Spiers Memorial Lecture: Coordination networks that switch between nonporous and porous structures: an emerging class of soft porous crystals
Received
1st June 2021
, Accepted 14th June 2021
First published on 14th June 2021
Abstract
Coordination networks (CNs) are a class of (usually) crystalline solids typically comprised of metal ions or cluster nodes linked into 2 or 3 dimensions by organic and/or inorganic linker ligands. Whereas CNs tend to exhibit rigid structures and permanent porosity as exemplified by most metal–organic frameworks, MOFs, there exists a small but growing class of CNs that can undergo extreme, reversible structural transformation(s) when exposed to gases, vapours or liquids. These “soft” or “stimuli-responsive” CNs were introduced two decades ago and are attracting increasing attention thanks to two features: the amenability of CNs to design from first principles, thereby enabling crystal engineering of families of related CNs; and the potential utility of soft CNs for adsorptive storage and separation. A small but growing subset of soft CNs exhibit reversible phase transformations between nonporous (closed) and porous (open) structures. These “switching CNs” are distinguished by stepped sorption isotherms coincident with phase transformation and, perhaps counterintuitively, they can exhibit benchmark properties with respect to working capacity (storage) and selectivity (separation). This review addresses fundamental and applied aspects of switching CNs through surveying their sorption properties, analysing the structural transformations that enable switching, discussing structure–function relationships and presenting design principles for crystal engineering of the next generation of switching CNs.
1. Introduction
Coordination networks (CNs)1 are a class of covalent network solids comprised of metal or metal clusters (nodes) connected in two or more directions by organic (e.g. metal–organic frameworks, MOFs2,3), inorganic (e.g. Prussian blue and its analogues, PBAs4,5) or combinations of organic and inorganic (e.g. hybrid ultramicroporous materials, HUMs6) linker ligands (Fig. 1). CNs are a subset of coordination polymers (CPs)7,8 and represent an extraordinarily diverse and growing class of metal–organic materials (MOMs).9,10 Interest in CNs is at least partly due to their inherent modularity and amenability to crystal engineering or reticular chemistry,11,12 which in turn facilitates the generation of closely related families of materials that allow for systematic structure–function studies. One property in particular has attracted the attention of researchers, namely porosity. Porosity is a direct outcome of the “node-and-linker” approach to the design of CNs that can be credited to Robson and Hoskins, who introduced the concept as a design principle for the generation of porous solids over 30 years ago.13,14 The existence of porosity deservedly attracts attention but is not in itself the only thing that makes CNs of special interest. Rather, it is the inherent modularity of CNs that brings with it an ability to design pore size, shape and chemistry. This in turn enables fine-tuning of properties to create potential utility for new energy-efficient technologies in areas of societal need, e.g. gas/vapour storage, separations, water purification, drug delivery and catalysis.15–19 Thus far, around 100
000 CNs have been deposited in the MOF subset of the Cambridge Structural Database (CSD),20 thereby accounting for ca. 10% of entries in the CSD.21 The majority, perhaps >99%, of CNs thus far reported can be classified as “first generation” or “second generation” CPs,7,22–24 which either undergo structural collapse (first generation) or possess rigid structures like zeolites (second generation) upon guest removal, respectively. Herein, we address a small subset of “third generation” or “soft” CPs that are stimulus responsive in that they change structure when exposed to gases, vapours or liquids.
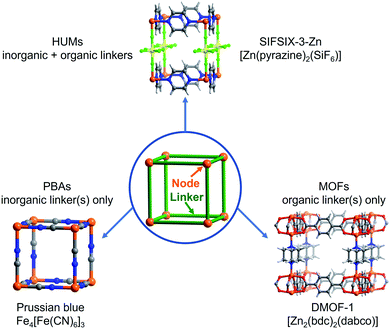 |
| Fig. 1 CNs can be classified into three subsets by the type of linker ligands: inorganic only (e.g. PBAs); organic only (e.g. MOFs); hybrid (e.g. HUMs). The three subsets are exemplified by Prussian blue, DMOF-1 and SIFSIX-3-Zn, respectively. | |
Seminal discoveries in the late 1990s and early 2000s25–29 introduced “third generation” CPs7,22–24 or “soft porous crystals”.30,31 Third generation CNs exhibit structural flexibility when exposed to external stimuli such as light,32 heat,33 mechanical pressure34 and/or guest molecules.35–37 Pioneered by Kitagawa, Férey and others,7,22–24,38–41 flexible CNs have been comprehensively reviewed from different perspectives such as smart switches42,43 multiscale design,44in situ characterisation,45 controlled flexibility design,46 simulation and computational studies47 and overall progress.48–50 Herein we focus upon a small but growing and potentially important subset of flexible CNs, namely those 2D (e.g. ELM-11 (ref. 28)) and 3D (e.g. [Cu2(bdc)2(bpy)]51) CNs that switch between closed (nonporous) and open (porous) phases.
A variety of terms have been coined to describe the flexibility of CNs including “accordionlike”,26 “springlike”,27,52 “spongelike”,53,54 “breathing”,55 “swelling”,56,57 “soft”,30,31,58 “elastic”,59,60 “dynamic”,24,61,62 “stimuli-responsive”,47,63,64 and “gate-opening”.28,65,66 An alternate approach to classify flexible CNs is to use their sorption isotherms and we have proposed a classification system based upon sorption isotherm types, namely type F-I to type F-V.67 IUPAC has classified type I isotherms as being characteristic of rigid microporous adsorbents thanks to the enhanced adsorbent–adsorbate interactions which results in micropore filling at very low relative pressure (Fig. 2a).68 In contrast, flexible microporous CNs usually exhibit distinct “stepped” or “S-shaped” isotherm profiles that are yet to be formally classified by IUPAC. When flexible CNs retain porosity after activation, a type I-like profile will be followed by the second step at a threshold pressure that coincides with a structural transformation from a less open phase to a more open phase. This transformation could be gradual (type F-I, e.g. the “breathing” effect in MIL-53(Cr)69) or sudden (type F-II, e.g. the “gate-opening” effect in ELM-12 (ref. 70)) (Fig. 2b). When flexible CNs are nonporous after activation, the transformation from a nonporous (closed) to a porous (open) phase can also occur gradually (type F-III, e.g. the “swelling” effect in [Co2(BME-bdc)2(dabco)]71) or suddenly (type F-IV, e.g. the “switching” effect in [Zn2(BME-bdc)2(dabco)]71) (Fig. 2c). In addition, type F-IV isotherms can be further sub-divided depending on the number of sorption steps, i.e., single-step type F-IVs or multiple-step (≥2) type F-IVm (Fig. 2d).72 We note that most of the flexible CNs reported to date undergo reversible structural changes in response to the presence/absence of external stimuli and that there are very few examples of flexible CNs that do not return to their activated forms after full desorption. There are, however, CNs that retain the structure of the fully open stage after the first sorption cycle and exhibit type I sorption isotherms during the subsequent cycles (type F-V, e.g. the “shape memory” effect in X-pcu-3-Zn-3i73) (Fig. 2e). Amongst the various isotherm types in Fig. 2, flexible CNs that exhibit type F-IV isotherms are perhaps the most desirable with respect to gas storage as they can offer higher working capacity than rigid porous materials with type I isotherms (Fig. 2f).72,74 It should be mentioned that hysteresis is a feature of most type F-IV isotherms and, to be of practical utility, the delivery pressure (Pde) must be lower than the desorption branch rather than the adsorption branch (Fig. 2f).
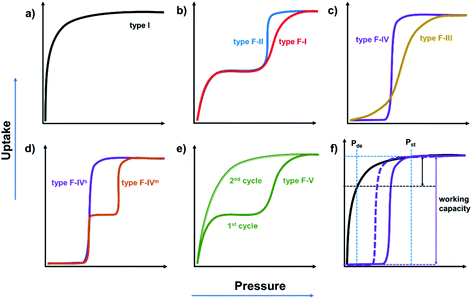 |
| Fig. 2 Schematic illustration of sorption isotherm types exhibited by CNs. (a) Type I, typical of rigid microporous CNs; (b) type F-I (open to more open, gradual) and F-II (open to more open, sudden), associated with flexible microporous CNs; (c) type F-III (closed to open, gradual) and F-IV (closed to open, sudden) associated with switching nonporous CNs; (d) type F-IV isotherms can be sub-divided into single-step type F-IVs and multiple-step (≥2) type F-IVm isotherms; (e) type F-V isotherms are indicative of a shape memory effect in second and subsequent consecutive sorption cycles; (f) comparison of working capacity between type I and F-IV isotherms with similar surface areas: black line = adsorption branch of type I isotherm (desorption branch overlays); solid/dash purple lines = adsorption and desorption branches of type F-IV isotherm, respectively; Pde: delivery pressure; Pst: storage pressure. | |
We adopt the term “switching” herein as it is consistent with the “on/off” nature of CNs that exhibit reversible “closed/open” structural changes of the type that affords type F-IV isotherms. Switching has also been used for other on/off events in materials chemistry such as thermal expansion/shrinkage, spin crossover, redox, photochromism, photoisomerization and valence tautomerism.42,43,75–77 In the following sections, we analyse and discuss reversible switching in the context of guest sorption by presenting case studies of switching CNs with particular emphasis upon structure–property relationships and the influence of variables such as metal node, linker ligand, and adsorbate.
2. Switching CNs
Whereas coordination complexes and organic molecules are long-known for the ability to exhibit guest inclusion or clathration that accompanies switching between closed and open phases,78–83 the first examples of 2D and 3D switching CNs were not reported until the beginning of the 21st century (Fig. 3).28,51 Werner complexes are prototypal coordination compounds and their guest-clathration ability was systematically studied by Schaeffer in 1957.78 The associated sorption isotherms were reported in 1969 by Barrer’s group, who studied the sorption behaviour of several Werner complexes upon exposure to a range of gases and vapours.79 Type F-IV isotherms were observed when the Werner complex [Co(etpy)4(NCS)2] was exposed to benzene, toluene and xylenes and this phenomenon was attributed to closed/open phase transformations. We recently reported a related example of such a Werner complex and termed it as a Switching Adsorbent Molecular Material (SAMM).83 The first 2D switching CN, [Cu(bpy)2(BF4)2], ELM-11,28 was reported in 2001 and can be regarded as being comprised of linked Werner complexes which serve as molecular building blocks (MBBs84). The first 3D switching CN, [Cu2(bdc)2(bpy)],51 was reported in 2002 and features a 2D square lattice layer comprised of linked “paddle-wheel” MBBs that is pillared by bpy linkers to form a 2-fold interpenetrated 3D network. Altering the length of the vertical pillar and horizontal linker afforded a non-interpenetrated switching CN DUT-8(Ni) which set benchmark uptakes for N2 (77 K) and CO2 (195 K) in switching CNs.113,114 Hybrid CNs6 with primitive cubic (pcu) topology can be generated from 2D square lattice (sql) CNs when an inorganic counter anion such as hexafluorosilicate can serve as a pillar ligand, as exemplified by the first switching hybrid CN, SIFSIX-23-Cu.151 Whereas the coordination spheres of Werner complexes, 2D sql CNs and 3D pcu CNs are chemically related, their dimensional rigidities can be different.40 In 2008, type F-IVm isotherms were observed in Co(bdp),101 which later set a benchmark for volumetric CH4 working capacity in the context of switching CNs.74 Although MIL-53 CNs were reported as early as 2002, a switching variant, MIL-53(Fe), was not introduced until 2009.106 X-dia-1-Ni, reported in 2018, was the first example of a diamondoid (dia) topology switching CN and it was found to exhibit the second highest CH4 working capacity in switching CNs.67 Overall, around 60 switching CNs have now been reported (Table 1), with sql and pcu topology CNs being the most common 2D and 3D switching CNs, respectively. Whereas 2D switching CNs were reported first, the proportion of 3D switching CNs is currently much higher than 2D switching CNs (∼4
:
1). This is likely a reflection of the larger number of 3D CNs (especially MOFs) that have been studied for their sorption properties in the past two decades but should not imply any prejudice in favour of modularity or properties for 3D CNs versus 2D CNs.
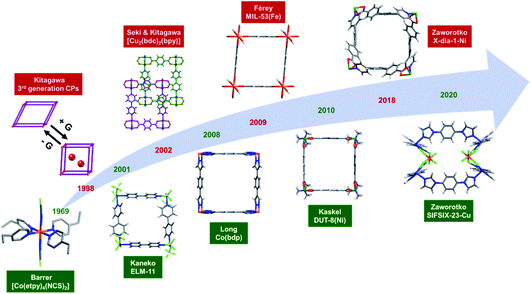 |
| Fig. 3 Chronology of key developments related to switching CNs. 1969 (Barrer): type F-IVs sorption isotherms observed in the Werner complex [Co(etpy)4(NCS)2]; 1998 (Kitagawa): 3rd generation CPs defined; 2001 (Kaneko): first 2D switching CN, ELM-11, reported; 2002 (Seki & Kitagawa): first 3D switching and “shape-memory” CN, [Cu2(bdc)2(bpy)], detailed; 2008 (Long): type F-IVm sorption isotherms observed in Co(bdp), currently the benchmark switching CN for CH4 storage; 2009 (Férey): reported the most widely studied switching CN: MIL-53(Fe); 2010 (Kaskel): benchmark N2 (77 K) and CO2 (195 K) uptakes for DUT-8(Ni); 2018 (Zaworotko): first dia topology switching CN, X-dia-1-Ni, and classification of the isotherm types for flexible CNs; 2020 (Zaworotko): first example of switching hybrid CN with inorganic and organic linkers, SIFSIX-23-Cu, published. | |
Table 1 Tabulated chronology (2001–2020) of switching CNs
CNs |
Dimension |
Guests |
Year |
Ref. |
ELM-11 |
2D |
N2, Ar, O2, CH4, CO2, C2H2, C4H10 |
2001 |
28, 72 and 85–90 |
[Co(NCS)2(3-pia)2] |
2D |
Me2CO |
2002 |
91
|
[Cu2(pzdc)2(dpyg)] |
3D |
MeOH, H2O |
2002 |
92
|
[Cu2(bdc)2(bpy)] |
3D |
N2, CH4, MeOH, CO2 |
2002 |
51 and 93 |
[Cu(dhbc)2(bpy)] |
2D |
O2, N2, CH4, CO2 |
2003 |
65 and 94 |
[Co(NCS)2(4-peia)2] |
2D |
Me2CO |
2004 |
95
|
[Cu(pyrdc)(bpp)] |
2D |
CO2, MeOH, EtOH |
2005 |
96
|
MOF-508 |
3D |
N2, H2, CO2, C2H2 |
2006 |
97–99
|
[Zn(TCNQ)2(bpy)] |
3D |
C6H6 |
2007 |
100
|
[Cd(bpndc)(bpy)] |
2D |
O2, N2, Ar |
2008 |
66
|
Co(bdp)/Fe(bdp) |
3D |
N2, H2, CH4, CO2 |
2008 |
74 and 101–103 |
ELM-31 |
2D |
CO2 |
2009 |
104
|
SNU-M11/SNU-M10 |
3D |
CO2 |
2009 |
105
|
MIL-53(Fe) |
3D |
CH4, C2H6, C3H8, C4H10, CO2, C8H10 |
2009 |
106–108
|
[Cd2(pzdc)2L] |
3D |
CO2 |
2009 |
109
|
[Zn(pydc)(dma)] |
3D |
N2, Ar, CO2, H2, CH4 |
2009 |
110
|
Zn(GA)2 |
3D |
CO2 |
2010 |
111
|
CID-5 |
2D |
CO2 |
2010 |
112
|
DUT-8(Ni) |
3D |
CO2, N2, Xe, C4H10, C2H4, C2H6, MeCN, C7H8, C7H16, CH2Cl2, CHCl3, CCl4 |
2010 |
113–117
|
[Zn2(bpdc)2(bpee)] |
3D |
N2, Ar, CO2, C2H2, C2H4, C2H6, C3H6, C3H8, C4H10 |
2010 |
118 and 119 |
[Zn2(bdc)2(dfbpb)] |
3D |
O2, Ar, CO2 |
2011 |
120
|
[Zn(DIP-bdc)2(dabco)] |
3D |
CO2 |
2012 |
121
|
[Zn(DB-bdc)2(dabco)] |
MIL-53(Sc) |
3D |
CO2 |
2012 |
122 and 123 |
[Ni(bdc)(bphy)] |
2D |
CO2 |
2012 |
124
|
[Cu(CF3SO3)2(bpp)2] |
2D |
O2, CO2 |
2013 |
125
|
[Zn2L2] |
3D |
N2, CH4 |
2014 |
126
|
DynaMOF-100 |
3D |
C8H10, C8H8 |
2014 |
127 and 128 |
JLU-Liu3/JLU-Liu4 |
3D |
N2 |
2014 |
129
|
[Ag2(L15)2] |
3D |
CO2 |
2014 |
130
|
[Sm(HL)(DMA)2] |
3D |
CO2, CH2Cl2 |
2015 |
131
|
f-MOF-1/f-MOF-2 |
3D |
CO2 |
2016 |
132
|
Co(F2-bdp)/Co(Me2-bdp) |
3D |
N2, CH4 |
2016 |
133
|
Mn(ina)2 |
3D |
C2H6, C3H6, C3H8 |
2016 |
134
|
JLU-Liu33 |
3D |
N2 |
2017 |
135
|
[Zn2(DPT)2(bpy)] |
3D |
CO2 |
2017 |
136
|
[Zn3(bdc)2(tz)2] |
3D |
C2H6, C3H8, C4H10 |
2017 |
137
|
[Co(VTTF)] |
2D |
C2H4 |
2017 |
138
|
DUT-98 |
3D |
N2, H2O |
2017 |
139 and 140 |
CPM-325 |
3D |
N2, CO2 |
2018 |
141
|
Cd(miba)2 |
3D |
CO2 |
2018 |
142
|
[Zn2(tdc)2(pvq)] |
3D |
N2 |
2018 |
143
|
Zn2(BME-bdc)2(dabco) |
3D |
CO2 |
2018 |
71
|
X-dia-1-Ni |
3D |
CO2, CH4 |
2018 |
67
|
sql-1-Co-NCS |
2D |
CO2, C8H10 |
2018 |
144 and 145 |
X-pcu-n-Zn, n = 5, 6, 7, 8 |
3D |
CO2, C2H2 |
2018 |
146 and 147 |
NJU-Bai8 |
3D |
C3H6, C3H8 |
2018 |
148
|
[Zn2(ndc)2(bpa)] |
3D |
CO2 |
2019 |
149
|
[Cu(HIsa-az-dmpz)] |
3D |
N2, CO2 |
2019 |
150
|
SIFSIX-23-Cu (NTU-65) |
3D |
N2, CO2, C2H2, C2H4 |
2020 |
151 and 152 |
ELM-13 |
2D |
N2, O2, Ar, NO, CO2 |
2020 |
153
|
JUK-8 |
3D |
CO2, H2O |
2020 |
154
|
Switching between a closed and a single open phase generally results in single-step type F-IVs isotherms51,65,66,71,74 whereas switching between closed and multiple open phases usually affords multiple-step type F-IVm isotherms (Fig. 2d and 4).72,85–87 The number of structural transitions and/or sorption steps in a switching CN can be influenced by factors such as temperature, pressure and adsorbate as exemplified by ELM-11.72,85–87 Several of the prototypal switching CNs are modular and amenable to crystal engineering through systematic variation of metal moieties, linker ligands, sorbates or a combination thereof. Importantly, in many published reports, both the closed and open phases are structurally characterized and/or computationally modelled, thereby providing insight into both the mechanism of switching and the nature of the sorbent–sorbate interactions that drive the switching event. In the following sections, we present case studies of representative switching CNs and succinctly analyse their switching behaviour.
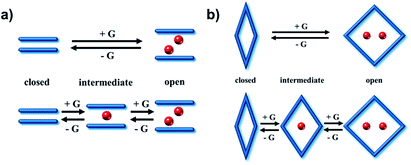 |
| Fig. 4 Schematic illustration of the types of structural transformations in (a) 2D and (b) 3D switching CNs induced by sorbate/guest molecules (G, red balls). Transformation between closed and open phases typically results in a single-step type F-IVs isotherm (top). Transformation between closed, intermediate (partially open) and fully open phases generally leads to a type F-IVm isotherm (bottom). | |
2.1. Examples of 3D switching CNs
2.1.1 MIL-53(M) family [M(OH)(bdc)].
The MIL-53 family of CNs represents perhaps the most widely studied family of flexible CNs thanks to the contributions of Férey and others.155 The metals (M) of MIL-53 are trivalent, e.g. Cr, Al, Fe, Sc, Ga or In.156–161 The structure of MIL-53(M) CNs is sustained by infinite trans corner sharing [MO4(OH)2] octahedra that form 1D chains by serving as rod building blocks, RBBs,162–164 which are cross-linked through bdc ligands (bdc = 1,4-benzenedicarboxylate) to afford sra topology networks (Fig. 5).162 The resulting 1D rhombic tunnels are occupied by solvent or guest molecules. The first members of the MIL-53(M) family, MIL-53(Cr) and MIL-53(Al), were reported in 2002 and 2003, respectively.156,157 Initial studies focused on structural transformations during hydration–dehydration processes.165,166 Large and reversible phase transformation between the activated “open” phase (MIL-53ht, ht = high temperature) and hydrated “contracted” phase (MIL-53lt, lt = low temperature) was revealed and this “breathing” phenomenon remains a rarity even after two decades. A superhydrated “open” phase was obtained by immersing MIL-53(Cr) in water and was reported in 2011.167
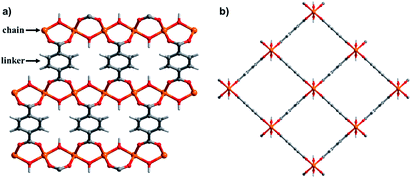 |
| Fig. 5 Crystal structures of MIL-53(M): (a) MO4(OH)2 chains connected by bdc linkers; (b) 1D rhombic channels in the open phase, guest molecules are omitted for clarity. Orange: M (Cr, Al, Fe, etc.), red: O, grey: C, light grey: H. | |
Gas sorption studies involving N2, H2, CH4 and CO2 on MIL-53(Cr, Al) revealed guest-dependent sorption profiles.69,156,157 For N2, H2 and CH4, the sorption isotherms are type I as expected for rigid microporous CNs and zeolites (Fig. 2a). In contrast, for CO2 MIL-53(Cr, Al) were observed to exhibit type F-I sorption isotherms (Fig. 6a).69 This was attributed to CO2 molecules interacting strongly with the hydroxide moieties that line the rhombic tunnels, resulting in pore contraction at low pressure and pore opening at high pressure.168 A two-step type F-I isotherm was observed with polar vapours (e.g. MeOH, EtOH) and C2–C8 hydrocarbon sorbates.169–171
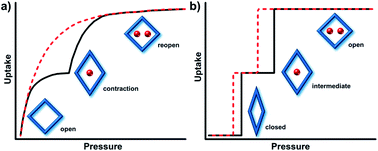 |
| Fig. 6 Schematic illustrations of the sorption isotherm types reported for the MIL-53(M) family. (a) Type F-I isotherms with open to less open to open transformations as observed in MIL-53(Cr, Al); (b) type F-IVm isotherm with closed to open to more open transformations as seen for MIL-53(Fe, Sc). Black solid line: adsorption curve; red dash line: desorption curve. | |
Substitution of the metal nodes by Fe(III) or Sc(III) resulted in nonporous “closed” phases in MIL-53(Fe or Sc) after activation.122,123,172 Compared to MIL-53(Sc), MIL-53(Fe) has been more widely subjected to study. CO2 sorption on MIL-53(Fe) revealed a multi-step type F-IVm isotherm (Fig. 6b).107 Other gases such as light hydrocarbons were observed to exhibit the same trend but with adsorbate-dependent switching pressures and uptakes (Fig. 7a).106 The structural transformations of MIL-53(Fe) involve at least two intermediate phases (ip1 and ip2) in between its closed (cp) and open (op) phases (Fig. 7b). A variety of liquid organics, including protic/nonprotic and polar/nonpolar molecules, resulted in various degrees of expansion in MIL-53(Fe).173 Increases in volume per formula unit (Vf.u.) between the closed and various open phases varied from 2 to 77% (Table 2).
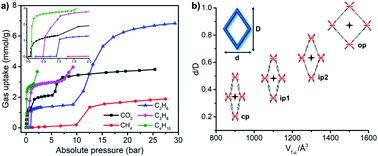 |
| Fig. 7 (a) Gas sorption isotherms recorded for MIL-53(Fe) at 303 K; (b) different phases observed in MIL-53(Fe), cp = closed phase, ip = intermediate phase, op = open phase. | |
Table 2 Guest sorption studies on MIL-53(Fe) and the corresponding volumes per formula unit of guest-loaded phasesa
Guests |
V
f.u. (Å3) |
Year |
Ref. |
py = pyridine; IPA = isopropanol; DMSO = dimethyl sulfoxide; DMF = N,N′-dimethylformamide; THF = tetrahydrofuran; DEF = N,N′-diethylformamide; EA = ethyl acetate; NB = nitrobenzene; DMC = dimethyl carbonate; MX = meta-xylene; OX = ortho-xylene; PX = para-xylene; bzta = benzothiazole; bztp = benzothiophene.
|
py |
1393 |
2005 |
158
|
Closed phase/H2O |
900/987 |
2008 |
172
|
IPA/DMSO/py/DMF/quinone/THF |
1271/1304/1395/1398/1455/1471 |
2008 |
173
|
CHCl3/DEF/EA/NB/toluene/EtOH |
1534/1534/1566/1581/1582/1585 |
DMC/MeOH/BuOH/lutidine/MeCN/MX |
1588/1591/1592/1593/1594/1587 |
Ibuprofen |
1407 |
2008 |
174
|
CH4 |
964 |
2009 |
106
|
C2H6 (three phases) |
984/1186/1587 |
C3H8 (three phases) |
1001/1272/1565 |
C4H10 (three phases) |
1014/1311/1566 |
Lutidine/py/H2O |
1213/1398/1000 |
2010 |
175
|
MeOH (two phases) |
1192/1595 |
2011 |
176
|
EtOH (two phases) |
1199/1580 |
1-PrOH (two phases) |
1243/1511 |
2-PrOH |
1271 |
OX/MX/PX |
1579/1584/1582 |
2012 |
177
|
bzta/bztp |
1590/1591 |
2013 |
178
|
CO2 (three phases) |
917/1083/1563 |
2015 |
107
|
Ligand functionalization impacts the flexibility of MIL-53(Fe) with various adsorbates (Table 3) as exemplified by MIL-53(Fe)-X variants prepared from a library of functionalised bdc ligands (Fig. 8a).179–183 Except for MIL-53(Fe)-2OH, the activated “dry” phases of MIL-53(Fe)-X exhibited larger volumes than MIL-53(Fe), consistent with the ip1 or ip2 phase of MIL-53(Fe) (Fig. 8b, black line).181 77 K N2 sorption studies revealed no porosity (closed phases) except for MIL-53(Fe)-2CF3. The larger pore volumes of activated MIL-53(Fe)-X phases were attributed to steric hindrance between functional groups which prevent pore closure. Hydration was found to result in only negligible changes to the unit-cell volumes (Fig. 8b, red line). MIL-53(Fe)-2OH is an exception as it was found to exhibit high water uptake thanks to hydrogen bonding between hydroquinolic OH groups and water molecules. Regarding other solvents, MIL-53(Fe)-2COOH was observed to be an outlier. It remained in its closed phase regardless of the liquid used and exhibited no significant solvent uptake. This outcome was attributed to strong intraframework hydrogen bonding interactions that drive the pores to remain closed. In general, pore opening in functionalised MIL-53(Fe)-X variants is governed by a balance between the intrinsic stability of the closed and open phases and guest–framework interactions. The influence of the linker on the switching of MIL-53(Fe) upon CO2 and C1–C9 hydrocarbon adsorption was also systematically studied (Fig. 8c).182,183 With the exception of methane, closed to open transformations occurred through an intermediate phase (X= Cl, Br, CH3), thus differing from the parent, MIL-53(Fe), for which two intermediate phases were observed (Fig. 8b). MIL-53(Fe)-2COOH and MIL-53(Fe)-NH2 remained closed when exposed to CO2 or hydrocarbons.182,183 A combination of steric effects and intraframework interactions in MIL-53(Fe)-X was deemed responsible for these observations.
Table 3 Chronology of MIL-53(Fe)-X variants
MIL-53(Fe)-X |
Guests |
Year |
Ref. |
2COOH |
H2O |
2004 |
179
|
NH2 |
H2BDC-NH2 |
2008 |
180
|
Cl, Br, NH2, CH3, 2OH, 2CF3, 2COOH |
H2O, pyridine, EtOH, tetrachloroethane |
2010 |
181
|
Cl, Br, NH2, CH3 |
CH4, C2H6, C3H8, C4H10, C6H14, C7H16, C8H18, C9H20 |
2011 |
182
|
Cl, Br, NH2, CH3, 2COOH |
CO2 |
2012 |
183
|
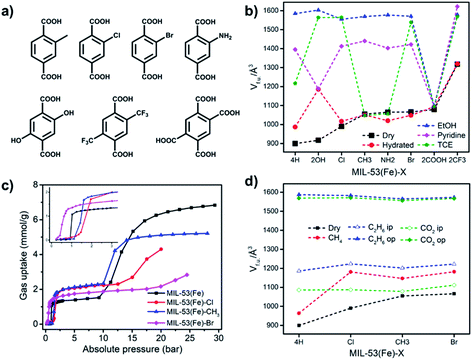 |
| Fig. 8 (a) The functionalised bdc ligands used to synthesise MIL-53(Fe)-X variants; (b) and (d) volume per formula unit (Vf.u.) of MIL-53(Fe)-X variants under different conditions, 4H = MIL-53(Fe); (c) C2H6 sorption isotherms of MIL-53(Fe)-X at 303 K. | |
2.1.2 Pillared-layer CNs.
Pillared-layer coordination networks (PLCNs) are a diverse class of CNs that have been extensively studied in the past two decades.6,184–188 Most PLCNs feature pcu or “jungle-gym” geometry and the most commonly studied subset has the general formula [M2L2P]n (M = divalent metal cation; class I: L = dicarboxylate linker and P = N-donor pillar; class II: L = N-donor linker and P = inorganic anionic pillar).188 Class I PLCNs comprise two-dimensional sql topology networks linked by dicarboxylate linker ligands (L) which are pillared in the third dimension by neutral N-donor linker ligands (P) to form pcu topology frameworks (Fig. 9). The metal nodes of the prototypal PLCNs are dinuclear paddle-wheel [M2(COO)4] that serve as 6-connected (6c) MBBs.
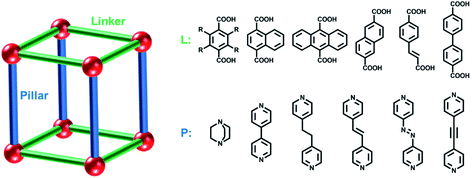 |
| Fig. 9 Schematic illustration of pillared-layer coordination networks with examples of linker (L) and pillar ligands (P). | |
The prototypal PLCN, [Zn2(bdc)2(dabco)]n (dabco = 1,4-diazabicyclo[2.2.2]octane), is widely known as DMOF-1. The bdc-linked sql nets are comprised of [Zn2(COO)4] paddle-wheel moieties that are pillared by dabco ligands to form the resulting pcu framework.189–192 Sorption studies using N2 and H2 on DMOF-1 revealed type I isotherms, an indication of structural rigidity.189 However, benzene was found to induce structural deformation of DMOF-1 from square to rhombic grids. Interestingly, sorption of IPA, MeOH and EtOH on DMOF-1 afforded two-step type F-I isotherms.190,191 The first step resulted from contraction whereas the second step corresponded to pore reopening. Molecular simulations have provided insight into these structural changes.192
The ready availability/accessibility of numerous dicarboxylic acids and dipyridyl ligands has enabled facile linker and pillar functionalization/substitution of DMOF-1 to create families of related pcu structures. In addition, Zn(II) can be replaced by several other transition metals. Studies on the sorption properties of DMOF-1 derivatives have revealed profound effects upon sorption profiles.51,71,93,97–99,113–121,146,147 For example, the DMOF-1 analogue [Ni2(ndc)2(dabco)]n (ndc = 1,4-naphthalenedicarboxylate), DUT-8(Ni), was reported to exhibit large and reversible expansion/shrinkage between its closed and open phases.113–117 Introduced by Kaskel’s group in 2010, DUT-8(Ni) is isostructural to DMOF-1, the main difference being the length of linker ligands. The longer ndc linker in DUT-8(Ni) not only leads to larger pores and higher sorption uptakes, but results in single-step type F-IVs isotherms induced by N2, Xe, CO2 and n-butane.113 As-synthesised DUT-8(Ni) transforms to its closed phase during desolvation accompanied by a colour change from green to yellow. Compared to its open phase, the paddle-wheel units in the closed phase are strongly distorted (Fig. 10a and b) and the N–Ni–Ni–N atoms of paddle-wheel units and dabco molecules are arranged in zigzag rather than linear fashion (Fig. 10c and d).115 Induced by guest sorption, the structural transformation from closed (Vf.u. = 648 Å3) to open (Vf.u. = 1644 Å3) phases involves >150% volume expansion without covalent bond breakage (Fig. 10e and f), one of the largest volume changes reported for flexible CNs. This large expansion enables exceptionally high N2 (∼700 cm3 g−1) and CO2 (∼600 cm3 g−1) uptakes (Fig. 10g).
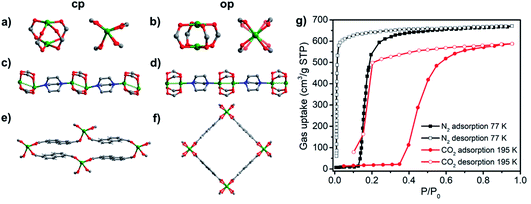 |
| Fig. 10 Comparison of the closed (a, c, e) and open (b, d, f) phases of DUT-8(Ni); (g) N2 (77 K) and CO2 (195 K) sorption isotherms of DUT-8(Ni). | |
Kaskel’s group subsequently reported that other metals can sustain DUT-8(M) analogues (M = Co, Zn, Cu) and exhibit distinct sorption profiles.114 Depending on the metal ion used, DUT-8 variants were found to exhibit reversible (DUT-8(Ni), DUT-8(Co)), irreversible (DUT-8(Zn)) or no (DUT-8(Cu)) transformation upon activation and/or guest sorption. It was noted that the particle size of DUT-8(Ni) can influence sorption profiles, which were either type F-IVs (particle size > 1 μm) or type I (particle size < 500 nm).116,193,194 A similar “downsizing” effect was also reported for the DMOF-1 analogue [Cu2(bdc)2(bpy)].93
Recently, our group reported the structures and sorption properties of a series of DMOF-1 variants, namely [Zn2(DMTDC)2(P)], X-pcu-n-Zn (n = 5, P = 1,2-di(4-pyridyl)-ethylene (dpe); n = 6, P = 1,2-bis(4-pyridyl)ethane (bpe); n = 7, P = 1,2-bis(4-pyridyl)acetylene (bpa); n = 8, P = 4,4′-azopyridine (apy); H2DMTDC = 3,4-dimethylthieno[2,3-b]thiophene-2,5-dicarboxylic acid).146,147 The four pillar ligands used are longer than dabco and enable 2-fold interpenetration in the X-pcu-n-Zn family (Fig. 11a). The as-synthesised “open” phases, X-pcu-n-Zn-α, were obtained by solvothermal synthesis with solvent molecules occupying voids and calculated guest-accessible volumes of ca. 45% (Fig. 11b). Single-crystal X-ray diffraction (SCXRD) studies revealed that activation of X-pcu-n-Zn-α resulted in “closed” nonporous phases, X-pcu-n-Zn-β, with unit cell volumes reduced by ca. 35% (Fig. 11c). CO2, C2H2 and C2H4 sorption on X-pcu-n-Zn-β revealed switching behaviour with comparable uptakes but different switching pressures (Fig. 11d–f). Up to 250 cm3 g−1 CO2 uptake and good recyclability (>35 sorption cycles) make X-pcu-n-Zn only the second family of flexible CNs to exhibit both high uptake and reversibility. The switching pressures for the three gases studied followed a consistent trend: X-pcu-6-Zn-β < X-pcu-5-Zn-β < X-pcu-7-Zn-β < X-pcu-8-Zn-β which was attributed to the relative degree of conformational flexibility of the pillar ligands.147
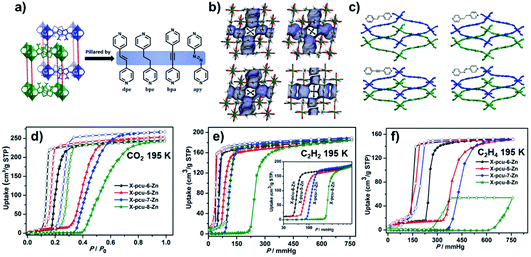 |
| Fig. 11 (a) The two-fold interpenetrated structures of X-pcu-n-Zn; (b) as-synthesised “open” phases, X-pcu-n-Zn-α; (c) activated “closed” phases, X-pcu-n-Zn-β; (d–f) CO2, C2H2 and C2H4 sorption isotherms on X-pcu-n-Zn-β at 195 K. Reproduced from ref. 147 with permission; copyright: 2019, John Wiley & Sons, Inc. | |
By exploring longer linkers and extended pillar ligands, other interpenetrated derivatives of DMOF-1 have been obtained. For example, with 4,4′-biphenyldicarboxylate as the linker and 1,4-bis(4-pyridyl)benzene or 4,4′-bis(4-pyridyl)biphenyl as the pillar, 3-fold interpenetrated CNs X-pcu-3-Zn and X-pcu-1-Zn were synthesised, respectively.73,195 Although interpenetration inevitably reduces guest-accessible voids, X-pcu-3-Zn and X-pcu-1-Zn nevertheless exhibited ca. 45% guest-accessible volume. Both PLCNs were found to exhibit complicated structural transformations through solvent exchange, heating, vacuum and gas sorption processes. Interestingly, a rare example of “shape memory” was observed for X-pcu-3-Zn and X-pcu-1-Zn, which exhibited irreversible phase transitions. The only other reported example of this phenomenon was for [Cu2(bdc)2(bpy)].93
A second diverse class of PLCNs is exemplified by the SIFSIX-n-M family of general formula [M(L)2(SiF6)] (M = divalent transition metal ions, L = N-donor ditopic ligands).6 This family is diverse in composition since the SIFSIX pillar can be replaced by other fluorinated divalent anions such as TiF62−, GeF62− and SnF62− and there are numerous N-donor linkers available. A difference between the SIFSIX-n-M and DMOF-1 families is that N-donor ligands act as linkers in the former whereas they serve as pillars in the latter. Cationic sql layers in the SIFSIX-n-M family are charge balanced by anionic pillars. The SIFSIX-n-M family is of particular interest because several members have been reported to exhibit benchmark separation performances towards a variety of gas mixtures.196,197 Whereas SIFSIX-n-M sorbents generally remain rigid during sorption cycles, rotation of linkers can cause inflections in gas adsorption isotherms.198,199 Recently, our group introduced the first switching SIFSIX-n-M PLCN, [Cu(L)2(SiF6)] (SIFSIX-23-Cu, L = 1,4-bis(1-imidazolyl)benzene), which was found to exhibit dramatic structural distortions.151 Unlike previous SIFSIX-n-M sorbents, the SIFSIX pillars in SIFSIX-23-Cu adopt a cis-bridging mode (Fig. 12a and b) and the sql layers undulate thanks to the “V”-shaped L(syn) linker ligands (Fig. 12c). Desolvation induces SIFSIX-23-Cu to undergo structural transformations involving multiple intermediate phases (SIFSIX-23-Cu-γ1, -γ2, -γ3) prior to forming a solvent-free closed phase, SIFSIX-23-Cu-β1 (Fig. 12d). N2 and CO2 sorption on SIFSIX-23-Cu-β1 reveal type F-IVs and type F-IVm isotherms, respectively (Fig. 12e). SIFSIX-23-Cu is one of the few switching CNs that exhibits >200 cm3 g−1 gas uptake and good recyclability (>40 sorption cycles). SIFSIX-23-Cu was also found to be stable in water for at least one year.
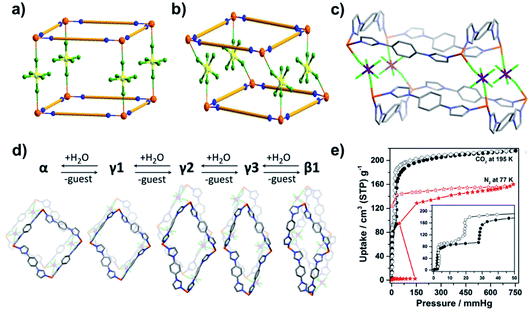 |
| Fig. 12 Schematic diagrams of (a) traditional SIFSIX-n-M networks with trans-bridging SIFSIX pillars and (b) SIFSIX-23-Cu with cis-bridging SIFSIX pillars; (c) coordination mode and geometry in SIFSIX-23-Cu; (d) reversible structural transformations in SIFSIX-23-Cu; (e) N2 (77 K) and CO2 (195 K) sorption isotherms of SIFSIX-23-Cu. Reproduced from ref. 151 with permission; copyright: 2020, American Chemical Society. | |
2.1.3 Diamondoid CNs.
Diamondoid CNs feature dia topology and are amongst the earliest and most comprehensively studied CNs. They are also highly amenable to crystal engineering.13,14,200,201 Interpenetration in diamondoid CNs tends to reduce surface area but can enhance rigidity.202 Nevertheless, structural flexibility was observed in the 2-fold interpenetrated diamondoid CN [In(ABDC)2] (SHF-61, ABDC = 2-aminobenzene-1,4-dicarboxylate) with continuous breathing/swelling behaviour during desolvation/solvation.203 This is a rare phenomenon that was previously observed in MIL-88 CNs.56,57 The 8-fold interpenetrated diamondoid CN [Zn(oba)(pip)] (JUK-8), which is based on 4,4′-oxybis(benzenedicarboxylate) (oba) and 4-pyridyl functionalised benzene-1,3-dicarbohydrazide (pip) linkers, exhibited switching upon exposure to H2O and CO2.154
Recently, our group reported a flexible diamondoid CN [NiL2], X-dia-1-Ni, which is based upon a mixed N/O-donor ligand, 4-(4-pyridyl)-biphenyl-4-carboxylic acid (HL).67 Despite 6-fold interpenetration, the accessible void volume in X-dia-1-Ni is 49% thanks to the mode of interpenetration and the rectangular channels sustained by ligand L (Fig. 13a–d). X-dia-1-Ni was observed to undergo single-crystal-to-single-crystal (SCSC) transformations through solvent exchange with CH2Cl2 and transform to its closed phase X-dia-1-Ni-c1 by heating under vacuum (Fig. 13e). 77 K N2 sorption indicated that X-dia-1-Ni-c1 is nonporous whereas 195 K CO2 sorption revealed multiple steps in both the adsorption and desorption branches. These profiles indicate reversible structural changes between closed and open phases. Interestingly, the CH4 adsorption isotherm measured at 298 K (Fig. 13f) revealed a type F-IVs isotherm that combined an appropriate switching pressure (5–35 bar) and high saturation uptake (>200 cm3 g−1). These metrics made X-dia-1-Ni only the second flexible CN after Co(bdp) to exhibit such a high working capacity for CH4. Co(bdp) is discussed in the following section.
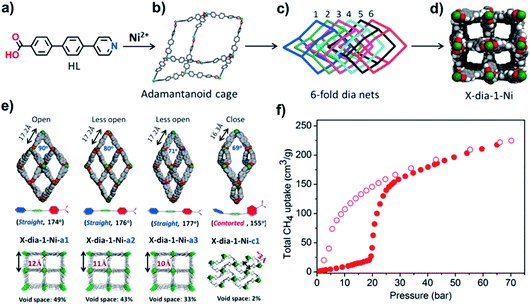 |
| Fig. 13 (a) Structure of 4-(4-pyridyl)-biphenyl-4-carboxylic acid (HL); (b) adamantanoid cage in X-dia-1-Ni; (c) 6-fold interpenetrated dia nets in X-dia-1-Ni; (d) rectangular channels of X-dia-1-Ni viewed along the c-axis; (e) single crystal structures of the porous (a1, a2, a3) and nonporous (c1) phases of X-dia-1-Ni; (f) high pressure CH4 sorption isotherm of X-dia-1-Ni at 298 K. Reproduced from ref. 67 with permission; copyright: 2018, John Wiley & Sons, Inc. | |
2.1.4 M(bdp) family.
Switching CNs usually exhibit only two phases (e.g. one open phase and one closed phase) and their structural transformations are typically induced by polar gases such as CO2 and C2H2 or polar solvents. Multiple phase transitions induced by nonpolar molecules such as H2 and N2 are somewhat rare. Long et al. reported that a 3D switching CN Co(bdp) (Fig. 14a, bdp = 1,4-benzenedipyrazolate),74,101–103 exhibits an unusual five-step N2 sorption isotherm at 77 K (Fig. 14b). A combination of in situ PXRD and molecular simulation studies revealed that the activated “closed” phase, Co(bdp)-dry, transformed to its fully “open” phase via three distinct intermediate phases (Fig. 14c). In addition, H2 sorption on Co(bdp)-dry revealed a phase transformation which was not seen in other switching CNs.101 Most importantly, Co(bdp)-dry exhibits a stepped type F-IVs isotherm when exposed to CH4 (Fig. 14d).74 The gate adsorption and desorption pressures are ca. 15 and 5 bar, respectively, suitable for CH4 storage and delivery in the context of vehicular transport (5–35 bar). High uptake and the right type of F-IV isotherm profile made Co(bdp) the benchmark for CH4 working capacity (ca. 200 cm3 cm−3). The Fe analogue, Fe(bdp), was also found to exhibit a type F-IVs isotherm but with a much higher switching pressure, highlighting the profound impact that metal centers can exert on switching pressures. In contrast, the Zn and Ni analogues, Zn(bdp) and Ni(bdp), were only found to exhibit type I gas sorption isotherms.204 Furthermore, ligand functionalization in Co(bdp) can be used to tune the switching pressures.133
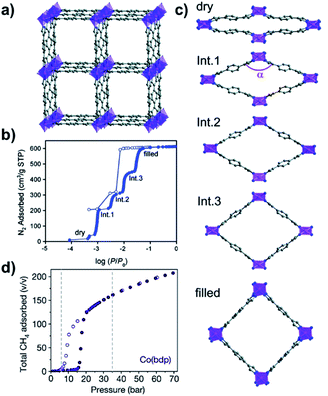 |
| Fig. 14 (a) Crystal structure of as-synthesised Co(bdp); (b) N2 sorption isotherms of Co(bdp)-dry at 77 K; (c) phase transition during N2 sorption; and (d) CH4 sorption isotherms of Co(bdp)-dry at 298 K. Reproduced from ref. 74 and 102 with permission; copyrights: 2015, Springer Nature and 2010, American Chemical Society. | |
2.2. Examples of 2D switching CNs
2.2.1 Square lattice (sql) CNs.
2D CNs may exhibit switching through clay-like intercalation. One would intuitively anticipate that such a switching mechanism is facile because the attractive forces between layers are relatively weak and little strain upon the CN is likely to be required. Square lattice (sql) CNs are quite prevalent and account for nearly half of reported 2D CNs.205 A well-studied family of sql CNs is comprised of octahedral metal ions (M), axial counter anions (A) and linear linker ligands (L).206 When M
:
L is 1
:
2, sql CNs of general formula [M(L)2(A)2]·xguest can be formed (Fig. 15).207–209 From a crystal engineering perspective, this family of CNs exemplify the “node and linker” strategy first developed by Robson and Hoskins over thirty years ago.13,14 An emphasis upon design and structural characterization of sql CNs preceded sorption studies until the ELM (elastic layer-structured MOF) family was investigated.28,59,72,85–90
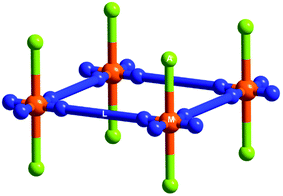 |
| Fig. 15 A schematic illustration of 2D sql CNs. Orange balls: octahedral metal ions (M), lime balls: axial counter anions (A), blue rods: linear linker ligands (L). | |
The first reported sorption study on sql CNs was conducted upon [Cu(bpy)2(BF4)2], ELM-11.59 In 2001, Kaneko et al. first studied the 1D linear chain CP [Cu(bpy)(BF4)2(H2O)2·bpy] (Fig. 16a)28 and its activated form, ELM-11, was observed to exhibit phase switching when exposed to N2, Ar and CO2. To our knowledge, this is the first example of type F-IV sorption isotherms in switching CNs. This behaviour was initially attributed to hydrogen bond regulation but there was no structural information concerning the activated form.28 It was not until 2006 that the structure of the activated form was determined by synchrotron PXRD to be the sql topology CN [Cu(bpy)2(BF4)2] (Fig. 16b).88 It was later found that ELM-11 underwent a multi-step rather than single-step phase transition induced by CO2 at 1 bar when the temperature was decreased from 273 to 195 K (Fig. 16c),86,87 revealing a type F-IVm isotherm. The mechanism of the switching behaviour was reinterpreted as expansion/shrinkage between adjacent layers triggered by inclusion of CO2 molecules (Fig. 16d).
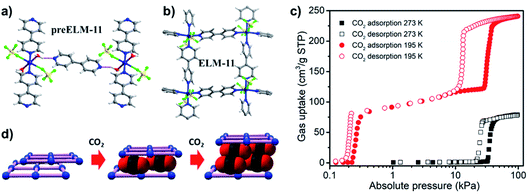 |
| Fig. 16 The structures of (a) preELM-11 and (b) ELM-11; (c) CO2 sorption isotherms of ELM-11 at 195 and 273 K; and (d) illustration of expansion/shrinkage of the adjacent layers in ELM-11. Figure (d) is reproduced from ref. 86 with permission; copyright: 2016, American Chemical Society. | |
Substitution of the metal cations or counter anions afforded several ELM-11 analogues,59,89 including [Cu(bpy)2(OTf)2] (ELM-12),70 [Cu(bpy)2(BF3CF3)2] (ELM-13),153 and [Ni(bpy)2(BF4)2] (ELM-31).104 ELM-12 exhibited an open phase after guest removal, possibly because of the “pillaring” role of the OTf− anions. With respect to N2 sorption, ELM-12 exhibited a two-step type F-II isotherm distinct from that observed in ELM-11. On the other hand, both ELM-13 and ELM-31 were observed to exhibit type F-IV isotherms like ELM-11 but with different uptakes and switching pressures.
Recently, our group studied the sorption properties of the previously known sql CN [Co(bpy)2(NCS)2],208 sql-1-Co-NCS,144,145 which is isostructural to the ELM family. sql-1-Co-NCS is sustained by Co(II) ions coordinated at equatorial coordination sites to bpy linker ligands with terminal NCS− anions occupying the axial positions. Whereas the N2 uptake of sql-1-Co-NCS at 77 K was found to be negligible (Fig. 17a), the 195 K CO2 isotherm was observed to exhibit a type F-IVs isotherm.144 Such an isotherm is consistent with the switching behaviour seen in ELM-11. High-pressure CO2 sorption isotherms of sql-1-Co-NCS were recorded at different temperatures and the relationship between temperature and switching pressure was found to obey the Clausius–Clapeyron equation. In addition, recyclability tests revealed that sql-1-Co-NCS exhibits good recyclability and fast kinetics. C8 aromatic vapour sorption isotherms were also collected on sql-1-Co-NCS at 298 K (Fig. 17b).145 It was observed that sql-1-Co-NCS switches in the presence of each of the C8 aromatic isomers but with marked differences in terms of adsorption capacity and switching pressure. Single-crystal and powder XRD studies revealed that the interlayer distance in the closed phase (4.5 Å) increased to 5.4 Å (CO2 loaded phase) and to 9.2 Å (xylene loaded phase) (Fig. 17c–e), the largest interlayer separation yet reported for bpy-based sql CNs.
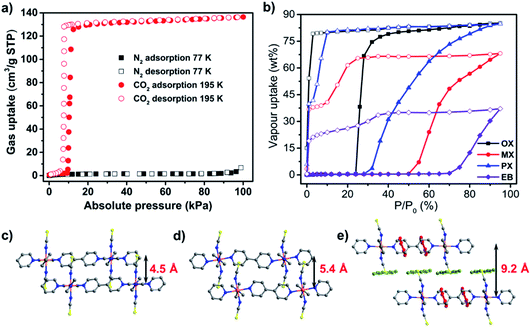 |
| Fig. 17 (a and b) N2 (77 K), CO2 (195 K) and C8 vapour (298 K) sorption isotherms for sql-1-Co-NCS; (c–e) layer packing of nonporous, CO2-loaded and xylene loaded phases of sql-1-Co-NCS, respectively. | |
2.2.2 Other examples of switching 2D CNs.
Kitagawa and co-workers documented several examples of 2D interdigitated layered CNs and studied their switching properties.65,66 [Cu(dhbc)2(bpy)] was prepared from copper nitrate, 2,5-dihydroxybenzoic acid (Hdhbc) and 4,4′-bipyridine (bpy). Cu(II) ions are linked by bpy linkers to generate chains that are further linked by two carboxyl groups of dhbc to afford a 2D layer motif.65 These layers are mutually interdigitated by the phenolic group of dhbc to generate 1D channels (Fig. 18a). Despite no change to the Cu(II) coordination environment after activation, the dhbc ligands tilt significantly and transform from cis-mode to trans-mode arrangement which results in layer shrinkage (Fig. 18b).94 High pressure N2, O2, CH4 and CO2 sorption studies demonstrated that the activated phase of [Cu(dhbc)2(bpy)] exhibits a switching effect with type F-IVs isotherms (Fig. 18c). This study was the first report of a stepped sorption in switching CNs at ambient temperature and high pressure. Adsorbate-dependent switching pressures were observed at 50, 35 and 0.4 bar for N2, O2 and CO2, respectively.
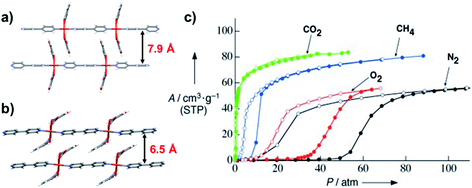 |
| Fig. 18 Crystal structures of (a) open and (b) closed phases of [Cu(dhbc)2(bpy)] and (c) high pressure gas sorption isotherms of its activated phase at 298 K. Figure (c) is reproduced from ref. 65 with permission; copyright: 2003, John Wiley & Sons, Inc. | |
In 2008, Kitagawa’s group introduced another 2D interdigitated CN, [Cd(bpndc)(bpy)] (bpndc = benzophenone-4,4′-dicarboxylate).66 SCXRD studies revealed that Cd(II) nodes are linked by bpndc ligands to produce 1D double-chain structures of [Cd(bpndc)] (Fig. 19a), which are in turn linked by bpy to afford a 2D bilayer motif (Fig. 19b). As-synthesised [Cd(bpndc)(bpy)] underwent a significant contraction through activation to form its closed phase (Fig. 19c). The sorption isotherms at 90 K revealed type F-IVs isotherms with different switching pressures for O2, Ar and N2 of 3.9, 40.1 and 55.3 kPa (Fig. 19d), respectively.
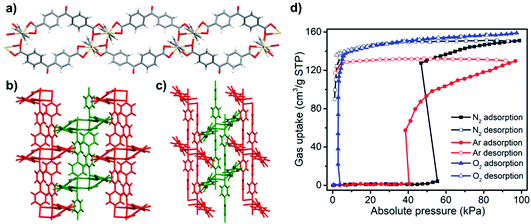 |
| Fig. 19 (a) 1D double chain structure of [Cd(bpndc)]; (b and c) 2D bilayer structure of as-synthesised and activated forms of [Cd(bpndc)(bpy)]; (d) O2, N2 and Ar sorption isotherms recorded on activated [Cd(bpndc)(bpy)] at 90 K. | |
2.3. Factors that impact switching between phases
Various factors can influence the flexibility of switching CNs. With respect to extrinsic factors, temperature, pressure and the nature of the adsorbate all impact switching events. Since switching between the closed and open phases is generally a thermodynamic event, low temperature and high pressure tend to promote more facile switching. To this end, in order to screen for switching it is recommended that sorption isotherms are collected at the boiling point of the adsorbate (e.g. 77 K for N2 or 195 K for CO2). High-pressure gas sorption might be necessary at ambient temperatures. For example, at 298 K and 1 bar, sql-1-Co-NCS does not exhibit switching upon exposure to CO2. Rather, switching requires either decreasing the temperature to 195 K or increasing pressure to around 30 bar.144 The adsorbate is also a key factor since adsorbate–adsorbent interactions play a role in triggering the phase transformations.106 In general, nonpolar gases such as H2, Ar, O2 and N2 tend to exhibit relatively weak interactions with most CNs. Hydrocarbons with unsaturated bonds may favour host–guest interactions with decreasing affinity as follows: aromatics > alkynes > alkenes > alkanes. The number of carbon atoms of hydrocarbon adsorbates also influences switching since more carbon atoms generally results in stronger host–guest interactions. However, this empirical rule-of-thumb is limited by the need for suitable pore size and shape in the open phase of switching CNs.
With respect to intrinsic factors, the composition of a CN is key to enabling and controlling phase transformations including switching. Ligand functionalization has been established as an effective route to fine-tune switching pressure as demonstrated with Co(bdp)-X133 and MIL-53(Fe)-X.182,183 Pillar ligand substitution of X-pcu-n-Zn PLCNs was also effective.146,147 However, linker ligand substitution in PLCNs (e.g. DUT-8(Ni)113,114vs. DMOF-1 (ref. 189–191)) can stop switching. This can also occur with metal-node substitution. For three well-studied families of switching CNs, MIL-53(M), DUT-8(M) and M(dpd), switching was profoundly affected by metal node substitution. Only specific metal nodes, i.e., MIL-53(Fe/Sc), DUT-8(Ni) and Co/Fe(dpd), were observed to exhibit switching behaviour. Particle size can also impact switching behaviour but remains largely understudied.140,194
3. Switching mechanisms
Although switching CNs generally exhibit type F-IV sorption isotherms and may share structural features, switching mechanisms can vary. Most switching CNs crystallize as solvated (open) phases and transform to their desolvated (closed) phases during solvent removal/activation. For some CNs this is the full scope of phases and switching occurs just between the as-synthesized (open) and activated (closed) phases. For other CNs, one or more metastable states (intermediate phases) that are partially open can exist in addition to the closed and fully open phases. To elucidate switching mechanisms we herein analyse the closed and open phases of switching CNs that are structurally characterized (usually by SCXRD). At the molecular level, switching mechanisms can be driven by intra-network distortions, inter-network interactions or most likely a combination thereof (Fig. 20 and Table 4).38–41 Intra-network distortions include ligand motions (Fig. 20a: bending, twisting and rotation), metal node coordination sphere changes (Fig. 20b: deformation and reconstitution/isomerism) and overall intra-network motions (Fig. 20c: edge bending/straightening and net shearing). Inter-network motions include subnetwork displacements such as interpenetrated net sliding (Fig. 20d) and layered net expansion (Fig. 20e).
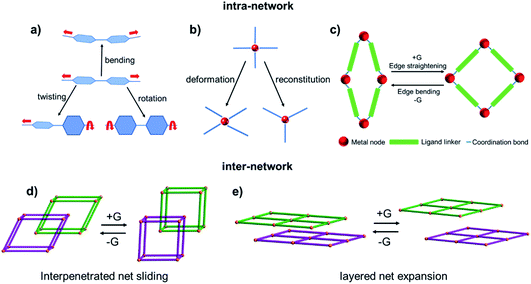 |
| Fig. 20 Schematic of switching mechanisms driven by intra-network and inter-network changes: (a) ligand motion; (b) metal node motion; (c) overall intra-network motion; (d) interpenetrated net sliding and (e) layered net expansion. | |
Table 4 Switching mechanisms of representative switching CNsa
Switching CNs |
Switching mechanisms |
Ref. |
Intra-network |
Inter-network |
Metal node |
Linker ligand |
Subnet |
A |
B |
C |
D |
E |
F |
G |
A: deformation; B: reconstitution; C: rotation; D: bending; E: twisting; F: interpenetrated net sliding; G: layered net expansion.
|
MIL-53(Fe) |
✗ |
✗ |
✓ |
✗ |
✗ |
✗ |
✗ |
106
|
MOF-508 |
✓ |
✗ |
✓ |
✗ |
✗ |
✓ |
✗ |
97 and 99 |
DUT-8(Ni) |
✓ |
✗ |
✓ |
✗ |
✗ |
✗ |
✗ |
115
|
[Cu2(bdc)2(bpy)] |
✓ |
✗ |
✓ |
✗ |
✓ |
✓ |
✗ |
93
|
[Zn2(tp)2(dfbpb)] |
✗ |
✓ |
✓ |
✓ |
✓ |
✓ |
✗ |
120
|
X-pcu-n-Zn (n = 5–8) |
✗ |
✗ |
✓ |
✗ |
✓ |
✓ |
✗ |
146 and 147 |
X-dia-1-Ni |
✗ |
✗ |
✗ |
✓ |
✓ |
✓ |
✗ |
67
|
Co(bdp) |
✗ |
✗ |
✓ |
✗ |
✓ |
✗ |
✗ |
102
|
ELM-11 |
✗ |
✗ |
✓ |
✗ |
✓ |
✗ |
✓ |
89
|
sql-1-Co-NCS |
✗ |
✗ |
✓ |
✗ |
✓ |
✗ |
✓ |
144 and 145 |
[Cu(dhbc)2(bpy)] |
✗ |
✗ |
✓ |
✗ |
✓ |
✗ |
✓ |
65 and 94 |
[Cu(pyrdc)(bpp)] |
✗ |
✓ |
✗ |
✓ |
✗ |
✗ |
✓ |
96
|
[Cd(bpndc)(bpy)] |
✗ |
✓ |
✗ |
✗ |
✗ |
✗ |
✓ |
66
|
SIFSIX-23-Cu |
✗ |
✗ |
✓ |
✓ |
✓ |
✗ |
✗ |
151
|
Ligand motion is often observed, not just in flexible CNs, but also in rigid CNs.210–212 When ligand motion occurs in switching CNs it tends to occur over a relatively large amplitude. Compositional analysis of switching CNs reveals that the most commonly used organic ligands are the linear linkers bpy and bdc, which occur in ca. 25% of switching CNs. The N-donor linker bpy can rotate and twist even without ligand bending, as exemplified by sql CNs such as sql-1-Co-NCS (Fig. 21a).144,145 The O-donor ligand bdc rotates, as exemplified by MIL-53(Fe) (Fig. 21b and 7b),106 and bending behaviour was reported for DMOF-1.189 Ligand rotation is a feature of functionalised MIL-53(Fe) analogues, MIL-53(Fe)-X, whereby the functional groups X in the closed phases project towards the channels and prevent full contraction.181 Ligand rotation and twisting was also reported for Co(bdp) (Fig. 21c),74 whereas more extreme ligand bending that could be described as contortion was seen for longer ligands such as pbpc in X-dia-1-Ni (Fig. 13e).67
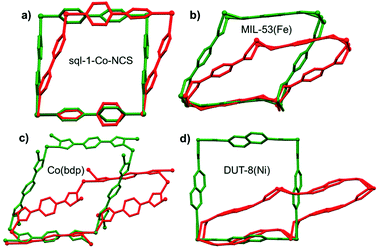 |
| Fig. 21 Overlay comparison of closed (red) and open (green) phases of four switching CNs: (a) sql-1-Co-NCS; (b) MIL-53(Fe); (c) Co(bdp) and (d) DUT-8(Ni). | |
Metal node motion does not always occur in switching CNs. For example, the coordination environments of metal nodes in sql-1-Co-NCS (octahedral geometry), MIL-53(Fe) (octahedral geometry) and Co(bdp) (tetrahedral geometry) were unchanged in their closed and open phases. Conversely, metal node deformation was observed in the [Zn2(COO)4] and [Ni2(COO)4] paddle-wheel units of MOF-508 (ref. 97 and 99) and DUT-8(Ni),115 respectively (Fig. 10a). Metal node isomerism involving coordination bond breakage remains relatively rare but plays an important role in some switching CNs, e.g. [Cu(pyrdc)(bpp)], [Zn2(bdc)2(dfbpb)] and [Co(VTTF)].96,120,138
Intra-network motion can often be attributed to cooperative ligand and metal node motions, e.g. net shearing and edge bending/strengthening (hinge motion) that arise thanks to nonlinear coordination modes between metal ions and organic ligands. Overall, our analysis indicates that intra-network motions are quite common in switching CNs that feature square or rhombic cavities (Fig. 21). This is presumably attributable to the rhombus being a basic flexible building block of a “truss network”, as observed even at the macro scale.213 For example, at the macro scale, a common use of “rhombus deformation” in a chemistry lab would be the lab jack. Integration of square or rhombic units into a CN is likely to be a viable crystal engineering strategy to design new switching CNs from first principles.
Subnetwork displacement is a phenomenon restricted to CNs that comprise individual subnets that interact with each other by weak forces, e.g. hydrogen bonding or van der Waals interactions. This includes interpenetrated net sliding as well as layered net expansion in 3D and 2D switching CNs, respectively. Interpenetrated net sliding usually occurs between centered interpenetrated nets (closed phase) and offset interpenetrated nets (open phase), thereby creating voids to accommodate guest molecules as exemplified by 2-fold interpenetrated PLCNs such as the X-pcu-n-Zn family.146,147 Layered net expansion occurs in 2D switching CNs thanks to their ability to offer clay-like intercalation of guest molecules as observed in switching sql CNs.144,145
A question that remains to be answered is why do isostructural CNs exhibit very different structural flexibility and sorption behaviour, e.g. MIL-47 does not exhibit breathing behaviour whereas MIL-53 does?214,215 Addressing this matter requires a quantitative understanding of the energy landscape including the energy barriers between multiple phases and the energy derived from host–guest and guest–guest interactions.216–218 In addition, kinetic factors such as linker rotation dynamics can affect the framework transition, especially across transient phases.219 In many cases, the precise mechanisms of phase transformations are beyond the scope of SCXRD, which only provides the static perspective of crystal structures. Further mechanistic insight will likely come through in situ experiments coupled with molecular modeling studies.
4. Applications
4.1. Gas storage
Gas storage is one of the most important and well-studied properties of porous CNs.220–223 Indeed, we are in the “age of gas”224 and physisorption is recognised as an energy-efficient approach to mitigate the energy footprint associated with traditional compression and liquefaction-based technologies. A variety of gases such as non-polar gases (H2, O2, N2, Ar, Xe), polar gases (CO, NO, CO2) and C2–C4 light hydrocarbon gases and vapours have been investigated in rigid porous CNs.220–223 77 K N2 sorption serves as the most common characterisation tool to experimentally determine the porosity of an adsorbent. The greenhouse gas CO2 is another extensively studied gas at low (e.g. 195 K and 1 bar) and high (e.g. 298 K and 50 bar) temperatures and pressures, driven by the importance of carbon capture from flue gases (bulk) and air (trace). On the other hand, as a relatively inexpensive and clean fuel gas, CH4 is of particular interest and importance given the energy footprints and limitations of liquified and compressed nature gas storage.
Recent studies have demonstrated that switching nonporous CNs that exhibit type F-IV isotherms can enable higher working capacities than physisorbents with type I isotherms as explained above (Fig. 2f). There are several other potential advantages of switching CNs for gas storage. First, since type F-IV isotherms usually exhibit hysteresis between their adsorption and desorption branches, it allows for a gas to be adsorbed at high pressure and stored at relatively low pressure.72 Second, switching pressure and temperature follow the Clausius–Clapeyron equation,72,86,144 which means that switching pressure can be calculated for a given temperature. This enables the selection of a bespoke sorbent that offers optimised parameters for temperature or pressure swing adsorption processes or combinations thereof. Last, but not least, endothermal/exothermal structural expansion/contraction in switching CNs naturally offsets the exothermal/endothermal nature of adsorption/desorption processes, thereby facilitating improved thermal management.74,87
As detailed earlier herein, various gases have been studied to determine if they can induce switching in CNs (Table 1). 77 K N2 and 195 K CO2 sorption isotherms are well documented as summarised in Table 5. Thus far, around 20 switching CNs exhibit >100 cm3 g−1 CO2 or N2 uptake at cryogenic temperatures and their switching pressures vary from ca. 0.1 to 80 kPa (Fig. 22a and b). DUT-8(Ni) exhibits the highest N2 (670 cm3 g−1) and CO2 (590 cm3 g−1) uptakes yet reported at 77 and 195 K, respectively.114 However, at 298 K, DUT-8(Ni) adsorbs negligible N2 and only 58% of its saturation CO2 uptake (345 cm3 g−1) at 50 bar. For CH4, DUT-8(Ni) was found to exhibit a characteristic type I isotherm and adsorbed only 70 cm3 g−1 at 50 bar, 298 K. Other switching CNs such as X-pcu-n-Zn, ELM-11 and M(bdp) exhibit >200 cm3 g−1 uptakes at cryogenic temperatures. With respect to the X-pcu-n-Zn family (n = 5, 6, 7, 8), X-pcu-6-Zn exhibits the lowest switching pressures for N2 and CO2.147 At 298 K, X-pcu-n-Zn CNs exhibit negligible CO2 uptake except for the “softest” variant, X-pcu-6-Zn, which adsorbs 50 cm3 g−1 of CO2 at 33 bar. Unsurprisingly, like DUT-8(Ni), high pressure CH4 adsorption isotherms to 50 bar revealed negligible uptake for all four X-pcu-n-Zn CNs. ELM-11 exhibits a type F-IVs isotherm for N2 at 77 K and a type F-IVm isotherm for CO2 at 195 K with almost identical saturation uptakes (ca. 250 cm3 g−1).59,86,87 High pressure CO2 sorption conducted upon ELM-11 at room temperature produces the type F-IVm isotherm but with slightly lower uptakes.86 High pressure CH4 sorption on ELM-11 at 303 K revealed a type F-IVs isotherm with 84 cm3 g−1 uptake,59 close to the CO2 uptake registered at the first step. This adsorbed volume is comparable to that of active carbon fiber (ACF), but the recovery percentage (i.e., working capacity) of ELM-11 is much higher,59 thanks to its isotherm profile. Very recently, ELM-11 (ref. 72) and another switching PLCN, MOF-508,225 were studied for a different fuel gas storage application, the storage of the explosive gas acetylene, C2H2, at ambient temperature. ELM-11 exhibits a four-step type F-IVm isotherm and it can deliver 163 cm3 g−1 or 174 cm3 cm−3 of C2H2 between the second and third steps. However, the switching pressure at the third step is higher than 2 bar, beyond the safety pressure limit for pure C2H2. It is reasonable to assume that the inclusion and intercalation of C2H2 into ELM-11 will desensitize C2H2 but this matter must be verified or a porous monolith might be exploited. In the case of the MOF-508 series, a C2H2 working capacity of 106 cm3 cm−3 can be delivered under a relatively narrow pressure range (70–120 kPa). Compared to the benchmark rigid CNs which are more suited for C2H2 sequestration rather than C2H2 storage/delivery,72 these two reports underline the high upside potential of switching CNs for practical C2H2 storage.
Table 5 N2 (77 K, 1 bar), CO2 (195 K, 1 bar) and CH4 (298 K) sorption parameters for representative switching CNsa
CNs |
N2 77 K |
CO2 195 K |
CH4 298 K |
Ref. |
P
ga (kPa) |
Uptake (cm3 g−1) |
P
ga (kPa) |
Uptake (cm3 g−1) |
P
ga (bar) |
Uptake (cm3 g−1) |
N.A. = not available; Pga = gate adsorption pressure.
|
DUT-8(Ni) |
13 |
670 |
35 |
590 |
Type I |
70 |
114
|
Co(bdp) |
3 |
668 |
N.A. |
N.A. |
15 |
246 |
74
|
Fe(bdp) |
0.8 |
638 |
N.A. |
N.A. |
25 |
291 |
Co(F-bdp) |
4 |
582 |
N.A. |
N.A. |
Type F-III |
173 |
133
|
Co(o-F2-bdp) |
4 |
560 |
N.A. |
N.A. |
12 |
160 |
Co(p-F2-bdp) |
1 |
504 |
N.A. |
N.A. |
10 |
157 |
Cu(HIsa-az-dmpz) |
3 |
360 |
8 |
310 |
N.A. |
N.A. |
150
|
JLU-Liu3 |
41 |
325 |
26 (F-II) |
337 |
Negligible uptake till 1 bar |
129
|
JLU-Liu4 |
26 |
306 |
16 (F-II) |
333 |
JLU-Liu33 |
6 |
319 |
4 (F-II) |
205 |
135
|
X-dia-1-Ni |
Remain closed |
Type F-II |
325 |
20 |
225 |
67
|
X-pcu-5-Zn |
45 |
135 |
30 |
254 |
Remain closed phase up to 50 bar |
146 and 147 |
X-pcu-6-Zn |
20 |
290 |
17 |
245 |
X-pcu-7-Zn |
68 |
217 |
33 |
267 |
X-pcu-8-Zn |
Remain closed |
39 |
243 |
ELM-11 |
10 |
250 |
30 |
245 |
38 |
84 |
59 and 86 |
ELM-13 |
20 |
237 |
50 |
180 |
N.A. |
N.A. |
153
|
CPM-325 |
26 |
216 |
2.6 |
200 |
N.A. |
N.A. |
141
|
[Cu2(bdc)2(bpy)] |
0.07 |
170 |
7 |
156 |
9 |
79 |
51 and 93 |
SIFSIX-23-Cu |
19 |
160 |
3.7 |
216 |
N.A. |
N.A. |
151
|
Cd(bpndc)(bpy) |
55 |
150 |
N.A. |
N.A. |
N.A. |
N.A. |
66
|
[Zn2(tdc)2(pvq)] |
15 |
140 |
N.A. |
N.A. |
N.A. |
N.A. |
143
|
MIL-53(Sc) |
Remain closed |
75 |
285 |
N.A. |
N.A. |
123
|
Zn2(tp)2(dfbpb) |
Remain closed |
40 |
248 |
N.A. |
N.A. |
120
|
Cu(OTf)2(bpp)2 |
Remain closed |
60 |
153 |
N.A. |
N.A. |
125
|
sql-1-Co-NCS |
Remain closed |
10 |
136 |
N.A. |
N.A. |
144
|
Zn(GA)2 |
Remain closed |
5 |
132 |
N.A. |
N.A. |
111
|
Cd(mida)2 |
N.A. |
N.A. |
35 |
130 |
N.A. |
N.A. |
142
|
SNU-M11 |
Remain closed |
18 |
124 |
N.A. |
N.A. |
105
|
f-MOF-1b |
Remain closed |
45 |
107 |
N.A. |
N.A. |
132
|
Cu(pyrdc)(bpp) |
Remain closed |
23 |
100 |
N.A. |
N.A. |
96
|
MIL-53(Fe) |
N.A. |
N.A. |
5 |
95 |
10 |
56 |
106 and 107 |
Cu(dhbc)2(bpy) |
N.A. |
N.A. |
N.A. |
N.A. |
9 |
62 |
65
|
The switching pressure, one of the two most critical parameters for utility in the context of gas storage, is strongly affected by temperature. For example, the CO2 switching pressure for sql-1-Co-NCS at 195 K is 0.1 bar but it increases to 26.7 bar at 298 K.144 The multi-step switching nature of ELM-11 was overlooked for some time until low-temperature or high-pressure gas sorption isotherms were collected.72,86 Although the switching pressure for a given adsorbate can be accurately calculated using the Clausius–Clapeyron equation, once the phase transformation enthalpy is determined, it is difficult to predict the switching pressure across a range of adsorbates. For instance, it is a challenge to quantitatively predict the CH4 switching pressure even if we know the N2 and/or CO2 switching pressures of a given CN. Thus far, most switching CNs that exhibit high N2 and/or CO2 uptake at low (e.g. cryogenic) temperatures exhibit low or negligible CH4 uptake at higher temperatures and pressures. This is likely because CH4 tends to form relatively weak interactions with most CNs, especially compared to CO2. The switching pressure of CH4 is expected to be much higher than that of N2 or CO2 at the same conditions and usually falls beyond the measurement limits of most studies reported in the literature.
With respect to methane storage, there are currently <10 switching CNs with CH4 uptake measured at 298 K and only three (Co(bdp), Fe(bdp) and X-dia-1-Ni) exhibit >200 cm3 g−1 CH4 uptake (Fig. 22c).67,74 Considering its relevance to adsorbed natural gas (ANG) storage,226–233 the working capacity is typically determined as the quantity of NG that is deliverable between 5–35 or 5–65 bar, pressure ranges that are relevant for commercial combustion engines. In this context, Co(bdp) and Fe(bdp) would be expected to deliver around 100% of the stored NG thanks to their type F-IV isotherms.74 To put this in context, rigid CNs that exhibit benchmark gravimetric uptake (e.g. >500 cm3 g−1) tend to have much lower volumetric working capacities because of their relatively low densities (e.g. <0.3 g cm−3).233 Overall, Co(bdp) and Fe(bdp) exhibit comparable or superior volumetric working capacities (∼200 cm3 cm−3) compared to the benchmark rigid CNs (Fig. 22d), and meet the early DOE (U.S. Department of Energy) target for volumetric CH4 uptake (180 cm3 cm−3). Unfortunately, these working capacities remain well below the current DOE target of 263 cm3 cm−3 (350 cm3 cm−3 considering ca. 25% packing loss).234 Indeed, regardless of structural rigidity or flexibility, there is no adsorbent that yet meets the ambitious target for methane storage. Accordingly, development of new switching CNs with improved uptakes, the right switching pressures and proper densities is needed to properly exploit their intrinsically advantageous isotherms.
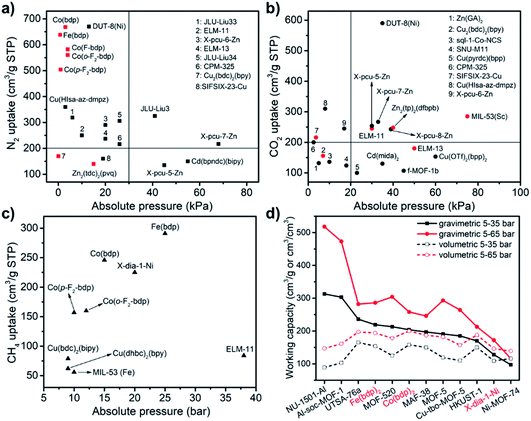 |
| Fig. 22 Comparison of switching CNs with respect to (a) N2 (77 K), (b) CO2 (195 K) and (c) CH4 (298/303 K) sorption. Black and red symbols denote CNs with type F-IVs and F-IVm isotherms respectively. For type F-IVm isotherms, only the switching pressures of the last step are shown. (d) Comparison of gravimetric and volumetric working capacities for CH4 at 298 K between current benchmarks for switching and rigid CNs. | |
4.2. Separations
Separations are of industrial relevance since most commodities are obtained as mixtures and require further purification steps prior to downstream processing and utilisation.235–241 Unfortunately, the energy footprint of separation/purification is generally high and currently represents ∼15% of the global energy consumption because of our reliance upon energy-intensive separation methods such as distillation, evaporation, chemisorption, and crystallization.242 Given that a three-fold increase in demand for commodities is projected to occur by 2050,224 more energy-efficient purification technologies are urgently required. In this context, adsorptive separation using porous solids is increasingly recognised as a promising alternative to traditional methods. Purification of a wide range of mixtures, from gaseous (e.g. CH4/CO2, CH4/N2, C2H2/CO2, C2H2/C2H4/C2H6, C3H4/C3H6/C3H8) to vapour and liquid forms (e.g. C5, C6 alkanes and C7, C8 aromatics) have been extensively studied using rigid porous CNs.235–241
Switching nonporous CNs remain underexplored in the context of separation but they are attracting increasing attention as stepwise adsorption can endow switching CNs with high adsorption selectivities, at least in principle (e.g. based on Henry’s law and/or IAST calculations). This is especially the case when a single component of a mixture exclusively induces structural transformation(s). However, in practice, separation performances tend to be inferior to the theoretical values derived from pure-component sorption isotherms. This is because of the complexities of dynamic conditions, especially sorption/diffusion kinetics and coadsorption. Switching CNs might also be unsuited for trace gas removal (e.g. <1000 ppm, or 0.1%) because the switching pressure is normally well above 0.1 kPa at room temperature. Nevertheless, switching CNs hold promise for adsorptive separation as reflected in some of the benchmark separation performances registered in Table 6. For example, ELM-11 exhibits good selectivity for CO2 over CH4 (66.7) at 273 K and 1 bar as verified by breakthrough experiments.243 Importantly, ELM-11 also retains this CO2/CH4 separation performance at 298 K and 20 bar, making it favourable for separation of a CH4/CO2 stream obtained directly from a natural gas source. Similarly, high-pressure purification of CH4 is also observed in Co(bdp), which exhibits “near-perfect” selectivity of CO2 over CH4 as confirmed by binary equilibrium adsorption experiments.103 Another sql CN, sql-1-Co-NCS, was found to exhibit excellent separation performance towards C8 aromatic isomers,145 one of the seven separation challenges that could “change the world”.242 This switching adsorbent layered material (SALMA) is the first sorbent that combines high xylene uptake (>50 wt%) with high selectivity (>5).145 MIL-53(Fe) also exhibited potential to separate xylene isomers,177 but its selectivity was determined to be lower than those of sql-1-Co-NCS and MIL-53(Al).145,244,245 Another recent example of a high performing switching CN is NTU-65 (also termed SIFSIX-23-Cu).152 Compared to zeolites and rigid CNs, NTU-65 exhibited comparable or superior separation performance for C2H4 purification from C2H2 and CO2 impurities. In summary, there is much scope to further explore the separation properties and performance of switching CNs which already show, perhaps counterintuitively, great promise for several separations that have been a great challenge for rigid physisorbents.
Table 6 Separation applications of representative switching CNsa
Switching CNs |
Mixtures |
Separation performance |
Methods |
Conditions |
Ref. |
BT: breakthrough; IAST: ideal adsorbed solution theory; BEA (QEA): binary (quaternary) equilibrium adsorption.
|
ELM-11 |
CO2/CH4 |
S
CO2/CH4: 31 |
BT (50 : 50) |
298 K, 20 bar |
128
|
S
CO2/CH4: 66.7 |
273 K, 1 bar |
CH4/N2 |
S
CH4/N2: 8.7 |
195 K, 20 bar |
Cu(dhbc)2(bpy) |
CO2/CH4 |
S
CO2/CH4: 28.9 |
298 K, 10 bar |
S
CO2/CH4: 53.4 |
273 K, 1 bar |
CH4/N2 |
S
CH4/N2: 5.5 |
273 K, 20 bar |
S
CH4/N2: 10.3 |
195 K, 1 bar |
ELM-11 |
C2H2/C2H4 |
S
CO2/CH4: 108 to 109 |
IAST (50 : 50) |
298 K, 1 bar |
85
|
S
CO2/CH4: 10–100 |
IAST (10 : 90) |
C2H2 preference |
BT (50 : 50) |
BT (10 : 90) |
ELM-13 |
S
CO2/CH4: 104 to 105 |
IAST (50 : 50) |
S
CO2/CH4: 104 to 105 |
IAST (10 : 90) |
C2H2 preference |
BT (50 : 50) |
BT (10 : 90) |
Co(bdp) |
CO2/CH4 |
Near-perfect selectivity (exact values not given) |
BEA (46 : 54) |
298 K, 6.7 bar |
103
|
BEA (42 : 58) |
298 K, 13.9 bar |
BEA (43 : 57) |
298 K, 25.3 bar |
S
CO2/CH4: 61 |
BEA (6 : 94) |
298 K, 58.6 bar |
NJU-Bai8 |
C3H6/C3H8 |
S
C3O6/C3H8: 4.6 |
IAST (50 : 50) |
298 K, 20 kPa |
78
|
C3H6 preference |
BT (20 : 20) |
298 K, 1 bar |
NTU-65 (SIFSIX-23-Cu) |
C2H2/C2H4 |
Outlet >99.95% C2H4 |
BT (1 : 99) |
263 K, 1 bar |
152
|
CO2/C2H4 |
BT (10 : 90) |
C2H2/CO2/C2H4 |
BT (1 : 9 : 90) |
Co(VTTE)2 |
C2H4/C2H6 |
S
C2H4/C2H6: 5.1 |
BEA (10 : 90) |
190 K, 1 bar |
70
|
S
C2H4/C2H6: 1.9 |
BEA (50 : 50) |
ELM-13 |
CO2/N2 |
S
CO2/N2: 13.3 |
BEA (50 : 50) |
273 K, 10 bar |
82
|
sql-1-Co-NCS |
OX/MX |
S
OX/MX: 7.5 |
BEA (1 : 1) |
Room temperature, liquid phase |
145
|
OX/PX |
S
OX/PX: 9.6 |
MX/PX |
S
MX/PX: 1.3 |
OX/EB |
S
OX/EB: 60.1 |
MX/EB |
S
MX/EB: 3.8 |
PX/EB |
S
PX/EB: 7.3 |
MIL-53(Fe) |
OX/MX |
S
OX/MX: 2.66 |
BT (1 : 1) |
323 K, 0.05 M in heptane |
175
|
OX/PX |
S
OX/MX: 1.88 |
MX/PX |
S
OX/MX: 1.51 |
DynaMOF-100 |
OX/PX/MX/EB |
PX preference |
QEA (1 : 1 : 1 : 1) |
Room temperature, liquid phase |
60
|
ST/EB |
ST preference |
BEA (1 : 1) |
Apart from working capacity and selectivity as discussed above, recyclability and kinetics are two other key metrics that must be evaluated for practical application, yet both remain largely understudied. Despite the strong recyclability demonstrated by Co(bdp)74 and X-pcu-n-Zn,146,147 several 3D switching CNs, such as X-dia-1-Ni,67 DUT-8(Ni)246 and [Zn2(DPT)2(bpy)],136 suffered from degradation in terms of switching pressure and uptake after multiple gas sorption cycles. In contrast, 2D layered switching CNs such as ELM-11 (ref. 246) and sql-1-Co-NCS144 demonstrated excellent recyclability over at least 100 cycles. The strong recyclability performance of several 2D CNs might be attributed to the relatively low structural strain and/or weak inter-network interactions that accompany expansion/contraction during recycling. With respect to kinetics, the CH4 adsorption kinetics of ELM-11 reached saturation uptake in 10 min (ref. 59) and we demonstrated that the CO2 adsorption of sql-1-Co-NCS requires less than 30 min.144 In general, the adsorption kinetics of switching CNs is even less well-studied than recyclability.
5. Conclusions
We detail herein the scope of CNs that switch between closed and open phases, an emerging class of physisorbents, and discuss the impact of metal ions, linker ligands and adsorbates on switching parameters upon properties of relevance to gas storage and separations. We also address the mechanisms associated with switching between closed and open phases. Switching CNs offer potential advantages over rigid CNs which results from their extreme flexibility, the specific stimulus-response and unusual sorption properties. However, both scientific and practical hurdles remain to be overcome before there can be utility.
From a scientific perspective, the synthetic design principles for switching or, more generally, even soft CNs largely remain to be established. This is despite the relative maturity of crystal engineering with respect to design of CNs from first principles. The introduction of switching elements into CNs and realisation of structural dynamism remains somewhat unpredictable due to uncertainties over their self-assembly processes and the complex interplay of local and global flexibility. Indeed, as revealed by Table 5, other than the effect of temperature and pressure upon switching pressure, there is no obvious correlation between sorbates and switching pressure other than the general observation that polar sorbates tend to promote switching. Improvements in understanding and predictability of switching will come from in-depth investigation of the origin and mechanism of switching, which remains limited by a reliance on X-ray diffraction.247 It is therefore important to further exploit in situ characterization using techniques such as nuclear magnetic resonance, Raman spectroscopy, transmission electron microscopy and neutron scattering to broaden the scopes offered by diffraction experiments.45 In this context, given the dynamics of switching transformations, time-resolved in situ techniques are of particular importance.176,248 It is also worth mentioning that the performance of CNs is determined not only by their atomic level structure, but also by their macroscopic features, i.e., morphology, particle size, etc. Therefore, further insights into the roles of particle size, morphology, defects and disorder are needed.249–251 Finally, development of machine learning and task-specific theoretical modelling approaches merit increased attention since they might not only address the underlying mechanisms, but also offer predictable modelling of the performance of switching CNs with pre-defined functions.252–254
From a practical viewpoint, the hygroscopicity of CNs must be taken into account in addition to the aforementioned performance metrics (i.e., recyclability and kinetics). This is because hygroscopicity can strongly impact both the lifetime and the performance of switching CNs.255 In addition, that switching between closed and open phases usually results in large volume changes in switching CNs may pose challenges to downstream processes such as formulation/shaping. It should be noted that the activation can also influence the switching pressure and uptake.28,136 Cost of ingredients and processing is also a consideration that must be addressed when assessing the viability of CN-based products and technologies. Ironically, even though this is not considered by most academic researchers, this is perhaps the one factor that a chemist can control. With respect to processing, the use of mechanochemistry such as ball milling and twin-screw extrusion256 offers promise for scale-up and several well-known rigid CNs have been prepared with high efficiency using mechanochemistry.257 The suitability of mechanochemistry for synthesis of switching or soft CNs is even more understudied though.
In conclusion, we are perhaps approaching the “end of the beginning”, but we are not yet there in terms of either design or properties. With respect to design, there are still just a few families of switching CNs and we remain mainly at the stage of empirical observation. With respect to properties, apart from adsorptive gas storage and mixture separation as discussed above, switching CNs and derived composites have been noted as having the potential to serve other emerging applications thanks to combination(s) of different applied stimuli such as light, redox and mechanical stress.258–261 Whereas these applications emphasise the relevance of switching CNs more than ever, there remains a large gap between a property serving as an indicator of performance and real-world applications. At this stage, ANG storage is perhaps the most promising short to medium term opportunity for end-use of switching CNs.
Conflicts of interest
There are no conflicts to declare.
Acknowledgements
M. J. Z. would like to acknowledge the support of Irish Research Council (IRCLA/2019/167) and Science Foundation Ireland (13/RP/B2549 and 16/IA/4624). S. M. thanks the Alexander von Humboldt Foundation for funding his postdoctoral research at TU Munich. S.-Q. W. would like to thank his colleague Dr Shaza Darwish for her proof reading and helpful suggestions.
References
- S. R. Batten, N. R. Champness, X.-M. Chen, J. Garcia-Martinez, S. Kitagawa, L. Öhrström, M. O’Keeffe, M. P. Suh and J. Reedijk, Pure Appl. Chem., 2013, 85, 1715–1724 CAS.
-
L. R. MacGillivray, Metal–organic frameworks: design and application, Wiley, Hoboken, 2010 Search PubMed.
-
D. Farrusseng, Metal–Organic Frameworks: Applications from Catalysis to Gas Storage, Wiley-VCH, Weinheim, 2011 Search PubMed.
- J. F. Keggin and F. D. Miles, Nature, 1936, 137, 577–578 CrossRef CAS.
- H. J. Buser, D. Schwarzenbach, W. Petter and A. Ludi, Inorg. Chem., 1977, 16, 2704–2710 CrossRef CAS.
- S. Mukherjee and M. J. Zaworotko, Trends Chem., 2020, 2, 506–518 CrossRef CAS.
- S. Kitagawa, R. Kitaura and S.-i. Noro, Angew. Chem., Int. Ed., 2004, 43, 2334–2375 CrossRef CAS PubMed.
-
S. R. Batten, S. M. Neville and D. R. Turner, Coordination Polymers: Design, Analysis and Application, Royal Society of Chemistry, London, 2009 Search PubMed.
- J. J. Perry IV, J. A. Perman and M. J. Zaworotko, Chem. Soc. Rev., 2009, 38, 1400–1417 RSC.
- T. R. Cook, Y.-R. Zheng and P. J. Stang, Chem. Rev., 2013, 113, 734–777 CrossRef CAS PubMed.
- B. Moulton and M. J. Zaworotko, Chem. Rev., 2001, 101, 1629–1658 CrossRef CAS PubMed.
- O. M. Yaghi, M. O’Keeffe, N. W. Ockwig, H. K. Chae, M. Eddaoudi and J. Kim, Nature, 2003, 423, 705–714 CrossRef CAS PubMed.
- B. F. Hoskins and R. Robson, J. Am. Chem. Soc., 1989, 111, 5962–5964 CrossRef CAS.
- B. F. Hoskins and R. Robson, J. Am. Chem. Soc., 1990, 112, 1546–1554 CrossRef CAS.
- S. Ma and H.-C. Zhou, Chem. Commun., 2010, 46, 44–53 RSC.
- J.-R. Li, J. Sculley and H.-C. Zhou, Chem. Rev., 2012, 112, 869–932 CrossRef CAS PubMed.
- P. A. Kobielska, A. J. Howarth, O. K. Farha and S. Nayak, Coord. Chem. Rev., 2018, 358, 92–107 CrossRef CAS.
- P. Horcajada, R. Gref, T. Baati, P. K. Allan, G. Maurin, P. Couvreur, G. Ferey, R. E. Morris and C. Serre, Chem. Rev., 2012, 112, 1232–1268 CrossRef CAS PubMed.
- J. Y. Lee, O. K. Farha, J. Roberts, K. A. Scheidt, S. B. T. Nguyen and J. T. Hupp, Chem. Soc. Rev., 2009, 38, 1450–1459 RSC.
- P. Z. Moghadam, A. Li, X.-W. Liu, R. Bueno-Perez, S.-D. Wang, S. B. Wiggin, P. A. Wood and D. Fairen-Jimenez, Chem. Sci., 2020, 11, 8373–8387 RSC.
- R. Taylor and P. A. Wood, Chem. Rev., 2019, 119, 9427–9477 CrossRef CAS PubMed.
- S. Kitagawa and M. Kondo, Bull. Chem. Soc. Jpn., 1998, 71, 1739–1753 CrossRef CAS.
- K. Uemura, R. Matsuda and S. Kitagawa, J. Solid State Chem., 2005, 178, 2420–2429 CrossRef CAS.
- S. Kitagawa and K. Uemura, Chem. Soc. Rev., 2005, 34, 109–119 RSC.
- G. Alberti, S. Murcia-Mascaros and R. Vivani, J.
Am. Chem. Soc., 1998, 120, 9291–9295 CrossRef CAS.
- G. Alberti, E. Brunet, C. Dionigi, O. Juanes, M. J. de la Mata, J. C. Rodríguez-Ubis and R. Vivani, Angew. Chem., Int. Ed., 1999, 38, 3351–3353 CrossRef CAS PubMed.
- O.-S. Jung, Y. J. Kim, Y.-A. Lee, J. K. Park and H. K. Chae, J. Am. Chem. Soc., 2000, 122, 9921–9925 CrossRef CAS.
- D. Li and K. Kaneko, Chem. Phys. Lett., 2001, 335, 50–56 CrossRef CAS.
- A. J. Fletcher, E. J. Cussen, T. J. Prior, M. J. Rosseinsky, C. J. Kepert and K. M. Thomas, J. Am. Chem. Soc., 2001, 123, 10001–10011 CrossRef CAS PubMed.
- S. Horike, S. Shimomura and S. Kitagawa, Nat. Chem., 2009, 1, 695–704 CrossRef CAS PubMed.
- S. Krause, N. Hosono and S. Kitagawa, Angew. Chem., Int. Ed., 2020, 59, 15325–15341 CrossRef CAS PubMed.
- S. Castellanos, F. Kapteijn and J. Gascon, CrystEngComm, 2016, 18, 4006–4012 RSC.
- H.-L. Zhou, Y.-B. Zhang, J.-P. Zhang and X.-M. Chen, Nat. Commun., 2015, 6, 1–7 Search PubMed.
- I. Beurroies, M. Boulhout, P. L. Llewellyn, B. Kuchta, G. Férey, C. Serre and R. Denoyel, Angew. Chem., Int. Ed., 2010, 49, 7526–7529 CrossRef CAS PubMed.
- M.-H. Yu, B. Space, D. Franz, W. Zhou, C. He, L. Li, R. Krishna, Z. Chang, W. Li, T.-L. Hu and X.-H. Bu, J. Am. Chem. Soc., 2019, 141, 17703–17712 CrossRef CAS PubMed.
- H. Zeng, M. Xie, Y.-L. Huang, Y. Zhao, X.-J. Xie, J.-P. Bai, M.-Y. Wan, R. Krishna, W. Lu and D. Li, Angew. Chem., Int. Ed., 2019, 58, 8515–8519 CrossRef CAS PubMed.
- P. Zhao, H. Fang, S. Mukhopadhyay, A. Li, S. Rudic, I. J. McPherson, C. C. Tang, D. Fairen-Jimenez, S. C. E. Tsang and S. A. T. Redfern, Nat. Commun., 2019, 10, 1–8 CrossRef PubMed.
- G. Férey and C. Serre, Chem. Soc. Rev., 2009, 38, 1380–1399 RSC.
- A. J. Fletcher, K. M. Thomas and M. J. Rosseinsky, J. Solid State Chem., 2005, 178, 2491–2510 CrossRef CAS.
- C. R. Murdock, B. C. Hughes, Z. Lu and D. M. Jenkins, Coord. Chem. Rev., 2014, 258, 119–136 CrossRef.
- A. Schneemann, V. Bon, I. Schwedler, I. Senkovska, S. Kaskel and R. A. Fischer, Chem. Soc. Rev., 2014, 43, 6062–6096 RSC.
- B. Qian, Z. Chang and X.-H. Bu, Top. Curr. Chem., 2020, 378, 5 CrossRef CAS PubMed.
- F. Bigdeli, C. T. Lollar, A. Morsali and H.-C. Zhou, Angew. Chem., Int. Ed., 2020, 59, 4652–4669 CrossRef CAS PubMed.
- S. B. Peh, A. Karmakar and D. Zhao, Trends Chem., 2020, 2, 199–213 CrossRef CAS.
- V. Bon, E. Brunner, A. Pöppl and S. Kaskel, Adv. Funct. Mater., 2020, 30, 1907847 CrossRef CAS.
- J.-P. Zhang, H.-L. Zhou, D.-D. Zhou, P.-Q. Liao and X.-M. Chen, Natl. Sci. Rev., 2018, 5, 907–919 CrossRef CAS.
- L. Vanduyfhuys, S. Rogge, J. Wieme, S. Vandenbrande, G. Maurin, M. Waroquier and V. Van Speybroeck, Nat. Commun., 2018, 9, 1–9 CrossRef CAS PubMed.
- Z. Chang, D.-H. Yang, J. Xu, T.-L. Hu and X.-H. Bu, Adv. Mater., 2015, 27, 5432–5441 CrossRef CAS PubMed.
- S. K. Elsaidi, M. H. Mohamed, D. Banerjee and P. K. Thallapally, Coord. Chem. Rev., 2018, 358, 125–152 CrossRef CAS.
- J. H. Lee, S. Jeoung, Y. G. Chung and H. R. Moon, Coord. Chem. Rev., 2019, 389, 161–188 CrossRef CAS.
- K. Seki, Phys. Chem. Chem. Phys., 2002, 4, 1968–1971 RSC.
- K. Biradha and M. Fujita, Angew. Chem., Int. Ed., 2002, 41, 3392–3395 CrossRef CAS PubMed.
- J.-S. Qin, S. Yuan, A. Alsalme and H.-C. Zhou, ACS Appl. Mater. Interfaces, 2017, 9, 33408–33412 CrossRef CAS PubMed.
- Q.-f. Qiu, C.-X. Chen, Z.-W. Wei, C.-C. Cao, N.-X. Zhu, H.-P. Wang, D. Wang, J.-J. Jiang and C.-Y. Su, Inorg. Chem., 2019, 58, 61–64 CrossRef CAS PubMed.
- C. Serre, F. Millange, C. Thouvenot, M. Nogues, G. Marsolier, D. Louër and G. Férey, J. Am. Chem. Soc., 2002, 124, 13519–13526 CrossRef CAS PubMed.
- C. Mellot-Draznieks, C. Serre, S. Surblé, N. Audebrand and G. Férey, J. Am. Chem. Soc., 2005, 127, 16273–16278 CrossRef CAS PubMed.
- C. Serre, C. Mellot-Draznieks, S. Surblé, N. Audebrand, Y. Filinchuk and G. Férey, Science, 2007, 315, 1828–1831 CrossRef CAS PubMed.
- A. U. Ortiz, A. Boutin, A. H. Fuchs and F.-X. Coudert, Phys. Rev. Lett., 2012, 109, 195502 CrossRef PubMed.
- H. Kanoh, A. Kondo, H. Noguchi, H. Kajiro, A. Tohdoh, Y. Hattori, W.-C. Xu, M. Inoue, T. Sugiura, K. Morita, H. Tanaka, T. Ohba and K. Kaneko, J. Colloid Interface Sci., 2009, 334, 1–7 CrossRef CAS PubMed.
- K. Yang, G. Zhou and Q. Xu, RSC Adv., 2016, 6, 37506–37514 RSC.
- H. Deng, M. A. Olson, J. F. Stoddart and O. M. Yaghi, Nat. Chem., 2010, 2, 439–443 CrossRef CAS PubMed.
- A. Gonzalez-Nelson, F.-X. Coudert and M. A. van der Veen, Nanomaterials, 2019, 9, 330 CrossRef CAS PubMed.
- S. Krause, V. Bon, U. Stoeck, I. Senkovska, D. M. Toebbens, D. Wallacher and S. Kaskel, Angew. Chem., Int. Ed., 2017, 56, 10676–10680 CrossRef CAS PubMed.
- P. Kanoo, R. Haldar, S. K. Reddy, A. Hazra, S. Bonakala, R. Matsuda, S. Kitagawa, S. Balasubramanian and T. K. Maji, Chem.–Eur. J., 2016, 22, 15864–15873 CrossRef CAS PubMed.
- R. Kitaura, K. Seki, G. Akiyama and S. Kitagawa, Angew. Chem., Int. Ed., 2003, 42, 428–431 CrossRef CAS PubMed.
- D. Tanaka, K. Nakagawa, M. Higuchi, S. Horike, Y. Kubota, T. C. Kobayashi, M. Takata and S. Kitagawa, Angew. Chem., Int. Ed., 2008, 47, 3914–3918 CrossRef CAS PubMed.
- Q. Y. Yang, P. Lama, S. Sen, M. Lusi, K. J. Chen, W. Y. Gao, M. Shivanna, T. Pham, N. Hosono, S. Kusaka, J. J. Perry IV, S. Ma, B. Space, L. J. Barbour, S. Kitagawa and M. J. Zaworotko, Angew. Chem., Int. Ed., 2018, 57, 5684–5689 CrossRef CAS PubMed.
- M. Thommes, K. Kaneko, A. V. Neimark, J. P. Olivier, F. Rodriguez-Reinoso, J. Rouquerol and K. S. Sing, Pure Appl. Chem., 2015, 87, 1051–1069 CAS.
- S. Bourrelly, P. L. Llewellyn, C. Serre, F. Millange, T. Loiseau and G. Férey, J. Am. Chem. Soc., 2005, 127, 13519–13521 CrossRef CAS PubMed.
- A. Kondo, H. Noguchi, L. Carlucci, D. M. Proserpio, G. Ciani, H. Kajiro, T. Ohba, H. Kanoh and K. Kaneko, J. Am. Chem. Soc., 2007, 129, 12362–12363 CrossRef CAS PubMed.
- A. Schneemann, P. Vervoorts, I. Hante, M. Tu, S. Wannapaiboon, C. Sternemann, M. Paulus, D. C. F. Wieland, S. Henke and R. A. Fischer, Chem. Mater., 2018, 30, 1667–1676 CrossRef CAS.
- S.-Q. Wang, X.-Q. Meng, M. Vandichel, S. Darwish, Z. Chang, X.-H. Bu and M. J. Zaworotko, ACS Appl. Mater. Interfaces, 2021, 13, 23877–23883 CrossRef CAS PubMed.
- M. Shivanna, Q.-Y. Yang, A. Bajpai, S. Sen, N. Hosono, S. Kusaka, T. Pham, K. A. Forrest, B. Space, S. Kitagawa and M. J. Zaworotko, Sci. Adv., 2018, 4, eaaq1636 CrossRef PubMed.
- J. A. Mason, J. Oktawiec, M. K. Taylor, M. R. Hudson, J. Rodriguez, J. E. Bachman, M. I. Gonzalez, A. Cervellino, A. Guagliardi, C. M. Brown, P. L. Llewellyn, N. Masciocchi and J. R. Long, Nature, 2015, 527, 357–361 CrossRef CAS PubMed.
- E. Coronado and G. Minguez Espallargas, Chem. Soc. Rev., 2013, 42, 1525–1539 RSC.
- S. Castellanos, F. Kapteijn and J. Gascon, CrystEngComm, 2016, 18, 4006–4012 RSC.
- O. Drath and C. Boskovic, Coord. Chem. Rev., 2018, 375, 256–266 CrossRef CAS.
- W. D. Schaeffer, W. S. Dorsey, D. A. Skinner and C. G. Christian, J. Am. Chem. Soc., 1957, 79, 5870–5876 CrossRef CAS.
- S. A. Allison and R. M. Barrer, J. Chem. Soc. A, 1969, 1717–1723 RSC.
- T. Dewa, K. Endo and Y. Aoyama, J. Am. Chem. Soc., 1998, 120, 8933–8940 CrossRef CAS.
- Z. Wang, N. Sikdar, S.-Q. Wang, X. Li, M. Yu, X.-H. Bu, Z. Chang, X. Zou, Y. Chen, P. Cheng, K. Yu, M. J. Zaworotko and Z. Zhang, J. Am. Chem. Soc., 2019, 141, 9408–9414 CrossRef PubMed.
- N. Sun, S.-Q. Wang, R. Zou, W.-G. Cui, A. Zhang, T. Zhang, Q. Li, Z.-Z. Zhuang, Y.-H. Zhang, J. Xu, M. J. Zaworotko and X.-H. Bu, Chem. Sci., 2019, 10, 8850–8854 RSC.
- A. M. Kałuża, S. Mukherjee, S.-Q. Wang, D. J. O’Hearn and M. J. Zaworotko, Chem. Commun., 2020, 56, 1940–1943 RSC.
- G. B. Gardner, D. Venkataraman, J. S. Moore and S. Lee, Nature, 1995, 374, 792–795 CrossRef CAS.
- L. Li, R. Krishna, Y. Wang, X. Wang, J. Yang and J. Li, Eur. J. Inorg. Chem., 2016, 2016, 4457–4462 CrossRef CAS.
- M. Ichikawa, A. Kondo, H. Noguchi, N. Kojima, T. Ohba, H. Kajiro, Y. Hattori and H. Kanoh, Langmuir, 2016, 32, 9722–9726 CrossRef CAS PubMed.
- S. Hiraide, H. Tanaka, N. Ishikawa and M. T. Miyahara, ACS Appl. Mater. Interfaces, 2017, 9, 41066–41077 CrossRef CAS PubMed.
- A. Kondo, H. Noguchi, S. Ohnishi, H. Kajiro, A. Tohdoh, Y. Hattori, W.-C. Xu, H. Tanaka, H. Kanoh and K. Kaneko, Nano Lett., 2006, 6, 2581–2584 CrossRef CAS PubMed.
- H. Kajiro, A. Kondo, K. Kaneko and H. Kanoh, Int. J. Mol. Sci., 2010, 11, 3803–3845 CrossRef CAS PubMed.
- V. Bon, I. Senkovska, D. Wallacher, A. Heerwig, N. Klein, I. Zizak, R. Feyerherm, E. Dudzik and S. Kaskel, Microporous Mesoporous Mater., 2014, 188, 190–195 CrossRef CAS.
- K. Uemura, S. Kitagawa, M. Kondo, K. Fukui, R. Kitaura, H.-C. Chang and T. Mizutani, Chem.–Eur. J., 2002, 8, 3586–3600 CrossRef CAS.
- R. Kitaura, K. Fujimoto, S. i. Noro, M. Kondo and S. Kitagawa, Angew. Chem., Int. Ed., 2002, 41, 133–135 CrossRef CAS PubMed.
- Y. Sakata, S. Furukawa, M. Kondo, K. Hirai, N. Horike, Y. Takashima, H. Uehara, N. Louvain, M. Meilikhov, T. Tsuruoka, S. Isoda, W. Kosaka, O. Sakata and S. Kitagawa, Science, 2013, 339, 193–196 CrossRef CAS PubMed.
- Y. Inubushi, S. Horike, T. Fukushima, G. Akiyama, R. Matsuda and S. Kitagawa, Chem. Commun., 2010, 46, 9229–9231 RSC.
- K. Uemura, S. Kitagawa, K. Fukui and K. Saito, J. Am. Chem. Soc., 2004, 126, 3817–3828 CrossRef CAS PubMed.
- T. K. Maji, G. Mostafa, R. Matsuda and S. Kitagawa, J. Am. Chem. Soc., 2005, 127, 17152–17153 CrossRef CAS PubMed.
- B. Chen, C. Liang, J. Yang, D. S. Contreras, Y. L. Clancy, E. B. Lobkovsky, O. M. Yaghi and S. Dai, Angew. Chem., Int. Ed., 2006, 45, 1390–1393 CrossRef CAS PubMed.
- S. Xiang, W. Zhou, J. M. Gallegos, Y. Liu and B. Chen, J. Am. Chem. Soc., 2009, 131, 12415–12419 CrossRef CAS PubMed.
- P. M. Bhatt, E. Batisai, V. J. Smith and L. J. Barbour, Chem. Commun., 2016, 52, 11374–11377 RSC.
- S. Shimomura, S. Horike, R. Matsuda and S. Kitagawa, J. Am. Chem. Soc., 2007, 129, 10990–10991 CrossRef CAS PubMed.
- H. J. Choi, M. Dincă and J. R. Long, J. Am. Chem. Soc., 2008, 130, 7848–7850 CrossRef CAS PubMed.
- F. Salles, G. Maurin, C. Serre, P. L. Llewellyn, C. Knofel, H. J. Choi, Y. Filinchuk, L. Oliviero, A. Vimont, J. R. Long and G. Ferey, J. Am. Chem. Soc., 2010, 132, 13782–13788 CrossRef CAS PubMed.
- M. K. Taylor, T. Runčevski, J. Oktawiec, J. E. Bachman, R. L. Siegelman, H. Jiang, J. A. Mason, J. D. Tarver and J. R. Long, J. Am. Chem. Soc., 2018, 140, 10324–10331 CrossRef CAS PubMed.
- A. Kondo, A. Chinen, H. Kajiro, T. Nakagawa, K. Kato, M. Takata, Y. Hattori, F. Okino, T. Ohba, K. Kaneko and H. Kanoh, Chem.–Eur. J., 2009, 15, 7549–7553 CrossRef CAS PubMed.
- H. S. Choi and M. P. Suh, Angew. Chem., Int. Ed., 2009, 48, 6865–6869 CrossRef CAS PubMed.
- P. L. Llewellyn, P. Horcajada, G. Maurin, T. Devic, N. Rosenbach, S. Bourrelly, C. Serre, D. Vincent, S. Loera-Serna, Y. Filinchuk and G. Ferey, J. Am. Chem. Soc., 2009, 131, 13002–13008 CrossRef CAS PubMed.
- N. Guillou, S. Bourrelly, P. L. Llewellyn, R. I. Walton and F. Millange, CrystEngComm, 2015, 17, 422–429 RSC.
- M. Agrawal, S. Bhattacharyya, Y. Huang, K. C. Jayachandrababu, C. R. Murdock, J. A. Bentley, A. Rivas-Cardona, M. M. Mertens, K. S. Walton, D. S. Sholl and S. Nair, J. Phys. Chem. C, 2018, 122, 386–397 CrossRef CAS.
- J. Seo, R. Matsuda, H. Sakamoto, C. Bonneau and S. Kitagawa, J. Am. Chem. Soc., 2009, 131, 12792–12800 CrossRef CAS PubMed.
- H. Chun and J. Seo, Inorg. Chem., 2009, 48, 9980–9982 CrossRef CAS PubMed.
- J. Rabone, Y. F. Yue, S. Y. Chong, K. C. Stylianou, J. Bacsa, D. Bradshaw, G. R. Darling, N. G. Berry, Y. Z. Khimyak, A. Y. Ganin, P. Wiper, J. B. Claridge and M. J. Rosseinsky, Science, 2010, 329, 1053–1057 CrossRef CAS PubMed.
- T. Fukushima, S. Horike, Y. Inubushi, K. Nakagawa, Y. Kubota, M. Takata and S. Kitagawa, Angew. Chem., Int. Ed., 2010, 49, 4820–4824 CrossRef CAS PubMed.
- N. Klein, C. Herzog, M. Sabo, I. Senkovska, J. Getzschmann, S. Paasch, M. R. Lohe, E. Brunner and S. Kaskel, Phys. Chem. Chem. Phys., 2010, 12, 11778–11784 RSC.
- N. Klein, H. C. Hoffmann, A. Cadiau, J. Getzschmann, M. R. Lohe, S. Paasch, T. Heydenreich, K. Adil, I. Senkovska, E. Brunner and S. Kaskel, J. Mater. Chem., 2012, 22, 10303–10312 RSC.
- V. Bon, N. Klein, I. Senkovska, A. Heerwig, J. Getzschmann, D. Wallacher, I. Zizak, M. Brzhezinskaya, U. Mueller and S. Kaskel, Phys. Chem. Chem. Phys., 2015, 17, 17471–17479 RSC.
- N. Kavoosi, T. Savchenko, I. Senkovska, M. Maliuta, V. Bon, A. Eychmüller and S. Kaskel, Microporous Mesoporous Mater., 2018, 271, 169–174 CrossRef CAS.
- S. Ehrling, I. Senkovska, V. Bon, J. D. Evans, P. Petkov, Y. Krupskaya, V. Kataev, T. Wulf, A. Krylov, A. Vtyurin, S. Krylova, S. Adichtchev, E. Slyusareva, M. S. Weiss, B. Büchner, T. Heine and S. Kaskel, J. Mater. Chem. A, 2019, 7, 21459–21475 RSC.
- H. Wu, R. S. Reali, D. A. Smith, M. C. Trachtenberg and J. Li, Chem.–Eur. J., 2010, 16, 13951–13954 CrossRef CAS PubMed.
- N. Nijem, H. Wu, P. Canepa, A. Marti, K. J. Balkus, T. Thonhauser, J. Li and Y. J. Chabal, J. Am. Chem. Soc., 2012, 134, 15201–15204 CrossRef CAS PubMed.
- J. Seo, C. Bonneau, R. Matsuda, M. Takata and S. Kitagawa, J. Am. Chem. Soc., 2011, 133, 9005–9013 CrossRef CAS PubMed.
- S. Henke, A. Schneemann, A. Wuetscher and R. A. Fischer, J. Am. Chem. Soc., 2012, 134, 9464–9474 CrossRef CAS PubMed.
- J. P. Mowat, V. R. Seymour, J. M. Griffin, S. P. Thompson, A. M. Slawin, D. Fairen-Jimenez, T. Düren, S. E. Ashbrook and P. A. Wright, Dalton Trans., 2012, 41, 3937–3941 RSC.
- L. Chen, J. P. S. Mowat, D. Fairen-Jimenez, C. A. Morrison, S. P. Thompson, P. A. Wright and T. Duren, J. Am. Chem. Soc., 2013, 135, 15763–15773 CrossRef CAS PubMed.
- X.-M. Liu, R.-B. Lin, J.-P. Zhang and X.-M. Chen, Inorg. Chem., 2012, 51, 5686–5692 CrossRef CAS PubMed.
- K. Fukuhara, S.-i. Noro, K. Sugimoto, T. Akutagawa, K. Kubo and T. Nakamura, Inorg. Chem., 2013, 52, 4229–4237 CrossRef CAS PubMed.
- B. Joarder, S. Mukherjee, A. K. Chaudhari, A. V. Desai, B. Manna and S. K. Ghosh, Chem.–Eur. J., 2014, 20, 15303–15308 CrossRef CAS PubMed.
- S. Mukherjee, B. Joarder, B. Manna, A. V. Desai, A. K. Chaudhari and S. K. Ghosh, Sci. Rep., 2014, 4, 5761 CrossRef CAS PubMed.
- S. Mukherjee, B. Joarder, A. V. Desai, B. Manna, R. Krishna and S. K. Ghosh, Inorg. Chem., 2015, 54, 4403–4408 CrossRef CAS PubMed.
- J. Wang, J. Luo, J. Zhao, D.-S. Li, G. Li, Q. Huo and Y. Liu, Cryst. Growth Des., 2014, 14, 2375–2380 CrossRef CAS.
- M. Handke, H. Weber, M. Lange, J. Moellmer, J. Lincke, R. Glaeser, R. Staudt and H. Krautscheid, Inorg. Chem., 2014, 53, 7599–7607 CrossRef CAS PubMed.
- C. Wang, L. Li, J. G. Bell, X. Lv, S. Tang, X. Zhao and K. M. Thomas, Chem. Mater., 2015, 27, 1502–1516 CrossRef CAS.
- P. Kanoo, R. Haldar, S. K. Reddy, A. Hazra, S. Bonakala, R. Matsuda, S. Kitagawa, S. Balasubramanian and T. K. Maji, Chem.–Eur. J., 2016, 22, 15864–15873 CrossRef CAS PubMed.
- M. K. Taylor, T. Runčevski, J. Oktawiec, M. I. Gonzalez, R. L. Siegelman, J. A. Mason, J. Ye, C. M. Brown and J. R. Long, J. Am. Chem. Soc., 2016, 138, 15019–15026 CrossRef CAS PubMed.
- D. Banerjee, H. Wang, A. M. Plonka, T. J. Emge, J. B. Parise and J. Li, Chem.–Eur. J., 2016, 22, 11816–11825 CrossRef CAS PubMed.
- X. Sun, S. Yao, G. Li, L. Zhang, Q. Huo and Y. Liu, Inorg. Chem., 2017, 56, 6645–6651 CrossRef CAS PubMed.
- E. R. Engel, A. Jouaiti, C. X. Bezuidenhout, M. W. Hosseini and L. J. Barbour, Angew. Chem., 2017, 129, 9000–9004 CrossRef.
- P. Lama and L. J. Barbour, J. Am. Chem. Soc., 2018, 140, 2145–2150 CrossRef CAS PubMed.
- S. Sen, N. Hosono, J.-J. Zheng, S. Kusaka, R. Matsuda, S. Sakaki and S. Kitagawa, J. Am. Chem. Soc., 2017, 139, 18313–18321 CrossRef CAS PubMed.
- S. Krause, V. Bon, U. Stoeck, I. Senkovska, D. M. Toebbens, D. Wallacher and S. Kaskel, Angew. Chem., Int. Ed., 2017, 56, 10676–10680 CrossRef CAS PubMed.
- S. Krause, V. Bon, H. Du, R. E. Dunin-Borkowski, U. Stoeck, I. Senkovska and S. Kaskel, Beilstein J. Nanotechnol., 2019, 10, 1737–1744 CrossRef CAS PubMed.
- J. Jin, X. Zhao, P. Feng and X. Bu, Angew. Chem., Int. Ed., 2018, 57, 3737–3741 CrossRef CAS PubMed.
- H. Yang, F. Guo, P. Lama, W.-Y. Gao, H. Wu, L. J. Barbour, W. Zhou, J. Zhang, B. Aguila and S. Ma, ACS Cent. Sci., 2018, 4, 1194–1200 CrossRef CAS PubMed.
- Y.-X. Shi, W.-X. Li, W.-H. Zhang and J.-P. Lang, Inorg. Chem., 2018, 57, 8627–8633 CrossRef CAS PubMed.
- S.-Q. Wang, Q.-Y. Yang, S. Mukherjee, D. O’Nolan, E. Patyk-Kazmierczak, K.-J. Chen, M. Shivanna, C. Murray, C. C. Tang and M. J. Zaworotko, Chem. Commun., 2018, 54, 7042–7045 RSC.
- S.-Q. Wang, S. Mukherjee, E. Patyk-Kaźmierczak, S. Darwish, A. Bajpai, Q.-Y. Yang and M. J. Zaworotko, Angew. Chem., Int. Ed., 2019, 58, 6630–6634 CrossRef CAS PubMed.
- A.-X. Zhu, Q.-Y. Yang, A. Kumar, C. Crowley, S. Mukherjee, K.-J. Chen, S.-Q. Wang, D. O’Nolan, M. Shivanna and M. J. Zaworotko, J. Am. Chem. Soc., 2018, 140, 15572–15576 CrossRef CAS PubMed.
- A.-X. Zhu, Q.-Y. Yang, S. Mukherjee, A. Kumar, C.-H. Deng, A. A. Bezrukov, M. Shivanna and M. J. Zaworotko, Angew. Chem., Int. Ed., 2019, 58, 18212–18217 CrossRef CAS PubMed.
- X. Wang, R. Krishna, L. Li, B. Wang, T. He, Y.-Z. Zhang, J.-R. Li and J. Li, Chem. Eng. J., 2018, 346, 489–496 CrossRef CAS.
- A. Hazra, D. P. van Heerden, S. Sanyal, P. Lama, C. Esterhuysen and L. J. Barbour, Chem. Sci., 2019, 10, 10018–10024 RSC.
- S. Millan, B. Gil-Hernandez, E. Milles, S. Gokpinar, G. Makhloufi, A. Schmitz, C. Schlusener and C. Janiak, Dalton Trans., 2019, 48, 8057–8067 RSC.
- B.-Q. Song, Q.-Y. Yang, S.-Q. Wang, M. Vandichel, A. Kumar, C. Crowley, N. Kumar, C.-H. Deng, V. Gascon Perez, M. Lusi, H. Wu, W. Zhou and M. J. Zaworotko, J. Am. Chem. Soc., 2020, 142, 6896–6901 CrossRef CAS PubMed.
- Q. Dong, X. Zhang, S. Liu, R. B. Lin, Y. Guo, Y. Ma, A. Yonezu, R. Krishna, G. Liu, J. Duan, R. Matsuda, W. Jin and B. Chen, Angew. Chem., Int. Ed., 2020, 59, 22756–22762 CrossRef CAS PubMed.
- A. Kondo, H. Kajiro, T. Nakagawa, H. Tanaka and H. Kanoh, Dalton Trans., 2020, 49, 3692–3699 RSC.
- K. Roztocki, F. Formalik, A. Krawczuk, I. Senkovska, B. Kuchta, S. Kaskel and D. Matoga, Angew. Chem., Int. Ed., 2020, 59, 4491–4497 CrossRef CAS PubMed.
- F. Millange and R. I. Walton, Isr. J. Chem., 2018, 58, 1019–1035 CrossRef CAS.
- F. Millange, C. Serre and G. Ferey, Chem. Commun., 2002, 822–823 RSC.
- G. Ferey, M. Latroche, C. Serre, F. Millange, T. Loiseau and A. Percheron-Guegan, Chem. Commun., 2003, 2976–2977 RSC.
- T. R. Whitfield, X. Wang, L. Liu and A. J. Jacobson, Solid State Sci., 2005, 7, 1096–1103 CrossRef CAS.
- E. V. Anokhina, M. Vougo-Zanda, X. Wang and A. J. Jacobson, J. Am. Chem. Soc., 2005, 127, 15000–15001 CrossRef CAS PubMed.
- M. Vougo-Zanda, J. Huang, E. Anokhina, X. Wang and A. J. Jacobson, Inorg. Chem., 2008, 47, 11535–11542 CrossRef CAS PubMed.
- J. P. S. Mowat, S. R. Miller, A. M. Z. Slawin, V. R. Seymour, S. E. Ashbrook and P. A. Wright, Microporous Mesoporous Mater., 2011, 142, 322–333 CrossRef CAS.
- N. L. Rosi, J. Kim, M. Eddaoudi, B. Chen, M. O’Keeffe and O. M. Yaghi, J. Am. Chem. Soc., 2005, 127, 1504–1518 CrossRef CAS PubMed.
- A. Schoedel, M. Li, D. Li, M. O’Keeffe and O. M. Yaghi, Chem. Rev., 2016, 116, 12466–12535 CrossRef CAS PubMed.
- D. J. O’Hearn, A. Bajpai and M. J. Zaworotko, Small, 2021, 17, 2006351 CrossRef PubMed.
- C. Serre, F. Millange, C. Thouvenot, M. Nogues, G. Marsolier, D. Louër and G. Férey, J. Am. Chem. Soc., 2002, 124, 13519–13526 CrossRef CAS PubMed.
- T. Loiseau, C. Serre, C. Huguenard, G. Fink, F. Taulelle, M. Henry, T. Bataille and G. Férey, Chem.–Eur. J., 2004, 10, 1373–1382 CrossRef CAS PubMed.
- N. Guillou, F. Millange and R. I. Walton, Chem. Commun., 2011, 47, 713–715 RSC.
- C. Serre, S. Bourrelly, A. Vimont, N. A. Ramsahye, G. Maurin, P. L. Llewellyn, M. Daturi, Y. Filinchuk, O. Leynaud, P. Barnes and G. Ferey, Adv. Mater., 2007, 19, 2246–2251 CrossRef CAS.
- S. Bourrelly, B. Moulin, A. Rivera, G. Maurin, S. Devautour-Vinot, C. Serre, T. Devic, P. Horcajada, A. Vimont, G. Clet, M. Daturi, J.-C. Lavalley, S. Loera-Serna, R. Denoyel, P. L. Llewellyn and G. Ferey, J. Am. Chem. Soc., 2010, 132, 9488–9498 CrossRef CAS PubMed.
- T. K. Trung, P. Trens, N. Tanchoux, S. Bourrelly, P. L. Llewellyn, S. Loera-Serna, C. Serre, T. Loiseau, F. Fajula and G. Ferey, J. Am. Chem. Soc., 2008, 130, 16926–16932 CrossRef CAS PubMed.
- V. Finsy, C. E. A. Kirschhock, G. Vedts, M. Maes, L. Alaerts, D. E. De Vos, G. V. Baron and J. F. M. Denayer, Chem.–Eur. J., 2009, 15, 7724–7731 CrossRef CAS PubMed.
- F. Millange, N. Guillou, R. I. Walton, J.-M. Greneche, I. Margiolaki and G. Ferey, Chem. Commun., 2008, 4732–4734 RSC.
- F. Millange, C. Serre, N. Guillou, G. Ferey and R. I. Walton, Angew. Chem., Int. Ed., 2008, 47, 4100–4105 CrossRef CAS PubMed.
- P. Horcajada, C. Serre, G. Maurin, N. A. Ramsahye, F. Balas, M. Vallet-Regi, M. Sebban, F. Taulelle and G. Ferey, J. Am. Chem. Soc., 2008, 130, 6774–6780 CrossRef CAS PubMed.
- F. Millange, N. Guillou, M. E. Medina, G. Ferey, A. Carlin-Sinclair, K. M. Golden and R. I. Walton, Chem. Mater., 2010, 22, 4237–4245 CrossRef CAS.
- R. I. Walton, A. S. Munn, N. Guillou and F. Millange, Chem.–Eur. J., 2011, 17, 7069–7079 CrossRef CAS PubMed.
- R. El Osta, A. Carlin-Sinclair, N. Guillou, R. I. Walton, F. Vermoortele, M. Maes, D. de Vos and F. Millange, Chem. Mater., 2012, 24, 2781–2791 CrossRef CAS.
- B. Van de Voorde, A. S. Munn, N. Guillou, F. Millange, D. E. De Vos and R. I. Walton, Phys. Chem. Chem. Phys., 2013, 15, 8606–8615 RSC.
- M. Sanselme, J.-M. Grenèche, M. Riou-Cavellec and G. Férey, Solid State Sci., 2004, 6, 853–858 CrossRef CAS.
- S. Bauer, C. Serre, T. Devic, P. Horcajada, J. Marrot, G. Férey and N. Stock, Inorg. Chem., 2008, 47, 7568–7576 CrossRef CAS PubMed.
- T. Devic, P. Horcajada, C. Serre, F. Salles, G. Maurin, B. Moulin, D. Heurtaux, G. Clet, A. Vimont, J.-M. Greneche, B. Le Ouay, F. Moreau, E. Magnier, Y. Filinchuk, J. Marrot, J.-C. Lavalley, M. Daturi and G. Ferey, J. Am. Chem. Soc., 2010, 132, 1127–1136 CrossRef CAS PubMed.
- N. A. Ramsahye, T.-K. Trung, S. Bourrelly, Q.-Y. Yang, T. Devic, G. Maurin, P. Horcajada, P. L. Llewellyn, P. Yot, C. Serre, Y. Filinchuk, F. Fajula, G. Ferey and P. Trens, J. Phys. Chem. C, 2011, 115, 18683–18695 CrossRef CAS.
- T. Devic, F. Salles, S. Bourrelly, B. Moulin, G. Maurin, P. Horcajada, C. Serre, A. Vimont, J.-C. Lavalley, H. Leclerc, G. Clet, M. Daturi, P. L. Llewellyn, Y. Filinchuk and G. Ferey, J. Mater. Chem., 2012, 22, 10266–10273 RSC.
- K. Seki and W. Mori, J. Phys. Chem. B, 2002, 106, 1380–1385 CrossRef CAS.
- R. Kitaura, F. Iwahori, R. Matsuda, S. Kitagawa, Y. Kubota, M. Takata and T. C. Kobayashi, Inorg. Chem., 2004, 43, 6522–6524 CrossRef CAS PubMed.
- H. Chun, D. N. Dybtsev, H. Kim and K. Kim, Chem.–Eur. J., 2005, 11, 3521–3529 CrossRef CAS PubMed.
- D. Tanaka, M. Higuchi, S. Horike, R. Matsuda, Y. Kinoshita, N. Yanai and S. Kitagawa, Chem.–Asian J., 2008, 3, 1343–1349 CrossRef CAS PubMed.
- F. ZareKarizi, M. Joharian and A. Morsali, J. Mater. Chem. A, 2018, 6, 19288–19329 RSC.
- D. N. Dybtsev, H. Chun and K. Kim, Angew. Chem., Int. Ed., 2004, 43, 5033–5036 CrossRef CAS PubMed.
- K. Uemura, Y. Yamasaki, Y. Komagawa, K. Tanaka and H. Kita, Angew. Chem., Int. Ed., 2007, 46, 6662–6665 CrossRef CAS PubMed.
- K. Uemura, Y. Yamasaki, F. Onishi, H. Kita and M. Ebihara, Inorg. Chem., 2010, 49, 10133–10143 CrossRef CAS PubMed.
- J. S. Grosch and F. Paesani, J. Am. Chem. Soc., 2012, 134, 4207–4215 CrossRef CAS PubMed.
- J. Y. Lee, L. Pan, X. Huang, T. J. Emge and J. Li, Adv. Funct. Mater., 2011, 21, 993–998 CrossRef CAS.
- H. Miura, V. Bon, I. Senkovska, S. Ehrling, S. Watanabe, M. Ohba and S. Kaskel, Dalton Trans., 2017, 46, 14002–14011 RSC.
- M. Shivanna, Q.-Y. Yang, A. Bajpai, E. Patyk-Kazmierczak and M. J. Zaworotko, Nat. Commun., 2018, 9, 3080 CrossRef PubMed.
- P. Nugent, Y. Belmabkhout, S. D. Burd, A. J. Cairns, R. Luebke, K. Forrest, T. Pham, S. Q. Ma, B. Space, L. Wojtas, M. Eddaoudi and M. J. Zaworotko, Nature, 2013, 495, 80–84 CrossRef CAS PubMed.
- X. L. Cui, K. J. Chen, H. B. Xing, Q. W. Yang, R. Krishna, Z. B. Bao, H. Wu, W. Zhou, X. L. Dong, Y. Han, B. Li, Q. L. Ren, M. J. Zaworotko and B. L. Chen, Science, 2016, 353, 141–144 CrossRef CAS PubMed.
- S. K. Elsaidi, M. H. Mohamed, C. M. Simon, E. Braun, T. Pham, K. A. Forrest, W. Xu, D. Banerjee, B. Space, M. J. Zaworotko and P. K. Thallapally, Chem. Sci., 2017, 8, 2373–2380 RSC.
- L. Yang, X. Cui, Y. Zhang, Q. Yang and H. Xing, J. Mater. Chem. A, 2018, 6, 24452–24458 RSC.
- L. Carlucci, G. Ciani, D. M. Proserpio and A. Sironi, J. Chem. Soc., Chem. Commun., 1994, 2755–2756 RSC.
- M. J. Zaworotko, Chem. Soc. Rev., 1994, 23, 283–288 RSC.
- S. K. Elsaidi, M. H. Mohamed, L. Wojtas, A. Chanthapally, T. Pham, B. Space, J. J. Vittal and M. J. Zaworotko, J. Am. Chem. Soc., 2014, 136, 5072–5077 CrossRef CAS PubMed.
- E. J. Carrington, C. A. McAnally, A. J. Fletcher, S. P. Thompson, M. Warren and L. Brammer, Nat. Chem., 2017, 9, 882–889 CrossRef CAS PubMed.
- S. Galli, N. Masciocchi, V. Colombo, A. Maspero, G. Palmisano, F. J. López-Garzón, M. Domingo-García, I. Fernández-Morales, E. Barea and J. A. R. Navarro, Chem. Mater., 2010, 22, 1664–1672 CrossRef CAS.
- T. G. Mitina and V. A. Blatov, Cryst. Growth Des., 2013, 13, 1655–1664 CrossRef CAS.
- M. J. Zaworotko, Chem. Commun., 2001, 1–9 RSC.
- M. Fujita, Y. J. Kwon, S. Washizu and K. Ogura, J. Am. Chem. Soc., 1994, 116, 1151–1152 CrossRef CAS.
- J. Lu, T. Paliwala, S. C. Lim, C. Yu, T. Niu and A. J. Jacobson, Inorg. Chem., 1997, 36, 923–929 CrossRef CAS.
- K. Biradha, A. Mondal, B. Moulton and M. J. Zaworotko, J. Chem. Soc., Dalton Trans., 2000, 3837–3844 RSC.
- J. vanden Bergh, C. Gücüyener, E. A. Pidko, E. J. M. Hensen, J. Gascon and F. Kapteijn, Chem.–Eur. J., 2011, 17, 8832–8840 CrossRef CAS PubMed.
- D. Fairen-Jimenez, S. A. Moggach, M. T. Wharmby, P. A. Wright, S. Parsons and T. Düren, J. Am. Chem. Soc., 2011, 133, 8900–8902 CrossRef CAS PubMed.
- N. Lock, Y. Wu, M. Christensen, L. J. Cameron, V. K. Peterson, A. J. Bridgeman, C. J. Kepert and B. B. Iversen, J. Phys. Chem. C, 2010, 114, 16181–16186 CrossRef CAS.
- L. Sarkisov, R. L. Martin, M. Haranczyk and B. Smit, J. Am. Chem. Soc., 2014, 136, 2228–2231 CrossRef CAS PubMed.
- S. Bourrelly, P. L. Llewellyn, C. Serre, F. Millange, T. Loiseau and G. Férey, J. Am. Chem. Soc., 2005, 127, 13519–13521 CrossRef CAS PubMed.
- N. Rosenbach Jr, A. Ghoufi, I. Deroche, P. L. Llewellyn, T. Devic, S. Bourrelly, C. Serre, G. Ferey and G. Maurin, Phys. Chem. Chem. Phys., 2010, 12, 6428–6437 RSC.
- R. Demuynck, S. M. J. Rogge, L. Vanduyfhuys, J. Wieme, M. Waroquier and V. Van Speybroeck, J. Chem. Theory Comput., 2017, 13, 5861–5873 CrossRef CAS PubMed.
- R. Numaguchi, H. Tanaka, S. Watanabe and M. T. Miyahara, J. Chem. Phys., 2013, 138, 054708 CrossRef PubMed.
- M. Pera-Titus and D. Farrusseng, J. Phys. Chem. C, 2012, 116, 1638–1649 CrossRef CAS.
- A. Gonzalez-Nelson, F.-X. Coudert and M. A. van der Veen, Nanomaterials, 2019, 9, 330 CrossRef CAS PubMed.
- M. P. Suh, H. J. Park, T. K. Prasad and D.-W. Lim, Chem. Rev., 2012, 112, 782–835 CrossRef CAS PubMed.
- L. J. Murray, M. Dinca and J. R. Long, Chem. Soc. Rev., 2009, 38, 1294–1314 RSC.
- B. Li, H.-M. Wen, W. Zhou, J. Q. Xu and B. Chen, Chem, 2016, 1, 557–580 CAS.
- K. Sumida, D. L. Rogow, J. A. Mason, T. M. McDonald, E. D. Bloch, Z. R. Herm, T.-H. Bae and J. R. Long, Chem. Rev., 2012, 112, 724–781 CrossRef CAS PubMed.
- S. Kitagawa, Angew. Chem., Int. Ed., 2015, 54, 10686–10687 CrossRef CAS PubMed.
-
M. Bonneau, C. Lavenn, K. Sugimoto, A. Legrand, T. Ogawa, F.-X. Coudert, R. Réau, K.-i. Otake and S. Kitagawa, Research Square, 2020, DOI:10.21203/rs.3.rs-102861/v1.
- Y. Peng, V. Krungleviciute, I. Eryazici, J. T. Hupp, O. K. Farha and T. Yildirim, J. Am. Chem. Soc., 2013, 135, 11887–11894 CrossRef CAS PubMed.
- F. Gándara, H. Furukawa, S. Lee and O. M. Yaghi, J. Am. Chem. Soc., 2014, 136, 5271–5274 CrossRef PubMed.
- B. Li, H.-M. Wen, H. Wang, H. Wu, M. Tyagi, T. Yildirim, W. Zhou and B. Chen, J. Am. Chem. Soc., 2014, 136, 6207–6210 CrossRef CAS PubMed.
- J. A. Mason, M. Veenstra and J. R. Long, Chem. Sci., 2014, 5, 32–51 RSC.
- D. Alezi, Y. Belmabkhout, M. Suyetin, P. M. Bhatt, Ł. J. Weseliński, V. Solovyeva, K. Adil, I. Spanopoulos, P. N. Trikalitis and A.-H. Emwas, J. Am. Chem. Soc., 2015, 137, 13308–13318 CrossRef CAS PubMed.
- J. M. Lin, C. T. He, Y. Liu, P. Q. Liao, D. D. Zhou, J. P. Zhang and X. M. Chen, Angew. Chem., Int. Ed., 2016, 55, 4674–4678 CrossRef CAS PubMed.
- I. Spanopoulos, C. Tsangarakis, E. Klontzas, E. Tylianakis, G. Froudakis, K. Adil, Y. Belmabkhout, M. Eddaoudi and P. N. Trikalitis, J. Am. Chem. Soc., 2016, 138, 1568–1574 CrossRef CAS PubMed.
- Z. Chen, P. Li, R. Anderson, X. Wang, X. Zhang, L. Robison, L. R. Redfern, S. Moribe, T. Islamoglu, D. A. Gómez-Gualdrón, T. Yildirim, J. F. Stoddart and O. K. Farha, Science, 2020, 368, 297–303 CrossRef CAS PubMed.
-
Y. He, W. Zhou and B. Chen, Current status of porous metal–organic frameworks for methane storage, Metal–Organic Frameworks: Applications in Separations and Catalysis, Wiley-VCH, 2018, pp. 163–198 Search PubMed.
- Z. R. Herm, E. D. Bloch and J. R. Long, Chem. Mater., 2014, 26, 323–338 CrossRef CAS.
- B. Li, H. Wang and B. Chen, Chem.–Asian J., 2014, 9, 1474–1498 CrossRef CAS PubMed.
- B. Van de Voorde, B. Bueken, J. Denayer and D. De Vos, Chem. Soc. Rev., 2014, 43, 5766–5788 RSC.
- K. Adil, Y. Belmabkhout, R. S. Pillai, A. Cadiau, P. M. Bhatt, A. H. Assen, G. Maurin and M. Eddaoudi, Chem. Soc. Rev., 2017, 46, 3402–3430 RSC.
- S. Mukherjee, A. V. Desai and S. K. Ghosh, Coord. Chem. Rev., 2018, 367, 82–126 CrossRef CAS.
- H. Wang and J. Li, Acc. Chem. Res., 2019, 52, 1968–1978 CrossRef CAS PubMed.
- R.-B. Lin, S. Xiang, W. Zhou and B. Chen, Chem, 2020, 6, 337–363 CAS.
- D. S. Sholl and R. P. Lively, Nature, 2016, 532, 435–437 CrossRef PubMed.
- L. Li, Y. Wang, J. Yang, X. Wang and J. Li, J. Mater. Chem. A, 2015, 3, 22574–22583 RSC.
- L. Alaerts, M. Maes, L. Giebeler, P. A. Jacobs, J. A. Martens, J. F. M. Denayer, C. E. A. Kirschhock and D. E. De Vos, J. Am. Chem. Soc., 2008, 130, 14170–14178 CrossRef CAS PubMed.
- V. Finsy, C. E. A. Kirschhock, G. Vedts, M. Maes, L. Alaerts, D. E. De Vos, G. V. Baron and J. F. M. Denayer, Chem.–Eur. J., 2009, 15, 7724–7731 CrossRef CAS PubMed.
- V. Bon, N. Kavoosi, I. Senkovska and S. Kaskel, ACS Appl. Mater. Interfaces, 2015, 7, 22292–22300 CrossRef CAS PubMed.
- J.-P. Zhang, P.-Q. Liao, H.-L. Zhou, R.-B. Lin and X.-M. Chen, Chem. Soc. Rev., 2014, 43, 5789–5814 RSC.
- J. D. Evans, V. Bon, I. Senkovska, H.-C. Lee and S. Kaskel, Nat. Commun., 2020, 11, 2690 CrossRef CAS PubMed.
- T. D. Bennett, A. K. Cheetham, A. H. Fuchs and F.-X. Coudert, Nat. Chem., 2017, 9, 11–16 CrossRef CAS PubMed.
- X. Yang, H.-L. Zhou, C.-T. He, Z.-W. Mo, J.-W. Ye, X.-M. Chen and J.-P. Zhang, Research, 2019, 2019, 9463719 CrossRef CAS PubMed.
- S. Ehrling, E. M. Reynolds, V. Bon, I. Senkovska, T. E. Gorelik, J. D. Evans, M. Rauche, M. Mendt, M. S. Weiss, A. Pöppl, E. Brunner, U. Kaiser, A. L. Goodwin and S. Kaskel, Nat. Chem., 2021, 13, 568–574 CrossRef CAS PubMed.
- S. Chibani and F.-X. Coudert, APL Mater., 2020, 8, 080701 CrossRef CAS.
- G. Fraux and F.-X. Coudert, Chem. Commun., 2017, 53, 7211–7221 RSC.
- F.-X. Coudert, Phys. Chem. Chem. Phys., 2010, 12, 10904–10913 RSC.
- F. J. Sotomayor and C. M. Lastoskie, Microporous Mesoporous Mater., 2020, 292, 109371 CrossRef CAS.
- S. Darwish, S.-Q. Wang, D. M. Croker, G. M. Walker and M. J. Zaworotko, ACS Sustainable Chem. Eng., 2019, 7, 19505–19512 CrossRef CAS.
- N. Stock and S. Biswas, Chem. Rev., 2012, 112, 933–969 CrossRef CAS PubMed.
- N. Chanut, A. Ghoufi, M.-V. Coulet, S. Bourrelly, B. Kuchta, G. Maurin and P. L. Llewellyn, Nat. Commun., 2020, 11, 1216 CrossRef CAS PubMed.
- J. Su, S. Yuan, H.-Y. Wang, L. Huang, J.-Y. Ge, E. Joseph, J. Qin, T. Cagin, J.-L. Zuo and H.-C. Zhou, Nat. Commun., 2017, 8, 1–8 CrossRef CAS PubMed.
- H. Li and M. R. Hill, Acc. Chem. Res., 2017, 50, 778–786 CrossRef CAS PubMed.
- C. Jones, A. Tansell and T. Easun, J. Mater. Chem. A, 2016, 4, 6714–6723 RSC.
|
This journal is © The Royal Society of Chemistry 2021 |
Click here to see how this site uses Cookies. View our privacy policy here.