereas Proteobacteria, and Actinobacteria are traced in minor proportions.3 Dysbiosis of the gut microbiota is indicative of various chronic and pathological conditions, such as obesity,4 inflammatory bowel disease,5 irritable bowel syndrome,6 allergies, cancer,7 non-intestinal autoimmune diseases,8 cardiovascular diseases9 and neurological disorders.10 Female postmenopausal osteopenia and osteoporosis are common skeletal diseases leading to fractures and disability, underlying the impact of estrogen deficiency on the skeleton. Declining estrogen levels result in a potent stimulation of bone resorption/osteoclastogenesis combined with a lower rate of bone formation/osteoblastogenesis leading to a period of rapid bone loss.11 There is no single cause of osteoporosis and multiple mechanisms are involved in the pathogenesis of osteopenia. Decreased bone density has been recently correlated with gut microbiota dysregulation whereas several mechanisms have been described to support a gut–bone axis.12 Research data have highlighted the role of the gut microbiota and its several metabolites (e.g. SCFAs) in maintaining bone health and bone mass through mechanisms including immune regulation, nutrient acquisition (calcium and phosphate), effects on gut serotonin or estrogen-like molecule production and alterations in gut barrier integrity and permeability.13–15 “osteomicrobiology” combines bone physiology, gastroenterology, immunology and microbiology in order to define the connection of the gut microbiota and bone beyond simply facilitating the absorption of minerals that are important for bone health.16
Diet is one of the major factors that affects the gut microbiota composition.17 According to the 2017 ISAPP consensus statement about the definition and scope of prebiotics, “a prebiotic is a substrate that is selectively utilized by host microorganisms conferring a health benefit”.17 The main fermentation products are short chain fatty acids (SCFAs), which have an important role in host's metabolism, beyond the gut environment.17 Recent accumulating data from animal and human trials have underlined the beneficial role of prebiotics on bone mass density, and/or calcium and magnesium absorption, and/or impact of bone turnover markers.15,18 Increased calcium absorption has been noticed in adolescents19–24 and postmenopausal women25–27 after consumption of prebiotics. In other studies, supplementation with calcium and prebiotics affected the bone turnover markers while it did not change the bone mineral density.28–30 Recently, research has been focused on finding new alternative sources of prebiotics, with evidence-based effects on bone health.31 So far mushrooms have demonstrated such a great potential, due to their high content of beta-glucans.32,33 Particularly, mushrooms produced by Ganoderma and Pleurotus species are well known for their health-beneficial properties, due to their antioxidant, immunoregulatory, anti-hypertensive, anti-inflammatory, hypocholesterolemic, anti-diabetic and prebiotic activities.34,35 Thus, gut microbiota manipulation by prebiotics of fungal origin might offer a plausible target in the fight against bone degenerative diseases by focusing on the bone remodeling cycle.
In this context, we aimed to investigate the in vitro prebiotic impact of Ganoderma lucidum and Pleurotus ostreatus mushrooms and their extracts on the gut microbiota of healthy and osteopenic women. Moreover the effects of the in vitro batch-culture fermentation products of G. lucidum and P. ostreatus on human osteoblasts were assessed by using the two important and highly interesting bone turnover markers osteoprotegerin (OPG) and human receptor activator of nuclear factor kappa-B ligand (RANKL).
2 Materials and methods
2.1 Characteristics of faecal donors
This study was conducted in Athens, Greece and the faecal donors were 6 postmenopausal women (3 healthy and 3 osteopenic) aged 55 to 65 years who met the following inclusion criteria: (a) normal weight [Body Mass Index (BMI) < 30 kg (m−2)] under no weight-loss program, (b) no consumption of antibiotics in the last two months prior to the study, (c) no consumption of dietary supplements related to bone metabolism (calcium, vitamin D, and cod oil) in the last six months prior to the study, (d) no history of chronic or autoimmune diseases (e.g. Idiopathic Inflammatory Bowel Disease, Irritable Bowel Syndrome, any form of constipation or diarrhea, kidney disease, liver disease, cardiovascular disease, cancer or any form of parathyroid disease) and (e) no consumption of dietary supplements or fortified foods that could affect the intestinal microbiota such as probiotics, prebiotics and symbiotics. This study was conducted according to the guidelines laid down in the Declaration of Helsinki and all procedures involving human subjects were approved by the Bioethics Committee of Harokopio University. Written informed consent was obtained from all subjects before their inclusion in the study (Approval Number: 58/10-11-2017). A flowchart of the experiments performed in the frame of this study is presented in the ESI (Fig. S1†).
2.2 Demographic, anthropometric, dietary and physical assessment
The faecal donors completed questionnaires (in the presence of an expert) related to sociodemographic parameters (including age, sex, marital status and education level), smoking habits, medical history and gastrointestinal symptomatology for the 7 days preceding stool collection. Their body weight and height were self-reported and the Body Mass Index (BMI) was calculated by dividing the weight (kg) by the squared height (m2). At the baseline, individual habitual energy intakes of the participants were assessed by a dietitian using 3-d food diaries, and their physical activity levels were assessed using the International Physical Activity Questionnaire-Short Form.36 The duration of sedentary activity (sitting or resting) expressed as ‘h week−1’ was also recorded.
2.3 Biochemical and bone density measurements
Blood samples were obtained after a 12 h overnight fasting. Blood serum analysis included the assessment of the lipidemic profile [i.e. Total Cholesterol (TC), Low-Density Lipoprotein Cholesterol (LDL-C), High-Density Lipoprotein Cholesterol (HDL-C), and Triglycerides (TG)], glucose metabolism [i.e. Fasting Blood Glucose (FBG) and insulin] and bone biomarkers [i.e. serum calcium (Ca), bone specific alkaline phosphatase (BALP), osteocalcin and 25-OH vitamin D]. HOMA-IR (Homeostatic Model Assessment of Insulin Resistance) was calculated using the formula: [fasting insulin (uIU mL−1) × fasting glucose (mg dL−1)]/22.5.37 DXA (dual energy X-ray absorptiometry) was used for the examination of bone health and diagnosis of osteopenia, based on T-score, Z-score and BMD (Bone Mass Density) (gr cm−2) evaluation.
2.4 Fungal strains, mushroom cultivation and determination of glucan contents
Ganoderma lucidum strain LGAM9720 and Pleurotus ostreatus strain LGAM1123 (maintained in the fungal culture collection of the Laboratory of General and Agricultural Microbiology, Agricultural University of Athens) were cultivated on a beech-sawdust based substrate and on a wheat-straw based substrate, respectively, as previously described.38 Mushrooms were freeze-dried in a Telstar Cryodos apparatus and milled to fine powder, which was further used either untreated (GLBS and POWS treatments for G. lucidum and P. ostreatus, respectively) or after a stage of polysaccharide extraction.
For polysaccharide extraction, the suspension of the mushroom powder in distilled H2O (1
:
40 w/v; initial volume: 400 ml) was maintained at 95 °C for 50 h. After thorough mixing and centrifugation (10
000g for 15 min at 4 °C), the supernatants were collected and subjected to condensation in a rotary evaporator to obtain a final volume of 30–40 ml and were stored at 4 °C for 24 h. Then the polysaccharides were precipitated by adding ice-cold ethanol to the samples (1
:
1 v/v), followed by stirring (200 rpm for 30 min at 4 °C) and centrifugation (10
000g for 5 min at 4 °C).39–42 The precipitate was freeze-dried for 24 h, milled to fine powder and used for the additional two treatments (GLBSE and POWSE for G. lucidum and P. ostreatus, respectively). The total glucan and alpha-glucan contents were estimated using a Mushroom and Yeast Assay Kit (Megazyme Int. Ireland). The beta-glucan content was calculated by subtracting the alpha-glucan content from the total glucan content.
2.5 Faecal sample collection and in vitro static batch culture fermentation
Stool collection was performed according to the method described by Mitsou et al.43 and the in vitro static batch culture fermentation procedure was based on the protocol of Olano-Martin et al.44 and Rycroft et al.45 with slight modifications, as previously described.46 In order to minimize the potential cytotoxicity of the basal medium, we further modified the composition by reducing or excluding some evidence-based ingredients with a cytotoxic effect (e.g. reduction of the amount of hemin and Tween® 80 and exclusion of resazurin from the recipe).47,48 The modified basal medium had comparable results with the classic basal medium in terms of in vitro fermentation capacity and microbial modifications, based on previous testing (data not shown).
In detail, the modified basal medium consisted of the following ingredients (g l−1): peptone water (Merck KGaA, Darmstadt, Germany), 2.0; yeast extract (Merck KGaA), 2; NaCl, 0.10; K2HPO4, 0.04; KH2PO4, 0.04; MgSO4·7H2O, 0.01; CaCl2·2H2O, 0.01; NaHCO3, 2.0; L-cysteine HCl (Merck KGaA), 0.50; dehydrated bile (OxgallTM, BD and Company, Sparks, MD, USA), 0.50; hemin (dissolved in some drops of 1.0 M NaOH) (Fluka, Sigma-Aldrich, Netherlands), 0.005;48 Tween® 80 (Panreac Quimica SA, Barcelona, Spain), 0.2 ml l−1 (ref. 47) and vitamin K1 (Fluka, Sigma-Aldrich, Switzerland), 10 μl l−1. The medium was adjusted to pH 7.0 with 1.0 M HCl, aliquoted in appropriate volumes into glass bottles (45 ml), sterilized at 121 °C for 15 min and transferred into an anaerobic chamber for overnight pre-reduction (BACTRON™ 1.5 Anaerobic Environmental Chamber, SHELLAB, Cornelius, Oregon). On the day of the in vitro experiment, we added 2% (w/v) untreated mushroom powders (POWS and GLBS) or 1% (w/v) mushroom hot-water extracts (POWSE and GLBSE) to the basal medium aliquots. Positive controls with already proven prebiotic effect were used (inulin by Orafti® GR, BENEO-Orafti, Oreye, Belgium), i.e., 1% w/v inulin (INU1) for POWSE and GLBSE, and 2% w/v inulin (INU2) for POWS and GLBS. A negative control (NC; a basal medium with no carbohydrate source) was also included in the experiment.
On the day of the in vitro experiment we prepared a faecal slurry (20%w/v) in PBS, pH 7.3 (8.0 g l−1 NaCl, 0.2 g l−1 KCl, 1.15 g l−1 Na2HPO4, and 0.2 g l−1 KH2PO4).49 From this slurry, 10% (v/v) inocula were transferred into the pre-reduced basal medium aliquots of the substrates or the controls. The static batch cultures were incubated for 24 h under anaerobic conditions at 37 °C. The samples were collected at the baseline (0 h), and after 8 h and 24 h of fermentation and stored at −80 °C until further analysis (gut microbiota and SCFA profiling). In addition, the samples were collected, centrifuged, filtered (0.22 μm) and stored at −20 °C at 0 h and 24 h for MG-63 cell line experiments, as described in the following sections.
2.6 Gut microbiota analysis
Enumeration of the total bacterial load and selected members of the gut microbiota (e.g. Bacteroides spp., Clostridium perfringens group, Bifidobacterium spp., Lactobacillus group, Roseburia spp.–Eubacterium rectale, and Faecalibacterium prausnitzii) at the baseline (0 h) and after 24 h of fermentation was performed by real-time quantitative PCR (qPCR), as previously described.43,46 In detail, genomic DNA was extracted from the frozen sample (1 ml, −80 °C) according to the method described by Salonen et al.50,51 using the QIAamp® DNA Mini Kit (QIAGEN GmbH). Quantitative real-time PCR based on SYBR Green I detection chemistry was used to characterize the gut microbiota using species-, genus- and group-specific primers targeting 16S rRNA genes of different bacterial groups and the KAPA SYBR® Fast Master Mix (2×) Universal Kit (Kapa Biosystems Inc.) (ESI, Table S1†). PCR amplification and detection were performed using a LightCycler® 2.0 Real-Time PCR System (Roche Diagnostics GmbH). Microbial quantification was performed based on the standard curves for genomic DNA from the reference strains using the LightCycler® software version 4.1 (Roche Diagnostics GmbH). Data were expressed as log10 copies of 16S rRNA gene per ml of sample.
2.7 Prebiotic indexes
The prebiotic potential of the tested substrates was qualitatively evaluated based on the calculation of prebiotic indexes (PIs) after 24 h of fermentation, as previously described.46 PI is a useful tool for the comparison of prebiotic efficiency52 and is calculated using the following equation,53 based on quantification of bacteria (copies of 16S rRNA gene per ml of sample):
PI = (Bif/Total) − (Bac/Total) + (Lac/Total) − (Clos/Total) |
where Bif refers to Bifidobacterium spp. numbers after 24 h of fermentation (t = 24 h)/numbers at inoculation (t = 0 h), Bac refers to Bacteroides spp. numbers after 24 h of fermentation (t = 24 h)/numbers at inoculation (t = 0 h), Lac refers to Lactobacillus group numbers after 24 h of fermentation (t = 24 h)/numbers at inoculation (t = 0 h), Clos refers to Clostridium perfringens group numbers after 24 h of fermentation (t = 24 h)/numbers at inoculation (t = 0 h) and Total refers to total bacteria numbers after 24 h of fermentation (t = 24 h)/numbers at inoculation (t = 0 h). According to the prebiotic index equation, an increase in the population of bifidobacteria and/or lactobacilli is assumed as a positive effect and an increase in bacteroides and/or clostridia is assumed as a negative effect.53 This prebiotic index equation offers the advantage of normalizing the microbial population changes in relation to the initial bacterial counts, accounting for the physiological variability that characterizes the experimental process of the in vitro fermentation.53
2.8 Measurement of SCFAs
Short-chain fatty acid (SCFA) concentrations of the in vitro static batch cultures were determined using capillary gas chromatography (GC), as previously described54 by Mountzouris et al.55 The samples (1 ml) were centrifuged at 13
000g for 15 min at 4 °C and 300 μl of the supernatants were transferred into fresh, sterile microcentrifuge tubes and stored at −80 °C until analysis. On the day of analysis, the supernatants (300 μl) were vortexed and centrifuged at 13
000g for 5 min at room temperature. Subsequently, 85 μl of each supernatant was mixed with 10 μl of 2-ethyl-butyrate (20 mM, internal standard) (2-ethyl butyric acid 99%, Sigma-Aldrich C., USA) and 5 μl of hydrochloric acid (HCl, 1 M). 1 μl of samples were injected into a gas chromatograph (Agilent 6890GC System, Agilent Technologies) using a Supelco Nukol™ Capillary GC Column (size × I.D. 30 m × 0.25 mm, df 0.25 μm) (Sigma-Aldrich C., USA). Chromatography was performed with an injection split ratio of 1
:
25, isothermal at 185 °C with injector and detector temperatures set to 200 °C and 220 °C, respectively. The concentrations of SCFAs were computed based on instrument calibration with the SCFA standard mixture (Supelco Volatile Acid Standard mix, Sigma-Aldrich C., USA). The total volatile fatty acid (VFA) and SCFA concentrations were expressed as μmol ml−1 of sample and the molar ratios of acetate, propionate, butyrate, branched-chain SCFAs (iso-butyrate, iso-valerate, and iso-caproic acid) and other SCFAs (valerate, caproic acid and heptanoic acid) were also calculated. The differences (Δ) in the concentrations (μmol mL−1) of total VFAs, major SCFAs (acetate, propionate, and butyrate) and minor SCFAs (BSCFAs and other SCFAs) after 8 h of fermentation (ΔCt8-0) and 24 h of fermentation (ΔCt24-0) in comparison with the baseline were further calculated as previously described.46
2.9 Viability of MG-63 cell line and quantification of bone metabolism parameters
MG-63 is an osteosarcoma cell line with osteoblastic phenotype, consisting of oval-spindle shape cells without branching extensions, having a doubling rate of about 24 h, which was obtained from the American Type Culture Collection (ATCC, Bethesda, MD). This cell line was grown in 75 cm2 culture flasks at 37 °C under 5% CO2 using Roswell Park Memorial Institute-1640 Medium (RPMI-1640, Gibco, USA) containing 10% fetal bovine serum (FBS, Gibco, USA).
The fermentation supernatants that were collected initially (0 h) and after 24 h of fermentation were diluted 1
:
8 for the treatment of human MG-63 cells.56–59 Cells were seeded at a density of 1500 per well into a 96-well plate. After 24 h, the cells were treated with the fermentation supernatants for 48 h. MTT [3-(4,5-dimethylthiazol-2-yl)-2,5-diphenyltetrazolium bromide] (Sigma M-5655) was added at a concentration of 5 mg mL−1 directly to each well for 4 h at 37 °C. The medium was aspirated and the blue MTT formazan precipitate was dissolved in dimethyl sulfoxide (DMSO). The absorbance was measured at 540 nm using a Powerwave microplate spectrophotometer (Biotek Instruments, Inc., Winooski, Vermont, USA) and the cell viability results are presented as the percent of OD in the treated wells versus the controls.60
The ELISA method was used for the quantitative determination of human OPG (Osteoprotegerin) (Human OPG ELISA Kit, ref. EA100335) and human RANKL (Receptor activator of Nuclear factor kappa-B ligand) (Human TNFSF11/RANKL ELISA Kit, ref. EA100531) (OriGene Technologies Inc., MD, USA) in MG-63 cells’ supernatant, in order to identify the effect of the processed fermentation products on these parameters according to the manufacturer's protocol. The 96-well microplates filled with the cell culture medium DMEM-10% FBS were treated with the fermentation supernatants of GLBS, GLBSE, POWS and POWSE in a dilution of 1
:
8 and were incubated for 48 h, while the cell culture medium (control) was used as the negative control and inulin (INU2) as the positive control. The absorbance was measured at 450 nm using a Powerwave microplate spectrophotometer (Biotek Instruments, Inc.).
2.10 Statistical analysis
Continuous variables are presented as mean values ± standard deviation or median and interquartile range (Q1–Q3). Categorical variables are presented as frequencies. Associations between the categorical variables were tested using the chi-square test. Comparison of the tested variables (e.g. bacterial levels and SCFAs) was performed for the whole six-plicate experiment and according to the bone health status (normal, n = 3; osteopenic, n = 3), respectively. The normality of the distribution of variables was tested by the Shapiro–Wilk test. The bacterial levels (t = 0 h and 24 h) and SCFA characteristics (t = 0 h, 8 h and 24 h) were compared prospectively by Repeated Measures ANOVA (RM-ANOVA) for parametric data and by the Friedman test for non-parametric data with post hoc (Tukey's HSD test) and parameter estimates analyses. Comparisons of the bacterial levels and SCFA characteristics after each treatment (NC, INU1, INU2, POWS, POWSE, GLBS, and GLBSE) for different time periods (0 h, 8 h, and 24 h) were performed by the paired-sample t test for parametric data and by the Wilcoxon signed rank test for non-parametric data. For prebiotic indexes, the Kruskal Wallis and Mann–Whitney tests were performed. For differences in the SCFA concentrations (ΔCt8-0 or ΔCt24-0), parametric and non-parametric tests were performed (the Kruskal Wallis test, t-test, and Mann–Whitney test). The software program IBM® SPSS® Statistics version 21 was used for the statistical analysis of the results and the significance threshold was set at 5% (p < 0.05).
3 Results and discussion
Based on glucan analysis, it was found that both powdered mushrooms and their extracts were high in beta-glucan content. Specifically, the beta-glucan content of GLBS was 35.83 ± 2.05% w/w of dry weight, whereas higher levels were detected in the extract (the beta-glucan content of GLBSE: 47.70 ± 0.91% w/w of dry weight). Regarding POWS and POWSE, a similar beta-glucan content was detected in both cases (POWS: 30.64 ± 2.45% w/w of dry weight; and POWSE: 28.80% ± 0.67% w/w of dry weight), indicating that the extraction methodology was not effective at purifying beta-glucans of P. ostreatus.39–42
3.1 Descriptive characteristics of faecal donors
Healthy subjects and participants with osteopenia (faecal donors) were comparable in terms of their baseline characteristics, including sociodemographic factors, anthropometric indices, biochemical measurements, physical activity levels and dietary intake. Higher protein intake (%) in healthy subjects was probably due to seasonal differences of dietary intake. Z-Scores between the two groups were significantly different as expected (p = 0.048), even though T-scores showed no difference (p = 0.114) since the subjects with osteopenia were just diagnosed in their annual check-up (Table 1). Significantly higher levels of insulin and consequently HOMA-IR were also detected in osteopenic subjects (ESI, Table S2†).
Table 1 Descriptive characteristics of faecal donors
|
Total (n = 6) |
Normal bone health (n = 3) |
Osteopenia (n = 3) |
p-value |
BMI: Body Mass Index; BMD: Bone Mass Density; Ca: serum calcium; BALP: Bone specific Alkaline Phosphatase; values are expressed as mean and standard deviation (SD) for parametric or median and interquartile range (Q1–Q3) for non-parametric data. * significantly different (p < 0.05). |
Anthropometric measurements
|
Age (years) |
56.17 ± 3.65 |
58.33 ± 3.51 |
54.00 ± 2.65 |
0.163 |
Body weight (kg) |
63.17 ± 7.39 |
65.00 ± 10.82 |
61.33 ± 3.06 |
0.602 |
Height (m) |
1.64 ± 0.05 |
1.62 ± 0.02 |
1.65 ± 0.07 |
0.432 |
BMI (kg m−2) |
23.66 ± 2.86 |
24.80 ± 3.40 |
22.52 ± 2.22 |
0.384 |
T-Score |
−0.98 ± 1.83 |
0.20 ± 1.47 |
−2.17 ± 1.41 |
0.114 |
Z-Score |
0.03 ± 1.66 |
1.27 ± 1.07 |
−1.20 ± 1.08 |
0.048* |
BMD |
0.93 ± 0.20 |
1.08 ± 0.18 |
0.78 ± 0.72 |
0.055 |
|
Nutritional parameters
|
Total energy Intake (kcal d−1) |
2036.1 ± 718.34 |
1624.55 ± 551.64 |
2447.83 ± 690.99 |
0.182 |
Protein intake (%) |
13.20 (11.98–16.15) |
16.00 (15.10–16.30) |
12.30 (11.70–12.30) |
0.046* |
Protein intake (g d−1) |
68.89 ± 17.15 |
63.67 ± 16.55 |
74.11 ± 19.48 |
0.518 |
Carbohydrate (% of energy) |
43.80 ± 7.82 |
40.77 ± 9.31 |
46.83 ± 6.20 |
0.401 |
Carbohydrate (g d−1) |
227.82 ± 93.17 |
162.34 ± 25.80 |
293.29 ± 90.41 |
0.073 |
Dietary fiber intake (g d−1) |
24.24 ± 9.07 |
20.94 ± 8.30 |
27.54 ± 10.21 |
0.434 |
Fat intake (%) |
40.62 ± 6.07 |
40.30 ± 7.39 |
40.93 ± 6.11 |
0.914 |
Fat intake (g d−1) |
96.02 ± 40.69 |
77.65 ± 40.35 |
114.39 ± 38.73 |
0.319 |
Calcium intake (mg d−1) |
822.85 ± 530.98 |
773.65 ± 597.90 |
872.05 ± 583.19 |
0.848 |
Vitamin D intake (μg d−1) |
0.92 (0.61–5.05) |
1.09 (0.85–10.12) |
0.75 (0.44–2.90) |
0.513 |
|
Biochemical measurements
|
Ca serum (mg dL−1) |
9.40 ± 0.25 |
9.47 ± 0.32 |
9.33 ± 0.21 |
0.579 |
BALP serum (μg dL−1) |
15.10 ± 2.30 |
16.30 ± 1.05 |
13.87 ± 2.75 |
0.220 |
Osteocalcin serum (ng mL−1) |
29.20 ± 5.38 |
32.20 ± 5.90 |
26.20 ± 3.27 |
0.198 |
Vitamin D serum (ng mL−1) |
18.95 ± 6.06 |
18.53 ± 6.15 |
19.37 ± 8.42 |
0.897 |
3.2 Effect of mushroom fermentation on the microbiota composition
Overall, no significant differences were detected among different treatments in terms of tested bacteria based on the main effects (Table 2 and Tables S3a, S3b†) and post-hoc analysis, though a trend was detected in the case of bacteroides in all subjects (p = 0.059) (Table 2) and bifidobacteria in osteopenia (p = 0.096) (Table S3b†). Time had a significant effect in the case of lactobacilli (p < 0.001), Roseburia spp.–E. rectale (p < 0.001) and likely C. leptum group (p = 0.080), whereas a significant time × treatment interaction was detected in the case of Bifidobacterium spp. (p = 0.006) and likely Bacteroides spp. (p = 0.055), lactobacilli (p = 0.068) and Roseburia spp.–E. rectale (p = 0.099). In healthy subjects, a significant time effect was detected in the case of lactobacilli and Roseburia spp.–E. rectale, with a trend for time × treatment interaction in the case of lactobacilli (p = 0.093). In osteopenic subjects time had significant effects on bifidobacteria, lactobacilli, C. leptum group and C. perfringens (p = 0.052), with significant time × treatment interactions in the case of total bacteria, bifidobacteria and likely bacteroides (p = 0.099).
Table 2 Faecal microbial quantification (qPCR; log10 copies of 16S rRNA gene per mL of sample) at the baseline and after 24 h fermentation of Pleurotus ostreatus IK1123 and Ganoderma lucidum LGAM 9720 in all volunteers
|
TOTAL (n = 6) |
Baseline (t = 0 h) |
After fermentation (t = 24 h) |
NC |
INU1 |
INU2 |
POWS |
GLBS |
POWSE |
GLBSE |
NC |
INU1 |
INU2 |
POWS |
GLBS |
POWSE |
GLBSE |
P overall |
Values are expressed as mean value and SD for parametric and median and interquartile range (Q1–Q3) for non-parametric data; *: significantly different compared to [NC] at t = 24 h (p < 0.05); †: significantly different compared to [INU1] at t = 24 h (p < 0.05); ‡: significantly different compared to [INU2] at t = 24 h (p < 0.05); a: significantly different compared to the baseline (p < 0.05) (paired-sample t-test or Wilcoxon signed-rank test); p-overall refers to the tests of between-subjects effects and symbols *,†, and ‡ refer to parameter estimates at t = 0 h and 24 h (repeated-measures ANOVA or Friedman test; NC: Negative control; INU1: Inulin 1% (w/v); INU2: Inulin 2% (w/v); POWS: P. ostreatus untreated mushroom powder; POWSE: P. ostreatus mushroom-extract; GLBS: G. lucidum untreated mushroom powder; GLBSE: G. lucidum mushroom-extract. |
Total bacteria |
10.08 (9.86–10.35) |
10.10 (9.93–10.32) |
10.05 (9.92–10.33) |
9.97 (9.89–10.30) |
9.96 (9.89–10.25) |
10.11 (9.95–10.40) |
10.08 (9.82–10.33) |
10.00 (9.88–10.11) †, ‡ |
10.33 (10.06–10.54)* |
10.38 (10.11–10.47)* |
10.45 (10.23–10.49) *a |
10.13 (9.99–10.17) |
10.21 (10.07–10.35) |
10.16 (9.91–10.23) |
0.407 |
Bifidobacterium spp. |
8.69 (7.52–9.10) |
8.69 (7.52–9.16) |
8.64 (7.49–9.00) |
8.60 (7.51–9.07) |
8.65 (7.51–9.14) |
8.67 (7.52–9.17) |
8.68 (7.42–9.20) |
8.81 (7.75–9.15) †, ‡ |
9.75 (9.729.96)*a |
9.94 (9.82–10.21)*a |
9.75 (8.56–9.95)a |
9.25 (7.33–9.57)† |
9.20 (7.59–9.51)† |
9.33 (7.81–9.94) |
0.875 |
Lactobacillus group |
6.28 ± 0.97 |
6.27 ± 0.88 |
6.23 ± 0.96 |
6.20 ± 0.91 |
6.03 ± 0.90 |
6.36 ± 0.90 |
6.24 ± 0.94 |
6.34 ± 0.93 † |
7.35 ± 0.83a |
7.60 ± 1.04*a |
6.90 ± 1.19 |
6.16 ± 1.23‡ |
6.64 ± 1.00 |
7.07 ± 1.01 |
0.734 |
Clostridium perfrigens group |
5.90 (5.71–7.01) |
6.07 (5.77–7.03) |
6.04 (5.77–7.05) |
6.27 (6.05–7.01) |
5.42 (4.93–6.69) |
6.08 (5.85–7.09) |
6.21 (5.79–7.03) |
6.10 (5.73–6.94) |
6.03 (5.53–7.17) |
6.08 (5.64–7.35) |
6.47 (5.93–7.21) |
6.08 (5.31–6.90) |
6.51 (5.68–7.32) |
5.98 (5.41–7.28) |
0.810 |
Bacteroides spp. |
9.43 ± 0.23 |
9.43 ± 0.16 |
9.43 ± 0.25 |
9.45 ± 0.23 |
9.39 ± 0.23 |
9.49 ± 0.20 |
9.43 ± 0.24 |
9.21 ± 0.29 †, ‡ |
9.60 ± 0.27* |
9.63 ± 0.35* |
9.19 ± 0.30†, ‡ |
9.45 ± 0.27 |
9.75 ± 0.15*a |
9.44 ± 0.20 |
0.059 |
Clostridium leptum group |
9.20 ± 0.43 |
9.22 ± 0.38 |
9.20 ± 0.44 |
9.18 ± 0.39 |
9.14 ± 0.47 |
9.32 ± 0.40 |
9.27 ± 0.45 |
9.12 ± 0.43 |
9.03 ± 0.54 |
9.13 ± 0.53 |
9.31 ± 0.47 |
9.11 ± 0.44 |
9.22 ± 0.41 |
9.04 ± 0.39 |
0.995 |
Faecalibacterium prausnitzii
|
8.52 (7.66–9.03) |
8.56 (7.80–8.89) |
8.51 (7.84–9.00) |
8.61 (7.81–8.97) |
8.58 (7.61–8.95) |
8.60 (7.86–8.97) |
8.54 (7.78–8.98) |
8.12 (7.53–8.40) ‡ |
8.81 (7.99 8.87) |
8.86 (8.32–8.94)* |
8.92 (8.70–9.24)*a |
8.65 (7.67–8.73) |
8.80 (8.40–8.94) |
8.48 (7.80–8.74) |
0.763 |
Roseburia spp.–Eubacterium rectale |
8.39 ± 0.58 |
8.35 ± 0.58 |
8.39 ± 0.60 |
8.35 ± 0.51 |
8.43 ± 0.59 |
8.44 ± 0.54 |
8.4 ± 0.60 |
7.62 ± 0.42a |
8.11 ± 0.83 |
8.10 ± 0.78 |
8.02 ± 0.76 |
7.67 ± 0.44a |
7.79 ± 0.43a |
7.91 ± 0.69a |
0.989 |
POWS fermentation resulted in an increase of total bacteria, Bifidobacterium spp. and Faecalibacterium prausnitzii counts after 24 h of fermentation compared to the baseline (Table 2). Based on the parameter estimates (Table 2), the butyrate producer F. prausnitzii increased after POWS fermentation relatively to NC. In addition, the levels of Bacteroides spp. after 24 h fermentation of POWS and counts of Bifidobacterium spp. and Lactobacillus group after 24 h fermentation of GLBS were significantly lower than INU2 in all volunteers (Table 2). The positive controls INU1 and INU2 exhibited increased the counts of total bacteria, Lactobacillus group, Bifidobacterium spp. and Bacteroides spp. after 24 h of fermentation compared to the NC (Table 2).
In healthy subjects, 24 h fermentation of POWS increased the level of total bacteria compared to the NC (p = 0.012) and decreased the count of Bacteroides spp. compared to INU1 (p = 0.034) and INU2 (p = 0.034) (ESI, Table S3a†).
In the osteopenic group, POWS fermentation ended in higher levels of total bacteria, Bifidobacterium spp. (p = 0.004), and F. prausnitzii (p = 0.010) than in the NC group. F. prausnitzii counts were also increased after 24 h fermentation of POWS compared to INU1 (p = 0.014) and INU2 (p = 0.034). Moreover, the fermentation of POWSE demonstrated higher populations of total bacteria, F. prausnitzii and Bacteroides spp. than the NC.
Bacteroides spp. increased after 24 h of fermentation compared to the baseline in this substrate, while Roseburia spp. – E. rectale decreased. GLBS fermentation resulted in the lower levels of Bifidobacterium spp. (p = 0.025) than INU2. In addition, the use of GLBSE exhibited higher populations of Bifidobacterium spp. (p = 0.047) and Bacteroides spp. (p = 0.019) than the NC at 24 h. Roseburia spp. – E. rectale decreased after 24 h of fermentation compared to the baseline in both substrates (PGLBS = 0.005 and PGLBSE = 0.043) (ESI, Table S3b†). Our results were in agreement with those of previous studies regarding bacterial changes after fermentation of inulin.61
In vitro studies have proved the prebiotic potential of inulin-type fructans after fermentation by human cecal or faecal inoculum, leading to a significant increase of Bifidobacterium spp. and Lactobacillus spp.62 Nevertheless, several members of the Lachnospiraceae and Ruminococcaceae families in the phylum Firmicutes also increased after inulin fermentation.63 These two families include most of the known butyrate producers,64 such as Eubacterium rectale, Roseburia spp. and F. prausnitzii, all of which are capable of producing butyrate from inulin fermentation.63,65 In our study, both positive controls showed powerful bifidogenic and lactogenic effects, especially INU2, which was also accompanied by higher F. prausnitzii levels compared to the NC.
With respect to different mushrooms, our data support that POWS fermentation increases the bifidobacteria and F. prausnitzii levels, a phenomenon that is more evident in osteopenic women. Mitsou et al. have recently examined the in vitro fermentation of different edible mushrooms using the same methodology while they used different faecal donors (men and women over 65 years old); increase of the same microbial populations has been also noticed after the in vitro fermentation of two different strains of P. ostreatus.46 In another paper where the in vitro fermentation of edible mushrooms was also studied, the authors highlighted the different impacts of various mushroom strains on the gut microbial populations.66P. ostreatus and P. eryngii mushrooms promoted the growth of Bifidobacterium spp. compared to the control group, while they did not favor the growth of Lactobacillus spp., as it was also noticed in the present study. Despite the similar research approach adopted by both studies involving in vitro fermentation of Pleurotus mushroom samples, the type of participants (young healthy faecal donors) and the methodology used (16SrRNA sequencing for microbial analysis) may explain the differences observed in the growth of other microbial groups (i.e., F. prausnitzii).66 The POWSE treatment appears to significantly impact F. prausnitzii and Bacteroides spp., but only in the group of osteopenic women. According to these results, the P. ostreatus mushroom powder has a more beneficial effect on the gut microbial populations than the respective mushroom-extract. In another relevant study where polysaccharide extracts from P. ostreatus and P. eryngii mushrooms were studied for their impact on the gut microbiota in vitro, a significant increase of Lactobacillus and Bifidobacterium species as well as Enterococcus faecium was observed.42 Furthermore, the consumption of P. eryngii polysaccharide extracts by mice strongly influenced their gut microbiota and increased the abundance of Porphyromonadaceae, Rikenellaceae, Bacteroidaceae and Lactobacillaceae.67 Even though the methodological approach was different from that used in the present study, the results were rather similar since Bacteroides spp. are the main members of the family Bacteroidaceae in the gut.
GLBS fermentation had no significant effect on the initial levels of bifidobacteria and lactobacilli (Table 3). However, in the osteopenic group the fermentation of GLBSE (t = 24 h) exhibited significantly higher bifidobacterial counts than in the NC group. Based on the results concerning the effect of G. lucidum, the mushroom-extract exhibits a more favorable effect on the gut microbial populations than the mushroom powder. Our results were in accordance with the outcome of previous studies that reported the promising prebiotic activity of Ganoderma mushroom-extracts.35,68 Recently Khan et al. has observed high levels of beneficial bacteria (e.g. Bifidobacterium choerinum, Lactobacillus johnsonii, and Lactococcus lactis) in the mice group, which was fed with G. lucidum mushroom-extract, compared to the control group.69 Similar results in the growth of bacteria of the genera Bifidobacterium, Lactobacillus and Bacteroides were also reported after 24 h fermentation of GLBSE using faecal material from healthy donors.70 However, Chang et al., who tested water extracts from G. lucidum mycelia in mice, did not notice an increase in the Bifidobacterium spp. levels, but noticed an enhancement in the growth of Clostridium clusters IV, XVIII and XIVa.71
Table 3 Total volatile fatty acid (VFAs) concentrations (μmol mL−1 of sample) and molar ratios (%) of SCFAs at the baseline and after 8 h and 24 h of fermentation in all subjects
Total (n = 6) |
Baseline (t = 0 h) |
|
|
Molar ratios of SCFAs |
Molar ratios of branched-chain SCFAs (%)† |
Molar ratios of other SCFAs (%)‡ |
|
Total VFAs (μmol mL−1 of sample) |
Acetate (%) |
Propionate (%) |
Butyrate (%) |
Branched-chain SCFA (%)‡ |
Iso-butyrate (%) |
Iso-valerate (%) |
Iso-caproic acid (%) |
Other SCFA (%) ¥ |
Valerate (%) |
Caproic acid (%) |
Heptanoic acid (%) |
NC |
4.38 †, ‡ (3.96–4.34) |
34.83 (31.08–48.48) |
12.94 (10.75–14.16) |
30.03‡ (20.61–43.17) |
7.91 (5.60–12.18) |
2.04 (1.04–3.21) |
3.01 (2.08–6.08) |
2.85 (1.18–4.56) |
5.94 (9.55–12.13) |
4.02 (2.54–5.47) |
0.96 (0.42–2.78) |
0.87 (0.39–1.77) |
INU1 |
2.41* (1.89–2.71) |
37.44 (34.06–43.43) |
13.62 (11.91–17.05) |
31.29‡ (24.97–39.47) |
9.21 (5.71–9.96) |
1.95 (1.56–2.46) |
2.89 (1.94–3.60) |
3.67 (1.06–5.04) |
6.50 (4.26–9.63) |
3.89 (2.70–4.51) |
1.02 (0.83–2.99) |
0.80 (0.71–1.00) |
INU2 |
2.58* (2.21–3.14) |
31.30† (28.69–33.45) |
11.74 (9.05–15.46) |
45.34*,† (38.13–48.67) |
6.33 (5.15–7.71) |
1.50 (0.99–2.39) |
2.31 (1.88–3.43) |
2.47‡ (0.92–3.50) |
4.72 (4.44–7.74) |
3.04 (2.91–3.16) |
0.88 (0.78–2.28) |
0.72 (0.65–0.87) |
POWS |
2.93†(2.79–3.84) |
32.48 (30.78–34.53) |
8.36*,† (5.85–11.77) |
47.90*,† (46.50–52.88) |
5.25*,† (4.36–5.70) |
1.07*,† (0.90–1.45) |
2.62 (2.34–3.00) |
0.69 *,†,‡ (0.62–1.7) |
3.79 (3.55–5.35) |
2.42*,†,‡ (2.25–2.47) |
0.70 (0.62–1.62) |
0.58 (0.56–0.69) |
GLBS |
2.74 † (2.51–3.79) |
32.93 (27.40–36.00) |
9.84*,† (7.02–11.39) |
48.85*,†,‡ (45.17–56.76) |
4.60*,†,‡ (4.08–5.38) |
1.90 (1.21–2.14) |
2.10 (1.79–2.25) |
0.69*,†,‡ (0.61–0.89) |
3.64*,† (3.37–4.39) |
2.09*,†,‡ (1.69–2.28) |
0.73 (0.67–1.36) |
0.62 (0.60–0.69) |
POWSE |
2.64 (2.41–3.07) |
35.35 (30.75–37.30) |
10.64† (9.69–11.78) |
45.58*,† (40.76–48.99) |
4.68*,† (3.82–6.02) |
1.60 (1.09–1.99) |
2.51 (1.91–3.36) |
0.76*,†,‡ (0.57–0.82) |
4.56‡ (4.08–5.38) |
2.73 (2.50–3.28) |
0.81 (0.72–0.83) |
0.74 (0.66–0.75) |
GLBSE |
2.16* (2.12–2.68) |
35.95‡ (33.84–37.21) |
11.42 (9.52–13.85) |
44.08*,† (40.01–46.74) |
4.12*,†,‡ (3.54–6.25) |
1.52 (1.27–2.00) |
1.75 (1.36–3.52) |
0.84*,† (0.65–0.95) |
4.82 (4.62–5.76) |
3.08 (2.71–3.62) |
0.90 (0.81–0.98) |
0.76 (0.63–0.87) |
After fermentation (t = 8 h) |
|
|
Molar ratios of SCFAs |
Molar ratios of branched-chain SCFAs (%)† |
Molar ratios of other SCFAs (%)‡ |
|
Total VFAs (μmol mL−1 of sample) |
Acetate (%) |
Propionate (%) |
Butyrate (%) |
Branched-chain SCFA (%)‡ |
Iso-butyrate (%) |
Iso-valerate (%) |
Iso-caproic acid (%) |
Other SCFA (%) ¥ |
Valerate (%) |
Caproic acid (%) |
Heptanoic acid (%) |
NC |
16.59†,‡a (11.34–17.32) |
47.65 (41.65–48.52) |
16.00 (15.18–21.52)a |
25.90 (24.40–27.42) |
5.60†, ‡ (4.28–9.36) |
1.47 (1.10–2.64) |
4.12†, ‡ (2.98–5.91) |
0.15†,‡a (0.13–0.26) |
5.94 †, ‡ (3.48–9.33) |
3.80†, ‡ (2.43–4.06) |
0.38† (0.12–1.00) |
0.14†,‡a (0.11–0.22) |
INU1 |
38.11*a (30.13–44.58) |
50.94a (46.59–54.68) |
14.47 (10.54–30.14) |
33.23 (11.79–33.51) |
1.46*a (0.77–2.10) |
0.94a (0.39–1.65) |
0.39*a (0.37–0.43) |
0.05*a (0.04–0.06) |
0.86*a (0.75–1.01) |
0.72*a (0.51–0.85) |
0.05*a (0.04–0.23) |
0.04*a (0.04–0.06) |
INU2 |
37.28*a (25.43–43.55) |
50.09a (44.37–69.40) |
14.3 (11.01–16.29) |
23.71a (17.55–36.65) |
1.67*a (1.15–2.26) |
1.19 (0.81–1.90) |
0.34*,‡a (0.29–0.41) |
0.05*,‡a (0.05–0.07) |
0.77*a (0.68–0.85) |
0.61*a (0.43–0.76) |
0.06a (0.05–0.23) |
0.05*a (0.04–0.06) |
POWS |
47.80*a (35.11–55.79) |
41.72a (39.45–58.56) |
18.07a (14.90–28.72) |
27.67a (15.44–41.97) |
1.57*a (1.04–2.73) |
1.07 (0.57–2.12) |
0.34*a (0.33–0.49) |
0.05*a (0.04–0.19) |
0.64*a (0.49–0.99) |
0.55*a (0.35–0.91) |
0.04*a (0.03–0.05) |
0.04*a (0.03–0.05) |
GLBS |
30.20*a (26.21–39.32) |
42.55†a (41.20–46.19) |
23.08‡a (19.56–25.58) |
27.18a (22.66–32.44) |
2.85†,‡ (2.26–5.19) |
1.17 (0.75–1.63) |
2.09†, ‡ (0.86–3.48) |
0.06a (0.05–0.07) |
2.46†, ‡ (1.50–3.22) |
2.24†, ‡ (1.31–3.07) |
0.11a (0.05–0.31) |
0.05*a (0.05–0.06) |
POWSE |
42.66*a (33.91–48.68) |
46.48a (38.71–49.42) |
22.17‡a (19.69–33.78) |
25.29a (23.40–27.90) |
2.08*,† (1.71–2.90) |
1.17 (0.83–1.60) |
0.82†,‡ (0.66–1.13) |
0.07*a (0.04–0.17) |
1.65*,†,‡a (0.96–2.08) |
1.48*,‡a (0.80–1.99) |
0.05*a (0.04–0.23) |
0.04*a (0.03–0.05) |
GLBSE |
39.93*a (30.44–42.28) |
48.32a (46.86–50.24) |
20.48a (15.34–23.16) |
26.23a (17.00–37.19) |
1.59*a (1.25–2.84) |
0.93 (0.57–1.25) |
0.50*,†a (0.42–0.68) |
0.15†a (0.05–0.03) |
1.09*a (0.73–2.00) |
0.98*a (0.58–1.90) |
0.05a (0.05–0.06) |
0.05*a (0.04–0.06) |
After fermentation (t = 24 h) |
|
|
Molar ratios of SCFAs |
Molar ratios of branched-chain SCFAs (%)† |
Molar ratios of other SCFAs (%)‡ |
Values are expressed as median and interquartile range (Q1–Q3) for non-parametric data; ‡ Sum of iso-butyrate, iso-valerate and iso-caproic acid; ¥ Sum of valerate, caproic acid and heptanoic acid; *: significantly different compared to [NC] at t = 0 h, 8 h or 24 h (p < 0.05); †: significantly different compared to [INU1] at t = 0 h, 8 h or 24 h (p < 0.05); ‡: significantly different compared to [INU2] at t = 0 h, 8 h or 24 h (p < 0.05); a: significantly different from the baseline compared to after 8 h of fermentation (p < 0.05) (paired sample t-test or Wilcoxon for non-parametric); b: significantly different from after 8 h of fermentation compared to after 24 h of fermentation (p < 0.05) (paired sample t-test or Wilcoxon for non-parametric); c: significantly different from the baseline compared to after 24 h of fermentation (p < 0.05) (paired sample t-test or Wilcoxon for non-parametric); p-overall refers to the tests of between-subjects effects and symbols *,†, and ‡ refer to parameter estimates at t = 0 h, 8 h and 24 h (repeated-measures ANOVA or the Friedman test); NC: Negative control; INU1: Inulin 1% (w/v); INU2: Inulin 2% (w/v); POWS: P. ostreatus untreated mushroom powder; POWSE: P. ostreatus mushroom-extract; GLBS: G. lucidum untreated mushroom powder; GLBSE: G. lucidum mushroom-extract. |
|
Total VFAs (μmol mL−1 of sample) |
Acetate (%) |
Propionate (%) |
Butyrate (%) |
Branched-chain SCFA (%)‡ |
Iso-butyrate (%) |
Iso-valerate (%) |
Iso-caproic acid (%) |
Other SCFA (%) ¥ |
Valerate (%) |
Caproic acid (%) |
Heptanoic acid (%) |
NC |
23.68†,‡b,c (19.52–26.24) |
44.52‡ (38.89–47.41) |
14.85 (12.64–18.65) |
25.35 (22.19–26.25) |
10.09 †,‡b (9.55–10.13) |
3.44 †,‡b (3.41–3.52) |
6.24†,‡b,c (6.15–6.57) |
0.45 (0.07–0.76) |
4.70†,‡ (2.73–8.99) |
3.76†,‡ (3.13–4.18) |
0.87†, ‡ (0.39–1.77) |
0.24 †, ‡, c (0.07–0.71) |
INU1 |
43.11*b,c (38.38–61.90) |
50.12 (46.60–60.75) |
16.00 (10.93–19.12) |
28.23 (10.53–37.19) |
1.48*c (1.21–2.05) |
0.93*c (0.44–1.46) |
0.44*c (0.38–0.50) |
0.21c (0.03–0.32) |
0.75*c (0.61–0.88) |
0.63*c (0.52–0.71) |
0.04*c (0.03–0.06) |
0.04*c (0.03–0.06) |
INU2 |
36.88*c (31.58–61.07) |
57.04*c (47.10–69.42) |
13.28 (10.69–18.59) |
20.39b,c (12.55–33.02) |
1.00*b,c (0.80–1.56) |
0.57*b,c (0.26–1.18) |
0.31*,†,‡c (0.20–0.37) |
0.16c (0.04–0.38) |
4.72*c (4.44–7.74) |
0.53*,‡c (0.37–0.64) |
0.05*c (0.04–0.06) |
0.05*c (0.03–0.06) |
POWS |
76.06*,†,‡b,c (69.36–81.49) |
37.28*,†,‡c (35.91–38.61) |
15.55c (14.81–19.45) |
43.47*,†,‡b,c (40.02–46.55) |
1.15*b,c (0.79–1.23) |
0.78*b (0.29–1.24) |
0.38*c (0.20–0.51) |
0.11 *c (0.03–0.11) |
0.52*c (0.41–1.00) |
0.43*c (0.32–0.95) |
0.03*c (0.03–0.11) |
0.02*,†,‡b,c (0.02–0.02) |
GLBS |
49.61*b,c (44.25–54.03) |
39.64†,‡c (36.14–42.54) |
20.50‡c (18.71–22.18) |
27.52c (25.32–29.17) |
7.91†, ‡b,c (6.91–8.19) |
2.47‡b (1.85–3.09) |
4.98*,†,‡b,c (4.76–5.33) |
0.17c (0.04–0.22) |
4.14†,‡,b (2.74–6.28) |
3.21†,‡c (2.67–4.33) |
0.78 (0.04–1.53) |
0.16c (0.03–0.42) |
POWSE |
60.27*,†,‡b,c (57.11–62.20) |
39.27†,‡,b (34.14–42.84) |
24.46*,†,‡c (22.41–28.46) |
30.31b,c (28.08–33.26) |
3.10*,†,‡c (2.24–3.83) |
0.98*,† (0.70–1.58) |
1.66*,†,‡b (1.13–2.22) |
0.24c (0.06–0.39) |
2.17†,‡c (1.58–3.10) |
2.01†,‡c (1.52–2.65) |
0.11c (0.04–0.21) |
0.05*c (0.03–0.11) |
GLBSE |
47.06*b,c (45.46–48.21) |
44.1 (41.60–48.71) |
18.44c (14.23–21.09) |
29.77b,c (19.34–39.36) |
2.40*,‡ (1.03–4.57) |
1.51* (0.42–2.30) |
0.74*,‡ (0.38–1.17) |
0.19c (0.05–0.27) |
1.15*,†,‡ (0.75–2.06) |
0.96*,† (0.68–1.97) |
0.05 (0.05–0.23) |
0.04*c (0.04–0.06) |
P overall |
<0.001 |
0.008 |
0.525 |
0.054 |
<0.001 |
0.016 |
<0.001 |
0.002 |
<0.001 |
<0.001 |
0.066 |
<0.001 |
3.3 Prebiotic effect of the mushroom substrates after 24 h of fermentation
The prebiotic indexes of INU1, INU2, POWS and GLBSE increased significantly in all volunteers after 24 h of fermentation compared to the NC (Fig. 1). Furthermore, no significant differences were detected in the PI values of GLBSE with respect to the positive controls (INU1 and INU2) (Fig. 1). POWS and GLBSE were characterized by positive prebiotic indexes in both groups of bone health status, while GLBSE demonstrated the highest PI values in both total and bone health groups (Fig. 1 and ESI, Fig. S2A, B†). When these results are associated with bacterial compositional analysis, our data suggest that the high counts of Bifidobacterium after POWS and GLBSE treatments clearly demonstrated an advantageous prebiotic effect, especially in the osteopenic group.
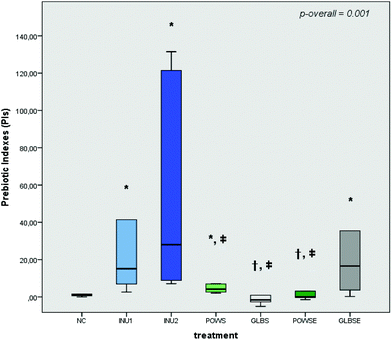 |
| Fig. 1 Prebiotic indexes (PIs) for all volunteers. NC: Negative control; INU1: Inulin 1% (w/v); INU2: Inulin 2% (w/v); POWS: P. ostreatus untreated mushroom powder; POWSE: P. ostreatus mushroom-extract; GLBS: G. lucidum untreated mushroom powder; GLBSE: G. lucidum mushroom-extract; *: significantly different compared to [NC] (p < 0.05) (Mann–Whitney test); †: significantly different compared to [INU1] (p < 0.05) (Mann–Whitney test); ‡: significantly different compared to [INU2] (p < 0.05) (Mann–Whitney test); data are presented as boxplots, with the horizontal line representing the median and the whiskers the minimum and maximum values. | |
3.4 Impact of mushroom substrates on faecal SCFAs
Although at the baseline (0 h) the total concentration of SCFAs was quite low in all cases examined (approximately 2–4 μmol mL−1 culture), after 8 h and particularly after 24 h of fermentation all substrates were characterised by higher SCFA concentration changes (p < 0.05) compared to the negative control.
Overall, the treatments resulted in significant differences in the total concentration and molar ratios of SCFAs in all subjects, except in the case of propionate (Table 3). Nevertheless, overall analysis in the osteopenic group indicated differences among treatments in cases of propionate (p = 0.078), butyrate (p = 0.083) and iso-butyrate (p = 0.015), a fact not evident in healthy subjects (Tables S4a and S4b†). Time had significant effects on the osteopenic group (priopionate, valerate, and BSCFAs) and on healthy subjects (iso-caproic acid). Furthermore, significant time × treatment interactions were detected in total SCFAs (all subjects and healthy group), propionate (all subjects), butyrate (all subjects and osteopenic group), iso-butyrate (all subjects), iso-valerate (all subjects and healthy group), iso-caproic acid (all subjects), BSCFAs (all subjects and osteopenic group), valerate (all subjects, and healthy and osteopenic groups) and other SCFAs (all subjects and healthy group) (p<0.05 for all).
Post hoc analysis indicated higher SCFA concentration after POWS treatment compared to the NC in all cases and when compared to the rest of the treatments (except POWSE) in all subjects and the healthy group. The POWSE substrate also demonstrated higher levels of SCFAs than the NC in all subjects and the osteopenic group. POWS and GLBS had a lower molar ratio of acetate than INU1, whereas POWS had a higher molar ratio of butyrate than the NC in all subjects. In general, the NC has a higher molar ratio of BSCFAs than INU1, INU2, POWS, POWSE (all subjects and osteopenic group) and GLBSE (all subjects and subgroups of bone health). Analysis of individual BSCFA indicated that POWS exhibited lower molar ratios of iso-butyrate (all subjects and osteopenic group), isovalerate (all subjects and subgroups of bone health) and isocaproic acid (all subjects) than the NC. Higher molar ratios of other SCFAs were detected after NC treatment compared to INU1, INU2, POWS, GLBSE (all subjects and healthy group) and INU2, POWS (osteopenic group). POWS had lower molar ratios of other SCFAs and valerate than the NC, POWSE, GLBS and GLBSE in all subjects and the healthy group. Furthermore, the POWS treatment induced a lower molar ratio of caproic acid than the NC in all subjects and lower levels of heptanoic acid than the NC and the rest of the treatments in all subjects (p <0.05 for all).
Based on parameter estimates the POWS and POWSE substrates demonstrated the highest 24 h SCFA concentrations, which were significantly higher than those of the negative and positive controls in all volunteers (Table 3).
Our results are in concordance with those of Mitsou et al., who reported that 24 h fermentation of P. ostreatus untreated mushroom powder exhibited significantly higher SCFA concentration levels compared than the negative control and inulin (2% w/v) in elderly healthy subjects.46 In the case of G. lucidum substrates, total SCFAs after 24 h of fermentation were similar to those of the positive controls and higher than the NC values (Table 3 and Fig. 2a). Similar results were obtained in both bone health groups (ESI, Tables S4a and S4b†). The main sources of SCFAs are dietary carbohydrates fermented by gut bacteria and due to the high content of glucans in mushrooms17 the total SCFA concentration was enhanced in all mushroom-based substrates in the present study. Primary bacterial degraders depolymerize specific polysaccharides to mono-, di-, and oligosaccharides that can be fermented to acidic end products such as acetate or lactate; such intermediate fermentation products are metabolized by secondary fermenters such as F. prausnitzii, Eubacterium rectale and Roseburia spp. into new molecules, including butyrate.62 In our study, both positive controls exhibited the highest molar ratio of acetate as expected, since they also showed increased levels of Bifidobacterium and Lactobacillus spp. It has been well established by studies performed in humans and in rodents,72,73 that inulin and oligofructose stimulate the growth of bifidobacteria, which lead to acetic and lactic acid production.61 Mushroom-based substrates significantly increased the acetate concentration after 24 h of fermentation, which is particularly notable in comparisons versus NC (Fig. 2b).
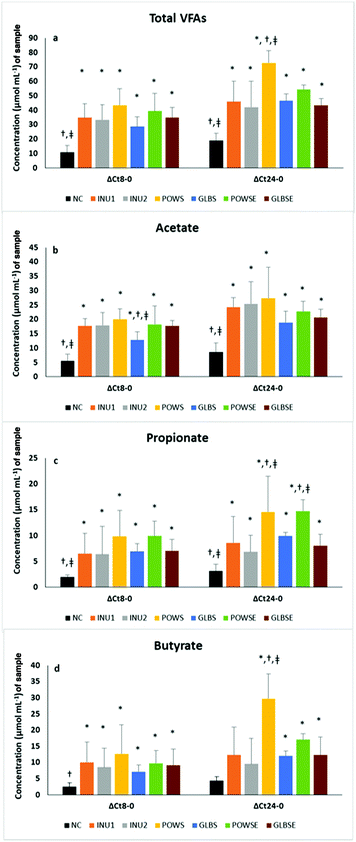 |
| Fig. 2 Differences (Δ) in the concentrations (μmol mL−1) of (a) total volatile fatty acids (VFAs), (b) acetate, (c) propionate and (d) butyrate after 8 h of fermentation (ΔCt8-0) and 24 h of fermentation (ΔCt24-0) compared to the baseline; values are expressed as mean and SD, where ΔCt8-0 is defined as ‘concentration t = 8 h minus concentration t = 0 h’ and ΔCt24-0 is defined as ‘concentration t = 24 h minus concentration t = 0 h’; *: significantly different compared to [NC] (p < 0.05) (Mann–Whitney test or t-test); †: significantly different compared to [INU1] (p < 0.05) (Mann–Whitney test or t-test); ‡: significantly different compared to [INU2] (p < 0.05) (Mann–Whitney test or t-test); NC: Negative control; INU1: Inulin 1% (w/v); INU2: Inulin 2% (w/v); POWS: P. ostreatus untreated mushroom powder; POWSE: P. ostreatus mushroom-extract; GLBS: G. lucidum untreated mushroom powder; GLBSE: G. lucidum mushroom-extract. | |
Similar results have been recently reported after 24 h in vitro fermentation of POWS; the mushroom-based substrate substantially increased the acetate concentration compared to the NC and INU2, whereas its molar ratio was significantly lower than both negative and positive controls.46
Propionate and butyrate are produced by distinct subsets of the gut bacteria.74,75 Butyrate is well known as the main energy source for intestinal epithelial cells and both of these preceding SCFAs have been associated with many beneficial effects on host health by activating not fully recognized signaling pathways,76,77 those associated with anti-lipogenic, anti-inflammatory and anti-carcinogenic activities74,78,79 or the enhancement of epithelial barrier integrity.80
SCFAs have been suggested to exert inhibitory effects on bone resorption and inflammation, especially by suppressing osteoclast formation, and to promote osteoblast differentiation by enhancing the production of bone sialoprotein and osteopontin in degenerative bone diseases such as osteoporosis.81–83
Regarding propionate, it reached the highest ratios after 8 h of fermentation in all treatments (POWS, POWSE, GLBS, and GLBSE) compared to the baseline, and remained stable after 24 h in all volunteers. In addition, fermentation of POWS and POWSE for 24 h resulted in the highest concentrations of propionate compared to the NC, INU1 and INU2 (Fig. 2c). Furthermore, a significant increase in the molar ratio of propionate was detected especially for POWSE and GLBS compared to the baseline and positive controls in all volunteers (Table 3).
In the healthy group, POWSE fermentation for 24 h resulted also in a significantly higher molar ratio compared to the baseline. In the osteopenic group, the molar ratio of propionate was higher compared to the baseline for POWS, POWSE and GLBS, while for POWSE it was also higher than the negative and positive controls (ESI, Tables S4a and S4b†). In our study, the POWSE treatment induced a significant increase of Bacteroides spp., which is probably associated with the propionate outcome. Previous studies have reported that Bacteroidetes were able to produce several SCFAs, such as acetic and propionic acids based on a broad range of glycoside hydrolases and the carbohydrate metabolic pathways.4 Furthermore, Bacteroidetes are considered as the major producers of propionate from dietary carbohydrates.17
With respect to GLBSE, our results were in agreement with those of Ding et al., who reported that in vivo and in vitro fermentation of Ganoderma atrum polysaccharides resulted in increased concentrations of total SCFAs, and acetic and propionic acids.84 Butyrate concentrations were found to be high especially in the case of POWS after 24 h of fermentation compared to both negative and positive controls (Fig. 2d); similar results were obtained when the molar ratio was measured in all volunteers (Table 3), particularly in the osteopenic group (ESI, Table S4b†). This fact could be partially explained by the increased presence of F. prausnitzii after fermentation of the POWS substrate. Moreover, the butyrate molar ratio increased significantly after 24 h of POWSE and GLBSE fermentation (compared to 8 h) in all volunteers. Our results were in agreement with those of Mitsou et al., demonstrating the same effect after 24 h fermentation of POWS on butyrate and propionate concentrations and molar ratios when compared to NC.46
It is well established that the degradation of branched-chain amino acids (valine, leucine, and isoleucine) leads to the production of isobutyrate, isovalerate and 2-methyl butyrate acid, respectively, which are known as branched-chain SCFAs (BCSCFAs).85,86 The production of BCSCFAs after 24 h fermentation of NC was significantly increased when compared to 8 h fermentation, which is probably due to the proteolytic activity of the faecal microbiota, a phenomenon that was reflected also in the iso-butyrate and iso-valerate molar ratios (Fig. 3b and Table 3).
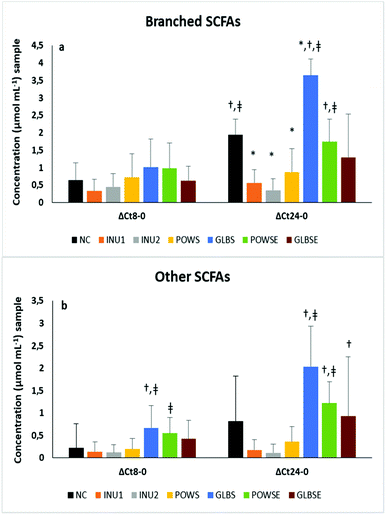 |
| Fig. 3 Differences (Δ) in the concentrations (μmol mL−1) of (a) branched SCFAs (BCSFAs) and (b) other SCFAs after 8 h of fermentation (ΔCt8-0) and 24 h of fermentation (ΔCt24-0) compared to the baseline; values are expressed as mean and SD, where ΔCt8-0 is defined as ‘concentration t = 8 h minus concentration t = 0 h’ and ΔCt24-0 is defined as ‘concentration t = 24 h minus concentration t = 0 h’; *: significantly different compared to [NC] (p < 0.05) (Mann–Whitney test or t-test); †: significantly different compared to [INU1] (p < 0.05) (Mann–Whitney test or t-test); ‡: significantly different compared to [INU2] (p < 0.05) (Mann–Whitney test or t-test); NC: Negative control; INU1: Inulin 1% (w/v); INU2: Inulin 2% (w/v); POWS: P. ostreatus untreated mushroom powder; POWSE: P. ostreatus mushroom-extract; GLBS: G. lucidum untreated mushroom powder; GLBSE: G. lucidum mushroom-extract. | |
The concentration levels of BCSCFAs and other SCFAs were significantly higher after 24 h fermentation of POWSE and GLBS compared to the positive controls (Fig. 3a and b). The molar ratio of total BCSCFAs diminished substantially in all substrates (except the GLBS) after 24 h of fermentation compared to the negative control in all volunteers.
Indeed, the molar ratio of total BCSCFAs for GLBS increased compared to the baseline and 8 h of fermentation. The same pattern was observed for iso-butyrate and iso-valerate (Table 3). Analysis by bone health status also indicated an increase in the molar ratios of BCSCFAs after 24 h fermentation of NC and GLBS in both groups (ESI, Tables S4a and S4b†).
Although both mushrooms exhibited a relatively high protein content,38,87,88 enhanced molar ratios of BCSCFAs were noticed only after fermentation of the substrates based on G. lucidum.
This result could imply different structures of mushroom macronutrients and/or the presence of complex compounds affecting protein fermentation. In general, the end-products of protein fermentation in the colon (e.g. ammonia, H2S and phenols) are associated with important injuries of the epithelium which may lead to inflammatory bowel diseases and colon cancer;86 however, the role of BCSCFAs is still poorly known. Otherwise, BCSCFAs such as isobutyric and isovaleric acid have been proposed to interfere with adipocyte lipid and glucose metabolism in vitro and may contribute to improved insulin sensitivity in metabolically burdened individuals.85
3.5 Cytotoxicity of fecal inocula on MG-63 cell line
As revealed by the results of the present study, individual's intestinal microbiota has a distinct impact on the viability of osteoblast cells (Fig. 4A). Despite the fact that the viability of MG-63 cells was significantly affected by interindividual variability of the fermentation procedure with different faecal inocula (p = 0.023) (Fig. 4A), this discrepancy was not verified by the group-specific analysis (p = 0.247) (Fig. 4B).
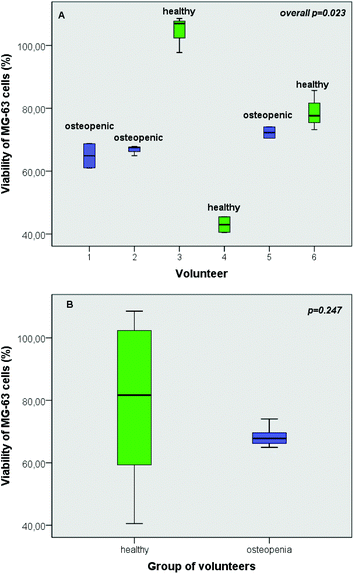 |
| Fig. 4 (A and B) Viability (%) of MG-63 cells in negative control treatments after 24 h of fermentation with faecal inocula from six volunteers (A) and by grouping volunteers according to their bone health (B); data are presented as boxplots, with the horizontal line representing the median and the whiskers the minimum and maximum values. | |
3.6 Impact of mushroom treatments on OPG and RANKL
The osteoblastic activity of OPG was induced in similar levels by the inulin (INU2) fermentation supernatant and the culture control treatment, both in all- and group-specific analysis (Fig. 5A–C). Similar results were also reported in the case of the osteoclastic effect of RANKL (Fig. 5D–F) in all groups (p for all >0.05). The POWSE treatment induced a drastic decrease in OPG levels compared to both the control (ptotal = 0.002, phealthy < 0.001, posteopenic < 0.001) and INU2 (ptotal < 0.001, phealthy < 0.001, posteopenic = 0.001) in all groups (Fig. 5A–C). G. lucidum based treatments also caused a significant reduction in OPG levels in all volunteers and according to the bone health status compared to INU2 (pGLBS-total < 0.001, pGLBS-healthy < 0.001, pGLBS-osteopenic = 0.001, pGLBSE-total < 0.001, pGLBSE-healthy = 0.005, pGLBSE-osteopenic = 0.026) and the culture control (pGLBS-total < 0.001, pGLBS-healthy < 0.001, pGLBS-osteopenic < 0.001, pGLBSE-total = 0.007, pGLBSE-healthy = 0.013, pGLBSE-osteopenic = 0.017) (Fig. 5A–C).
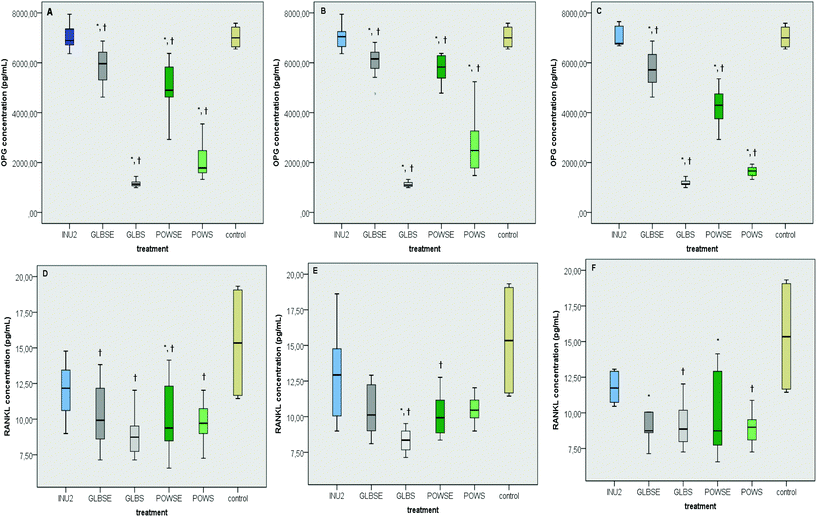 |
| Fig. 5 (A–F) Bone metabolism parameters of MG-63 cell cultures [OPG (A, B and C) and RANKL (D, E and F)] after treatment with 24 h fermentation supernatants from all subjects (A and D), healthy subjects (B and E) or subjects with osteopenia (C and F); *: significantly different compared to the control (p < 0.05); †: significantly different compared to INU2 (p < 0.05); INU2: Inulin 2% (w/v); POWS: P. ostreatus untreated mushroom powder; POWSE: P. ostreatus mushroom-extract; GLBS: G. lucidum untreated mushroom powder; GLBSE: G. lucidum mushroom-extract; control: MG-63 culture supernatant. Boxplots show OPG or RANKL concentration; data are presented as boxplots, with the horizontal line representing the median and the whiskers the minimum and maximum values. | |
Moreover, the POWS treatment resulted in lower RANKL levels than INU2 in all volunteers and osteopenic women. The hot-water extract of the same mushroom (POWSE) showed lower levels of RANKL than INU2 (p = 0.013) or the control (p < 0.001) in all volunteers and different subgroups (Fig. 5D–F). The RANKL levels after the GLBS treatment decreased when compared to INU2 in all groups, and the same effect was observed when GLBS was compared to the control in the healthy group only (p = 0.045) (Fig. 5E). In addition, GLBSE resulted in significantly lower RANKL levels than the control in the osteopenic group (p = 0.030) and INU2 in all volunteers (p = 0.011). Bone health specific analysis revealed also a lower RANKL concentration in GLBSE than in INU2 for both healthy (p = 0.098) and osteopenic (p = 0.076) women (Fig. 5D–F). In our study, INU2 induced no significant changes in parameters related to bone metabolism, such as OPG and RANKL levels. The dynamic osteoblastic potential of POWS could be attributed to the effect of butyrate and at a lesser extent to the effect of propionate.
Previous studies have highlighted the anti-resorptive properties of butyrate and propionate. Hence, Lucas et al. have recently proposed that propionate or butyrate increased the bone volume of healthy mice following diets rich in fermentable, indigestible fibers; no consistent effects of SCFA were reported on osteoblasts and bone formation, whereas propionate and butyrate strongly suppressed osteoclast differentiation.82
Previous in vitro studies based on administration of butyrate as a histone deacetylase inhibitor of bone cell metabolism reported suppression of osteoclastic activity, with incoherent effects on osteoblastic activity.71,89–91 In contrast to pre-existing evidence, Chang et al. supported that butyrate can stimulate RANKL, but decreases OPG expression and secretion from osteoblasts, within 24 h of exposure; however, exposure to lower concentrations of butyrate (<8 mM) for 72 h was demonstrated to stimulate OPG secretion.92 Due to the wide heterogeneity of results, caused by methodological discrepancies (e.g. different cell types, differentiation stage, butyrate concentration, and exposure time), no concluding statements could be made on the role of butyrate in bone metabolism.
The beneficial role of G. lucidum in RANKL inhibition could be attributed to its beta-glucan content and other equally bioactive compounds, such as triterpenoids. Among triterpenoids isolated from G. lucidum, ganoderic acid DM and its structurally related ganoderic acid F show inhibitory activity against osteoclastic differentiation.93
Miyamoto et al. proposed that the ethanol extracts of G. lucidum exhibit a bone-protective effect in ovariectomized rats, without substantially affecting the uterus. Ganoderic acid DM in particular suppressed the expression of c-Fos and NFATc1, which consequently regulated DC-STAMP expression and reduced osteoclast fusion.94 In addition, Tran et al. reported that Ganomycin I, which is a meroterpenoid compound isolated from G. lucidum, withheld RANKL-induced osteoclast differentiation, actin-ring formation, and resorption pit formation, while it also repressed the activation of MAPKs and their downstream transcription factor c-Fos expression.95
4. Conclusions
Recent data demonstrated that gut microbiota manipulation could be a promising strategy in the prevention or/and adjuvant treatment of chronic metabolic diseases, including bone metabolism disorders. Edible mushrooms are inexpensive, safe food choices and their potential positive effects on bone physiology have been currently highlighted, offering new alternatives in pharmacotherapy choices. The present work aimed to investigate two mushroom species, namely the ‘reishi mushroom’ (G. lucidum) and the ‘oyster mushroom’ (P. ostreatus), as potential novel prebiotics with possible beneficial effects on bone metabolism. In the present work an in vitro fermentation study was performed by using fresh faecal inocula from healthy and osteopenic women and different lyophilized mushrooms (rich in beta-glucans) as substrates. Instead of focusing only on a single category of potentially bioactive substances (e.g. beta-glucans), mushrooms were treated as suitable food in order to explore the possible synergistic effects of their constituents. In addition and in accordance with our experimental design, we hypothesized that the fermentation supernatant contains the microbial metabolic products that can be transferred from the gut environment to exert their biological action. Therefore, we used the fermentation products, which resulted from different gut microbiota inocula, to study their impact on the osteoblasts; in the past, most of the in vitro studies used single isolated products (e.g. SCFAs).71,89
Our data suggested that high counts of Bifidobacterium spp., after fermentation of POWS and GLBSE, demonstrated an advantageous prebiotic effect especially in the osteopenic group. Furthermore, fermentation of POWS significantly enhanced the growth of F. prausnitzii and was accompanied by a substantial increase in butyrate production. Our results also indicated that the gut microbiota of each volunteer influences in a unique way the viability of osteoblastic cells. The fermentation products of mushrooms and extracts induced a drastic decrease in OPG and RANKL levels, compared to inulin, in all volunteers. In subjects with osteopenia, fermentation of GLBSE and POWSE resulted in lower RANKL levels than the control. Hence, human studies of larger scale are necessary to elucidate the effects of edible mushrooms on bone health (based on the current human skeletal status) and bone turnover mechanisms in order to optimize osteopenia/osteoporosis prevention strategies, generate effective interventions for individuals belonging to high-risk groups and offer novel targeted therapeutics.
Conflicts of interest
The authors have no conflicts of interest to declare.
Acknowledgements
We warmly acknowledge the study participants for their contribution. We would also like to thank Dr Martsoukou Maria for kindly providing help in subject recruitment from the Department of Microbiology, “Sismanoglio” General Hospital, Attica and Mrs Tsiafitsa Antigoni for her excellent technical assistance in blood sampling and DXA scan measurements.
References
- I. Sekirov, S. L. Russel, L. C. M. Antunes and B. B. Finlay, Gut Microbiota in Health and Disease, Physiol. Rev., 2010, 90, 859–904 CrossRef CAS.
- A. T. Vieira, P. M. Castelo, D. A. Ribeiro and C. M. Ferreira, Influence of Oral and Gut Microbiota in the Health of Menopausal Women, Front. Microbiol., 2017, 8, 1884–1890 CrossRef.
- P. B. Eckburg, E. M. Bik, C. N. Bernstein, E. Purdom, L. Dethlefsen, M. Sargent, S. R. Gill, K. E. Nelson and D. A. Relman, Diversity of the Human Intestinal Microbial Flora, Science, 2005, 308, 1635–1638 CrossRef.
- P. J. Turnbaugh, R. E. Ley, M. A. Mahowald, V. Magrini, E. R. Mardis and J. I. Gordon, An obesity-associated gut microbiome with increased capacity for energy harvest, Nature, 2006, 444, 1027–1031 CrossRef.
- A. D. Kostic, R. J. Xavier and D. Gevers, The Microbiome in Inflammatory Bowel Disease: Current Status and the Future Ahead, Gastroenterology, 2014, 146, 1489–1499 CrossRef CAS.
- P. P. Chong, V. K. Chin, C. Y. Looi, W. F. Wong, P. Madhavan and V. C. Yong, The Microbiome and Irritable Bowel Syndrome – A Review on the Pathophysiology, Current Research and Future Therapy, Front. Microbiol., 2019, 10, 1136–1158 CrossRef.
- E. Untersmayr, H. J. Bax, C. Bergmann, R. Bianchini, W. Cozen, H. J. Gould, K. Hartmann, D. H. Josephs, F. Levi-Schaffer, M. L. Penichet, L. O'Mahony, A. Poli, F. A. Redegeld, F. Roth-Walter, M. C. Turner, L. Vangelista, S. N. Karagiannis and E. Jensen-Jarolim, AllergoOncology: Microbiota in allergy and cancer—A European Academy for Allergy and Clinical Immunology position paper, Allergy, 2019, 74, 1037–1051 CrossRef.
- M. C. Opazo, E. M. Ortega-Rocha, I. Coronado-Arrázola, L. C. Bonifaz, H. Boudin, M. Neunlist, S. M. Bueno, A. M. Kallergis and C. A. Riedel, Intestinal Microbiota Influences Non-intestinal Related Autoimmune Diseases, Front. Microbiol., 2018, 9, 432–451 CrossRef.
- W. W. Tang, T. Kitai and S. L. Hazen, Gut Microbiota in Cardiovascular Health and Disease, Circ. Res., 2017, 120, 1183–1196 CrossRef CAS.
- C. R. Martin, V. Osadchiy, A. Kalani and E. A. Mayer, The Brain-Gut-Microbiome Axis, Cell. Mol. Gastroenterol. Hepatol., 2018, 6, 133–148 CrossRef.
- N. E. Lane, Epidemiology, etiology, and diagnosis of osteoporosis, Am. J. Obstet. Gynecol., 2006, 194, 3–11 CrossRef.
- C. R. Villa, W. E. Ward and E. M. Comelli, Gut microbiota-bone axis, Crit. Rev. Food Sci. Nutr., 2015, 57, 664–1672 Search PubMed.
- C. Li, Q. Huang, R. Yang, Y. Dai, Y. Zeng, L. Tao, X. Li, J. Zeng and Q. Wang, Gut microbiota composition and bone mineral loss—epidemiologic evidence from individuals in Wuhan, China, Osteoporosis Int., 2019, 30, 1003–1013 CrossRef CAS.
- R. M. Jones, J. G. Mulle and R. Pacifici, Osteomicrobiology: The influence of gut microbiota on bone in health and disease, Bone, 2017, 115, 59–67 CrossRef.
- R. Pacifici, Bone Remodeling and the Microbiome, Cold Spring Harbor Perspect. Med., 2018, 8 DOI:10.1101/cshperspect.a031203.
- C. Ohlsson and K. Sjogren, Effects of the gut microbiota on bone mass, Trends Endocrinol. Metab., 2015, 26, 69–74 CrossRef CAS.
- G. R. Gibson, R. Hutkins, M. E. Sanders, S. L. Prescott, R. A. Reimer, S. J. Salminen, K. Scott, C. Stanton, K. S. Swanson, P. D. Cani, K. Verbeke and G. Reid, The International Scientific Association for Probiotics and Prebiotics (ISAPP) consensus statement on the definition and scope of prebiotics, Nat. Rev. Gastroenterol. Hepatol., 2017, 14, 491–502 CrossRef.
-
C. Whisner and C. Weaver, in Understanding the Gut-Bone Signaling Axis. Advances in Experimental Medicine and Biology, ed, L. McCabe and N. Parameswaran, Springer, Cham, 2017, pp. 201–224 Search PubMed.
- C. M. Whisner, B. Martin, M. Schoterman, C. Nakatsu, L. McCabe, G. McCabe, M. Wastney, E. Van den Heuvel and C. Weaver, Galacto-oligosaccharides increase calcium absorption and gut bifidobacteria in young girls: a double-blind cross-over trial, Br. J. Nutr., 2013, 110, 1292–1303 CrossRef CAS.
- C. Whisner, B. Martin, C. Nakatsu, J. Story, C. MacDonald-Clarke, L. McCabe, G. McCabe and C. Weaver, Soluble Corn Fiber Increases Calcium Absorption Associated With Shifts in the Gut Microbiome: A Randomized Dose-Response Trial in Free-Living Pubertal Females, J. Nutr., 2016, 146, 1298–1306 CrossRef CAS.
- S. Abrams, I. Griffin, K. Hawthorne, L. Liang, S. Gunn, G. Darlington and K. Ellis, A combination of prebiotic short- and long-chain inulin-type fructans enhances calcium absorption and bone mineralization in young adolescents, Am. J. Clin. Nutr., 2015, 82, 471–476 CrossRef.
- I. Griffin, P. Hicks, R. Heaney and S. Abrams, Enriched chicory inulin increases calcium absorption mainly in girls with lower calcium absorption, Nutr. Res., 2003, 23, 901–909 CrossRef CAS.
- E. G. van den Heuvel, T. Muys, W. van Dokkum and G. Schaafsma, Oligofructose stimulates calcium absorption in adolescents, Am. J. Clin. Nutr., 1999, 69, 544–548 CrossRef CAS.
- E. van den Heuvel, T. Muijs, F. Brouns and H. Hendriks, Short-chain fructo-oligosaccharides improve magnesium absorption in adolescent girls with a low calcium intake, Nutr. Res., 2009, 29, 229–237 CrossRef CAS.
- M. Tahiri, J. Tressol, J. Arnaud, F. Bornet, C. Bouteloup-Demange, C. Feillet-Coudray, M. Brandolini, V. Ducros, D. Pépin, F. Brouns, A. Roussel, Y. Rayssiguier and C. Coudray, Effect of short-chain fructooligosaccharides on intestinal calcium absorption and calcium status in postmenopausal women: a stable-isotope study, Am. J. Clin. Nutr., 2003, 77, 449–457 CrossRef CAS.
- S. Jakeman, C. Henry, B. Martin, G. McCabe, L. McCabe, G. Jackson, M. Peacock and C. Weaver, Soluble corn fiber increases bone calcium retention in postmenopausal women in a dose-dependent manner: a randomized crossover trial, Am. J. Clin. Nutr., 2016, 104, 837–843 CrossRef CAS.
- L. Holloway, S. Moynihan, S. Abrams, K. Kent, A. Hsu and A. Friedlander, Effects of oligofructose-enriched inulin on intestinal absorption of calcium and magnesium and bone turnover markers in postmenopausal women, Br. J. Nutr., 2007, 97, 365–372 CrossRef CAS.
- M. Slevin, P. Allsopp, P. Magee, M. Bonham, V. Naughton, J. Strain, M. Duffy, J. Wallace and E. Mc Sorley, Supplementation With Calcium and Short-Chain Fructo-Oligosaccharides Affects Markers of Bone Turnover but Not Bone Mineral Density in Postmenopausal Women, J. Nutr., 2014, 144, 297–304 CrossRef CAS.
- N. Drabińska, U. Krupa-Kozak, P. Abramowicz and E. Jarocka-Cyrta, Beneficial Effect of Oligofructose-Enriched Inulin on Vitamin D and E Status in Children with Celiac Disease on a Long-Term Gluten-Free Diet: A Preliminary Randomized, Placebo-Controlled Nutritional Intervention Study, Nutrients, 2018, 10, 1768–1776 CrossRef.
- N. Drabińska, E. Jarocka-Cyrta, D. Złotkowska, P. Abramowicz and U. Krupa-Kozak, Daily oligofructose-enriched inulin intake impacts bone turnover markers but not the cytokine profile in pediatric patients with celiac disease on a gluten-free diet: Results of a randomised, placebo-controlled pilot study, Bone, 2019, 122, 184–192 CrossRef.
- R. Rizzoli, Nutritional influence on bone: role of gut microbiota, Aging: Clin. Exp. Res., 2019, 31, 743–751 Search PubMed.
- F. Zhu, B. Du, Z. Bian and B. Xu, Beta-glucans from edible and medicinal mushrooms: Characteristics, physicochemical and biological activities, J. Food Compos. Anal., 2015, 41, 165–173 CrossRef CAS.
- G. Koutrotsios, N. Kalogeropoulos, A. C. Kaliora and G. I. Zervakis, Toward an Increased Functionality in Oyster (Pleurotus) Mushrooms Produced on Grape Marc or Olive Mill Wastes Serving as Sources of Bioactive Compounds, J. Agric. Food Chem., 2018, 66, 5971–5983 CrossRef CAS.
- M. L. Gargano, L. J. L. D. van Griensven, O. S. Isikhuemhen, U. Lindequist, G. Venturella, S. P. Wasser and G. I. Zervakis, Medicinal mushrooms: Valuable biological resources of high exploitation potential, Plant Biosyst., 2017, 151, 548–565 CrossRef.
- M. Jayachandran, J. Xiao and B. Xu, A critical review on health promoting benefits of edible mushrooms through gut microbiota, Int. J. Mol. Sci., 2017, 18, 1934–1945 CrossRef.
- G. Papathanasiou, G. Georgoudis, M. Papandreou, P. Spyropoulos, D. Georgakopoulos, V. Kalfakakou and A. Evangelou, Reliability measures of the short International Physical Activity Questionnaire (IPAQ) in Greek young adults, Hellenic J. Cardiol., 2009, 50, 283–294 Search PubMed.
- D. Matthews, J. Hosker, A. Rudenski, B. Naylor, D. Treacher and R. Turner, Homeostasis Model Assessment: Insulin Resistance and Beta-Cell Function From Fasting Plasma Glucose and Insulin Concentrations in Man, Diabetologia, 1985, 28, 412–419 CrossRef CAS.
- G. Koutrotsios, M. Patsou, E. Mitsou, G. Bekiaris, M. Kotsou, P. Tarantilis, V. Pletsa, A. Kyriacou and G. Zervakis, Valorization of Olive By-Products as Substrates for the Cultivation of Ganoderma lucidum and Pleurotus ostreatus Mushrooms with Enhanced Functional and Prebiotic Properties, Catalysts, 2019, 9, 537–554 CrossRef CAS.
- J. Wang, J. Zhang, B. Zhao, X. Wang, Y. Wu and J. Yao, A comparison study on microwave-assisted extraction of Potentilla anserina L. polysaccharides with conventional method: Molecule weight and antioxidant activities evaluation, Carbohydr. Polym., 2010, 80, 84–93 CrossRef CAS.
- W. Chen, W. P. Wang, H. S. Zhang and Q. Huang, Optimization of ultrasonic - assisted extraction of water - soluble polysaccharides from Boletus edulis mycelia using response surface methodology, Carbohydr. Polym., 2012, 87, 614–619 CrossRef CAS.
- A. C. Ruthes, F. R. Smiderle and M. Iacomini, D - glycans from edible mushrooms: A review on the extraction, purification and chemical characterization approaches, Carbohydr. Polym., 2015, 117, 753–761 CrossRef CAS.
- A. Synytsya, K. Mícková, A. Synytsya, I. Jablonsky, J. Spevácek, V. Erban, E. Kováríková and J. Copíková, Glucans from fruit bodies of cultivated mushrooms Pleurotus ostreatus and Pleurotus eryngii: Structure and potential prebiotic activity, Carbohydr. Polym., 2009, 76, 548–556 CrossRef CAS.
- E. K. Mitsou, A. Kakali, S. Antonopoulou, K. C. Mountzouris, M. Yannakoulia, D. B. Panagiotakos and A. Kyriacou, Adherence to the Mediterranean diet is associated with the gut microbiota pattern and gastrointestinal characteristics in an adult population, Br. J. Nutr., 2017, 117, 1645–1655 CrossRef CAS.
- E. Olano-Martin, K. C. Mountzouris, G. R. Gibson and R. A. Rastall,
In vitro fermentability of dextran, oligodextran and maltodextrin by human gut bacteria, Br. J. Nutr., 2000, 83, 247–255 CrossRef CAS.
- C. Rycroft, M. Jones, G. Gibson and R. Rastall, A comparative in vitro evaluation
of the fermentation properties of prebiotic oligosaccharides, J. Appl. Microbiol., 2001, 91, 878–887 CrossRef CAS.
- E. K. Mitsou, G. Saxami, E. Stamoulou, E. Kerezoudi, E. Terzi, G. Koutrotsios, G. Bekiaris, G. I. Zervakis, K. C. Mountzouris, V. Pletsa and A. Kyriacou, Effects of rich in β-glucans edible mushrooms on aging gut microbiota characteristics: an in vitro study, Molecules, 2020, 25, 2806–2830 CrossRef CAS.
- M. Hamzeloo-Moghadam, N. Taiebi, M. Mosaddegh, B. E. Tehrani and S. Esmaeili, The effect of some cosolvents and surfactants on viability of cancerous cell lines, Res. J. Pharmacogn., 2014, 1, 41–45 CAS.
- N. Zhang, X. Huang, Y. Zeng, X. Wu and X. Peng, Study on prebiotic effectiveness of neutral garlic fructan in vitro, Food Sci. Hum. Wellness, 2013, 2, 119–123 CrossRef.
- R. J. Palframan, G. R. Gibson and R. A. Rastall, Effect of pH and dose on the growth of gut bacteria on prebiotic carbohydrates in vitro, Anaerobe, 2002, 8, 287–292 CrossRef CAS.
- A. Salonen, J. Nikkilä, J. Jalanka-Tuovinen, O. Immonen, M. Rajilić-Stojanović, R. A. Kekkonen, A. Palva and W. M. De Vos, Comparative analysis of fecal DNA extraction methods with phylogenetic microarray: effective recovery of bacterial and archaeal DNA using mechanical cell lysis, J. Microbiol. Methods, 2010, 81, 127–134 CrossRef CAS.
- Z. Yu and M. Morrison, Improved extraction of PCR-quality community DNA from digesta and fecal samples, BioTechniques, 2004, 36, 808–812 CrossRef CAS.
- E. Olano-Martin, G. Gibson and R. Rastall, Comparison of the in vitro bifidogenic properties of pectins and pectic-oligosaccharides, J. Appl. Microbiol., 2002, 93, 505–511 CrossRef CAS.
- R. Palframan, G. Gibson and R. Rastall, Development of a quantitative tool for the comparison of the prebiotic effect of dietary oligosaccharides, Lett. Appl. Microbiol., 2003, 37, 281–284 CrossRef CAS.
- E. Mitsou, E. Kougia, T. Nomikos, M. Yannakoulia, K. Mountzouris and A. Kyriacou, Effect of banana consumption on faecal microbiota: a randomised, controlled trial, Anaerobe, 2011, 17, 384–387 CrossRef CAS.
- K. C. Mountzouris, C. Balaskas, F. Fava, K. M. Tuohy, G. R. Gibson and K. Fegeros, Profiling of composition and metabolic activities of the colonic microflora of growing pigs fed diets supplemented with prebiotic oligosaccharides, Anaerobe, 2006, 12, 178–185 CrossRef CAS.
- D. Lajeunesse, C. Frondoza, B. Schoffield and B. Sacktor, Osteocalcin secretion by the human osteosarcoma cell line MG-63, J. Bone Miner. Res., 1990, 5, 915–922 CrossRef CAS.
- D. Lajeunesse, G. Kiebzak, C. Frondoza and B. Sacktor, Regulation of osteocalcin secretion by human primary bone cells and by the human osteosarcoma cell line MG-63, Bone Miner., 1991, 14, 237–250 CrossRef CAS.
- R. Franceschi, W. James and G. Zerlauth, 1 alpha, 25-dihydroxyvitamin D3 specific regulation of growth, morphology, and fibronectin in a human osteosarcoma cell line, J. Cell. Physiol., 1985, 123, 401–409 CrossRef CAS.
- B. Boyan, Z. Schwartz, L. Bonewald and L. Swain, Localization of 1,25-(OH)2D3-responsive alkaline phosphatase in osteoblast-like cells (ROS 17/2.8, MG 63, and MC 3 T3) and growth cartilage cells in culture, J. Biol. Chem., 1989, 264, 11879–11886 CrossRef CAS.
- D. T. Vistica, P. Skehan, D. Scudiero, A. Monks, A. Pittman and M. R. Boyd, Tetrazolium-based assays for cellular viability: a critical examination of selected parameters affecting formazan production, Cancer Res., 1991, 51, 2515–2520 CAS.
- G. Schaafsma and J. L. Slavin, Significance of Inulin Fructans in the Human Diet, Compr. Rev. Food Sci. Food Saf., 2015, 14, 37–47 CrossRef CAS.
- M. Rossi, C. Corradini, A. Amaretti, M. Nicolini, A. Pompei, S. Zanoni and D. Matteuzzi, Fermentation of fructooligosaccharides and inulin by bifidobacteria: a comparative study of pure and fecal cultures, Appl. Environ. Microbiol., 2005, 71, 6150–6158 CrossRef CAS.
- F. Moens and L. De Vuyst, Inulin-type fructan degradation capacity of Clostridium cluster IV and XIVa butyrate-producing colon bacteria and their associated metabolic outcomes, Benefic. Microbes, 2017, 8, 473–490 CrossRef CAS.
- P. Louis and H. J. Flint, Formation of propionate and butyrate by the human colonic microbiota, Environ. Microbiol., 2017, 19, 29–41 CrossRef CAS.
- N. T. Baxter, A. W. Schmidt, A. Venkataraman, K. S. Kim, C. Waldron and T. M. Schmidt, Dynamics of Human Gut Microbiota and Short-Chain Fatty Acids in Response to Dietary Interventions with Three Fermentable Fibers, mBio, 2019, 10 DOI:10.1128/mBio.02566-18.
- R. Zhao, W. Yang, F. Pei, L. Zhao and Q. Hu,
In vitro fermentation of six kinds of edible mushrooms and its effects on fecal microbiota composition, LWT–Food Sci. Technol., 2018, 96, 627–635 CrossRef CAS.
- G. Ma, B. Kimatu, L. Zhao, W. Yang, F. Pei and Q. Hu,
In vivo fermentation of a Pleurotus eryngii polysaccharide and its effects on fecal microbiota composition and immune response, Food Funct., 2017, 8, 1810–1821 RSC.
- O. Taofiq, S. A. Heleno, R. C. Calhelha, M. J. Alves, L. Barros, A. M. Gonzalez-Paramas, M. F. Barreiro and I. C. Ferreira, The potential of Ganoderma lucidum extracts as bioactive ingredients in topical formulations, beyond its nutritional benefits, Food Chem. Toxicol., 2017, 108, 139–147 CrossRef CAS.
- I. Khan, G. Huang, X. Li, W. Leong, W. Xia and W. W. Hsiao, Mushroom polysaccharides from Ganoderma lucidum and Poria cocos reveal prebiotic functions, J. Funct. Foods, 2018, 41, 191–201 CrossRef CAS.
- S. Yamin, M. Shuhaimi, A. Arbakariya, A. Fatimah, A. Khalilah, O. Anas and A. Yazid, Effect of Ganoderma lucidum polysaccharides on the growth of Bifidobacterium spp. as assessed using Real-time PCR, Int. Food Res. J., 2012, 19, 1199–1205 CAS.
- M. Chang, Y. Tsai, E. Liou, C. Tang, T. Wang, H. Liu, M. Liao, S. Yeung, C. Chan and J. Jeng, Effect of butyrate on collagen expression, cell viability, cell cycle progression and related proteins expression of MG-63 osteoblastic cells, PLoS One, 2016, 11 DOI:10.1371/journal.pone.0165438.
- D. Meyer and M. Stasse-Wolthuis, The bifidogenic effect of inulin and oligofructose and its consequences for gut health, Eur. J. Clin. Nutr., 2009, 63, 1277–1289 CrossRef CAS.
- M. Roberfroid, Prebiotics: the concept revisited, J. Nutr., 2007, 137, 830–837 CrossRef.
- T. Zhang, Y. Yang, Y. Liang, X. Jiao and C. Zhao, Beneficial Effect of Intestinal Fermentation of Natural Polysaccharides, Nutrients, 2018, 10, 1055–1076 CrossRef.
- H. Flint, P. Louis and S. Duncan, The role of the gut microbiota in nutrition and health, Gastroenterol. Hepatol., 2012, 9, 577–589 CAS.
- R. O. Corrêa, J. L. Fachi, A. Vieira, F. T. Sato and M. A. R. Vinolo, Regulation of immune cell function by short-chain fatty acids, Clin. Transl. Immunol., 2016, 5, 73–80 CrossRef.
- Y. Feng, Y. Wang, P. Wang, Y. Huang and F. Wang, Short-Chain Fatty Acids Manifest Stimulative and Protective Effects on Intestinal Barrier Function Through the Inhibition of NLRP3 Inflammasome and Autophagy, Cell. Physiol. Biochem., 2018, 49, 190–205 CrossRef CAS.
- E. Hosseini, C. Grootaert, W. Verstraete and T. Van de Wiele, Propionate as a health-promoting microbial metabolite
in the human gut, Nutr. Rev., 2011, 69, 245–258 CrossRef.
- H. Hamer, V. De Preter, K. Windey and K. Verbeke, Functional analysis of colonic bacterial metabolism: Relevant to health, Am. J. Physiol.: Gastrointest. Liver Physiol., 2012, 302, 1–9 CrossRef.
- G. Besten, K. van Eunen, A. Groen, K. Venema, D. Reijngoud and B. Bakker, The Role of Short-Chain Fatty Acids in the Interplay between Diet, Gut Microbiota, and Host Energy Metabolism, J. Lipid Res., 2013, 54, 2325–2340 CrossRef.
- M. Bao, K. Zhang, Y. Wei, W. Hua, Y. Gao, X. Li and L. Ye, Therapeutic potentials and modulatory mechanisms of fatty acids in bone, Cell Proliferation, 2019, 53, 12735–12762 Search PubMed.
- S. Lucas, Y. Omata, J. Hofmann, M. Böttcher, A. Iljazovic, K. Sarter, O. Albrecht, O. Schulz, B. Krishnacoumar, G. Krönke, M. Herrmann, D. Mougiakakos, T. Strowig, G. Schett and M. M. Zaiss, Short-chain fatty acids regulate systemic bone mass and protect from pathological bone loss, Nat. Commun., 2018, 9, 55–64 CrossRef.
- T. Katono, T. Kawato, N. Tanabe, N. Suzuki, T. Iida, A. Morozumi, K. Ochiai and M. Maeno, Sodium butyrate stimulates mineralized nodule formation and osteoprotegerin expression by human osteoblasts, Arch. Oral Biol., 2008, 53, 903–909 CrossRef CAS.
- Q. Ding, S. Nie, J. Hu, X. Zong, Q. Li and M. Xie,
In vitro and in vivo gastrointestinal digestion and fermentation of the polysaccharide from Ganoderma atrum, Food Hydrocolloids, 2017, 63, 646–655 CrossRef CAS.
- E. Heimann, M. Nyman, A. Pålbrink, K. Lindkvist-Petersson and E. Degerman, Branched short-chain fatty acids modulate glucose and lipid metabolism in primary adipocytes, Adipocyte, 2016, 5, 359–368 CrossRef CAS.
- C. K. Yao, J. Muir and P. Gibson, Review article: insights into colonic protein fermentation, its modulation and potential health implications, Aliment. Pharmacol. Ther., 2016, 43, 181–196 CrossRef CAS.
- D. Roy, A. Azad, F. Sultana, A. Anisuzzaman and P. Khondkar, Nutritional profile and mineral composition of two edible mushroom varieties consumed and cultivated in Bangladesh, J. Phytopharm., 2015, 4, 217–220 Search PubMed.
- R. Singh, N. Kaur, R. Shri, A. Singh and G. Dhingra, Proximate composition and element contents of selected species of Ganoderma with reference to dietary intakes, Environ. Monit. Assess., 2020, 192, 270–284 CrossRef CAS.
- K. Iwami and T. Moriyama, Effects of short chain fatty acid, sodium butyrate, on osteoblastic cells and osteoclastic cells, Int. J. Biochem., 1993, 25, 1631–1635 CrossRef CAS.
- M. Rahman, A. Kukita, T. Kukita, T. Shobuike, T. Nakamura and O. Kohashi, Two histone deacetylase inhibitors, trichostatin A and sodium butyrate, suppress differentiation into osteoclasts but not into macrophages, Blood, 2003, 101, 3451–3459 CrossRef CAS.
- A. Morozumi, High concentration of sodium butyrate suppresses osteoblastic differentiation and mineralized nodule formation in ROS17/2.8 cells, J. Oral Sci., 2011, 53, 509–516 CrossRef CAS.
- M. Chang, Y. Chen, Y. Lian, B. Chang, C. Huang, W. Huang, Y. Pan and J. Jeng, Butyrate Stimulates Histone H3 Acetylation, 8-Isoprostane Production, RANKL Expression, and Regulated Osteoprotegerin Expression/Secretion in MG-63 Osteoblastic Cells, Int. J. Mol. Sci., 2018, 19, 4071–4088 CrossRef.
- J. Liu, J. Shiono, K. Shimizu and R. Kondo, Ganoderic acids from Ganoderma lucidum: inhibitory activity of osteoclastic differentiation and structural criteria, Planta Med., 2010, 76, 137–139 CrossRef CAS.
- I. Miyamoto, J. Liu, K. Shimizu, M. Sato, A. Kukita and R. Kondo, Regulation of osteoclastogenesis by ganoderic acid DM isolated from Ganoderma lucidum, Eur. J. Pharmacol., 2009, 602, 1–7 CrossRef CAS.
- P. T. Tran, N. T. Dat, N. H. Dang, P. V. Cuong, S. Lee, C. Hwangbo, C. V. Minh and J.-H. Lee, Ganomycin I from Ganoderma lucidum attenuates RANKL-mediated osteoclastogenesis by inhibiting MAPKs and NFATc1, Phytomedicine, 2018, 55, 1–8 CrossRef.
Footnote |
† Electronic supplementary information (ESI) available. See DOI: 10.1039/d0fo02581j |
|
This journal is © The Royal Society of Chemistry 2021 |
Click here to see how this site uses Cookies. View our privacy policy here.