Pilot-scale removal of organic micropollutants and natural organic matter from drinking water using ozonation followed by granular activated carbon†
Received
15th October 2020
, Accepted 15th January 2021
First published on 22nd January 2021
Abstract
Conventional drinking water treatment is inefficient in removing a large fraction of known organic micropollutants (OMPs). Therefore more efficient treatment approaches are needed to limit exposure to OMPs via drinking water. Here, the OMP removal performance of a combination of ozonation/no ozonation and two types of granular activated carbon (GAC) was tested in a one-year pilot-scale study, alongside a study of full-scale treatment. The raw water was lake water with low ambient concentrations of OMPs. In total, 29 of 99 targeted OMPs (per- and polyfluoroalkyl substances (PFASs), pharmaceuticals and other OMPs) were detected (mean ∑OMPs = 57 ± 16 ng L−1). Only a few OMPs were consistently removed in the full-scale process, while ozonation in the pilot experiment effectively removed 72% of detected compounds to levels <30%. The GAC columns showed breakthrough of OMPs and dissolved organic carbon (DOC) for both ozonated and non-ozonated water, with earlier breakthrough for DOC than OMPs. Breakthrough of OMPs was delayed in ozonated columns, possibly because of lower adsorption competition with low-molecular-weight natural organic matter (NOM) fractions measured with liquid chromatography (LC-OCD). The OMP removal performance of the two GAC materials was not affected by greater DOC loading, but Filtrasorb showed 25% higher removal of DOC without losing capacity to remove OMPs. Compounds with low log
KOC tended to break through earlier than those with higher KOC values. The lowest levels of OMPs were observed in GAC effluents using ozonated feed water demonstrating the efficacy of combining ozone with GAC for managing OMP levels during drinking water production.
Water impact
Human exposure to organic micropollutants through contaminated raw water sources is getting increased attention. This study shows a complex and dynamic system treating environmentally relevant concentrations of micropollutants over the course of twelve months. Further, detailed data of the composition of the natural organic matter (NOM) contributes to the understanding of competition processes between different fractions of NOM and micropollutants.
|
1 Introduction
Water bodies are constantly exposed to various organic micropollutants (OMPs), such as pharmaceuticals, personal care products and industrial chemicals, particularly in urbanised and heavily populated areas with a high presence of industries and wastewater treatment plants.1–4 Drinking water producers face the challenge of choosing appropriate raw water for production of potable water, but are limited by local hydrogeological conditions, land use and legislation. Cost-efficient solutions for drinking water producers unavoidably mean using raw water with some degree of contamination, i.e. a mix of known and unknown potentially toxic substances of varying concentrations, often showing seasonal and other concentration fluctuations. It is likely that this challenge will continue or worsen,5 calling for improved or novel water treatment techniques. Due to rising awareness of potential health risks associated with intake of drinking water6 and a tendency for stricter regulations and guidelines,7,8 drinking water producers face increasing expectations to deliver water that has been treated with techniques known to be efficient in removal of OMPs. Most OMPs commonly targeted for and detected in raw water intended for use as drinking water are found at trace levels (pg L−1 to ng L−1 levels).9–12 Microbial contamination is a more tangible risk with more acute consequences; however, intake of multiple and different micropollutants via drinking water may still be a concern, as people consume up to several litres per day throughout their lives.13 Drinking water quality guidelines regulate allowed levels of targeted drinking water contaminants (e.g. ref. 14 and 15) but are limited to well-known OMPs such as pesticides and polyfluoroalkyl substances (PFASs). Although there are established toxicity assessment concepts and a constant development of health-related thresholds for chemicals in drinking water, chronic exposure to low doses of potentially hazardous individual and mixtures of OMPs may occur, while long-term effects of such exposure are poorly understood.16–18
Conventional treatment techniques for drinking water, such as coagulation, sedimentation and rapid or slow sand filtration, are primarily designed to remove bacteria, colour, odour and excess organic matter, but are not optimised for removal of OMPs.9,10,19–22 Many advanced treatment techniques are available on the market, such as advanced oxidation techniques, membrane filters with a range of pore size cut-offs and different activated carbon systems, all of which are proven to be effective in removing certain OMPs and natural organic matter (NOM).9,23–25 A risk with use of oxidation techniques, such as ozonation, is formation of hazardous persistent transformation products.26,27 However, whereas ozonation may add hazardous degradation products,27 granular activated carbon filters (GAC) generally remove hydrophobic compounds, including potentially hazardous OMPs, from the feed water. But as GAC materials have broad-spectrum adsorption properties, it is likely that a large fraction of transformation products in the feed water can be removed. These characteristics make combined ozonation and GAC filtration a promising, powerful and safe treatment of raw or partially treated water and is also one of the most promising tools for water reuse.28 As many transformation products have high polarity, making them relatively mobile and resulting in early breakthrough in GAC,27,29 it is particularly important to utilize new (or recently regenerated) GAC filter when used in combination with ozone. A large number of studies have explored how OMPs and dissolved organic carbon (DOC; a common proxy for quantification of NOM) behave and interact during ozonation and GAC filtration processes at different scales (laboratory-, pilot- and full-scale experiments).9,30–37,67,68 Recent studies on combined ozone and GAC treatments have mainly focused on biofiltration aspects and NOM removal (e.g. ref. 31–33 and 38), while Zietzschmann et al.40 studied the impact of ozonation on competition between OMPs and NOM in powdered activated carbon (PAC), and Boucherie et al.41 investigated synergistic treatment effects between ozonation and GAC in a pilot-scale experiment using water spiked with pharmaceuticals and pesticides. The great interest of ozonation in combination with GAC filtration as a future technology for safer drinking water also requires a deep understanding of how such a system works in a dynamic situation with natural raw water with low levels of various organic substances that vary in quality over time. This study evaluated the behaviour of OMPs and DOC in a long-term combined pilot-scale ozonation and GAC filtration treatment experiment, while also recording detailed data on NOM composition using liquid chromatography with organic carbon detection (LC-OCD) and ultraviolet (UV) absorbance. The aim was to provide detailed insights into how low, and therefore environmentally relevant, ambient concentrations behave in a complex and dynamic water treatment system. Specifically, the sorption dynamics of OMPs to GAC filter materials were studied in a pilot-scale column experiment run over a one-year period. The pilot plant was fed with water from one of Sweden's most important source waters (Lake Mälaren) that had been partially treated in a full scale drinking water treatment plant (by coagulation, flocculation, sedimentation/flotation and rapid sand filtration) and performance of combined ozonation and GAC filters (two different types) was compared with full-scale treatment. The OMPs analysed (n = 99) represented pharmaceuticals, personal care products, per- and polyfluoroalkyl substances (PFASs) and industrial chemicals. The primary objective was to determine how OMPs are removed by ozonation and GAC with respect to NOM adsorption competition, in a complex dynamic system with natural water and ambient low OMP concentrations.
2 Experimental
Material and methods
Full-scale treatment set-up and sampling.
The site for the experiment was Görväln drinking water treatment plant (DWTP), one of the primary drinking water producers in Sweden, with production of around 50 million m3 water annually and serving more than 600
000 people. The plant uses surface water from Lake Mälaren (two depths) and treatment steps in the full-scale facility comprise microsieving (250 μm), coagulation (Al2(SO4)3), flocculation, sedimentation, rapid sand filtration, biological activated carbon (BAC) filtration, UV disinfection and finally secondary disinfection by dosing with monochloramine and pH adjustment with lime, before distribution (Fig. 1). The full-scale treatment was sampled between May 2018 and July 2019 at the raw water intake, after coagulation, flocculation, sedimentation/flotation and rapid sand filtration (RFS), after one of the biological active GAC filters (BAC), and in the finished drinking water (DW) after additional UV disinfection and dosing of monochloramine and lime. Total organic carbon (TOC) data for the full scale BAC filter were only collected from March 2016 to August 2018 but, given the age of the filter material and the stable removal process, the values are comparable to the more recent data for the other full-scale TOC sampling points. The material in the sampled BAC filter (Fig. 1, point 3; Norit 830 W) had been in use for 14 years and was operated with a 5–6 min empty bed contact time (EBCT) and a surface load of 20–25 m h−1.
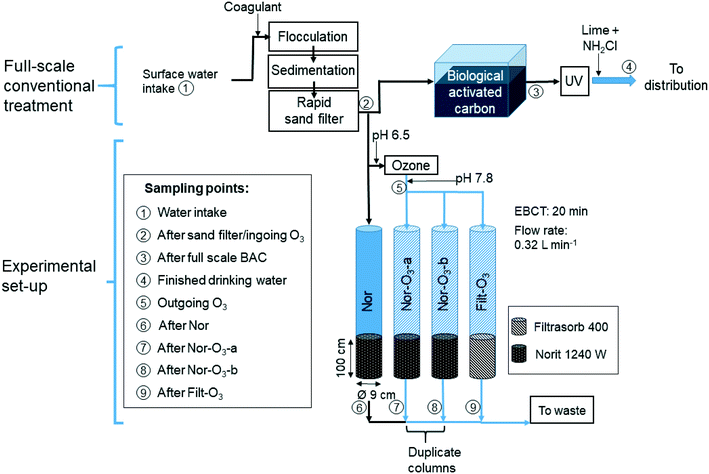 |
| Fig. 1 Schematic image of the full-scale plant and experimental columns. The material used in the full-scale biological activated carbon (BAC) filter was Norit 830 W. The rapid sand filtration (RSF) water fed to the treatment column (Nor) had pH 6.8. | |
Column experiment set-up and sampling.
The water used in the pilot-scale experiment was taken after the full-scale rapid sand filtration (Fig. 1, point 2), adjusted to pH 6.5 and then split into two different streams. One of these streams was ozonated and thereafter adjusted to pH 7.8, and the other stream (pH 6.5–6.8) was fed directly to a GAC column that was used as a reference column for the full-scale treatment (Nor). The pilot set-up (including the reference column) consisted of four parallel cylindrical columns (diameter 9 cm) filled with 6.4 L of pre-soaked GAC to a height of 1 m (Fig. 1). This set-up is partly the same as a previous study,42 which served as a pre-study to the current experiment. The water flow was 0.32 L min−1, fed from above, and the EBCT was 20 minutes for all columns. The columns were backwashed with permeate water (from ozonated columns), on average once a week, to prevent clogging and pressure build-up. Two columns (Nor-O3-a and Nor-O3-b) were set up as duplicate treatments, with the same feed water and active carbon material (Norit 1240 W). One column (Filt-O3) was filled with Filtrasorb 400, but otherwise operated under the same conditions as the duplicate Norit 1240 W columns. The non-ozonated column were also filled with Norit 1240 W. The experiment was conducted between May 2018 and July 2019. Sampling was performed weekly for TOC and DOC (in total 43 sampling occasions), and monthly for OMP and LC-OCD analysis (in total 12 sampling occasions). The samples for OMP, LC-OCD and TOC/DOC analysis were collected directly from taps into 1 L PP bottles, 40 mL clear glass vials and 0.5 L PET bottles, respectively, from a total of nine different sampling points: (point 1) at the raw water intake, (point 2) after RSF, (point 3) after full-scale BAC, (point 4) after full-scale disinfection and pH adjustment (UV disinfection + dosing of monochloramine and lime), (point 5) after ozonation and pH adjustment, and (point 6–9) after each of the four parallel pilot-scale GAC filters (Fig. 1). All samples for OMP analysis were kept cold (approximately 8 °C) during transport to the laboratory and stored in a refrigerator (8 °C) until extraction within 5 days or in a freezer (−18 °C) if extraction was conducted >5 days after sampling.
Activated carbon materials.
Two commonly used granulated activated carbon materials, Filtrasorb 400® and Norit 1240 W, were tested for their capacity to adsorb OMPs and DOC. Filtrasorb 400® (Calgon Carbon Corporation, Feluy, Belgium) is prepared from steam-activated bituminous coal that is pulverised and re-agglomerated. It has an effective particle size of 0.55–0.75 mm (D10), an average surface area 1050 m2 g−1 (Brunauer–Emmett–Teller (BET) method) and an iodine number of 1000 mg g−1. The Norit 1240 W material (Cabot Norit Nederland, Amersfoort, Netherlands) is also prepared from steam-activated bituminous coal and has an effective particle size 0.6–0.7 mm (D10), surface area 1100 m2 g−1 (BET), and iodine number 975 mg g−1. Both materials have a bed density (backwashed and drained) of 420 kg m−3. However, according to available fact sheets provided by the manufacturers, there seems to be a significant difference in pore size distribution, with Filtrasorb having a broader distribution of pores (micro- meso- and macropores) than Norit which is mainly microporous. The main differences between the two materials are thus the manufacturing process and the surface area, with the re-agglomerated Norit 1240 W having ∼10% higher surface area and a more microporous structure. The full-scale DWTP filter consisted of Norit 830 W (slightly coarser than 1240 W), which at the time of the study had been in use for 14 years.
Ozonation.
Ozone was generated with an ICT-10 generator (Ozonetech; Hägersten, Sweden), in which oxygen gas is concentrated from the surrounding air and ozone is generated through high-voltage electrical discharge. The ozone was mixed into the feed water in two consecutive steps; first with a minor stream of sand filtered water through an injection mixer and then mixed with the rest of the feed water. The ozonated water then passed through a 50 L reaction tank with a contact time of 4.2 minutes. The average ozone concentration (± standard deviation) directly after the reaction tank was 0.75 (± 0.2) mg L−1. After the initial reaction tank, the water was led through three further tanks (70 L, 63 L and 63 L) in series, in order to have the option to bypass water, although this alternative was not utilised. After ozonation, 40 mL duplicate samples were taken weekly to measure the residual O3, at the outlet of each tank. Each duplicate was analysed twice, using Ozone Accuvac 0.01–1.5 mg L−1 O3 ampules (Hach Lange, Colorado USA). Samples containing residual ozone (typically only outgoing water from ozonation; Table 1, point 5) were quenched immediately at sampling using 1 mL mg−1 O3 of 0.01 M sodium thiosulphate solution to prevent further degradation of OMPs or DOC in the samples.
Table 1 Average ingoing concentrations and removal efficiency by ozonation and removal efficiency in full scale DWTP process (raw water compared to finished drinking water) for individual compounds (n = 12; ± standard deviation). Sample points (see Fig. 1) in square brackets
Compound group |
Compound |
Average ingoing concentration (ng L−1) [2] |
Average removal efficiency by ozone (%) [between 2 to 5] |
Average removal efficiency by DWTP-process (%) months 1–6 [between 1 to 4] |
Average removal efficiency by DWTP-process (%) months 7–12 [between 1 to 4] |
Effluent water concentration was below LOQ, and LOQ/2 was used in the calculation.
Influent and effluent water concentrations were below LOQ.
|
Pharmaceuticals |
Carbamazepine |
7.3 ± 1.1 |
100 ± 0.4 |
13 ± 11 |
−15 ± 11 |
Lidocaine |
1.4 ± 0.4 |
99 ± 0.4a |
18 ± 8.2 |
−23 ± 30 |
Fexofenadine |
1.8 ± 0.4 |
99 ± 2.4 |
39 ± 14 |
−18 ± 20 |
Tramadol |
1.4 ± 1.1 |
99 ± 1.1a |
−1.9 ± 21 |
−857 ± 1155 |
Metoprolol |
2.1 ± 1.2 |
98 ± 1.0a |
43 ± 19 |
58 ± 35 |
O-desmethyl-venlafaxine |
2.6 ± 3.0 |
98 ± 2.0a |
11 ± 17 |
−293 ± 417 |
Clindamycin |
0.3 ± 0.1 |
98 ± 1.2a |
98 ± 1.2a |
98 ± 0.5 |
Cetirizine |
2.9 ± 0.6 |
98 ± 2.5 |
19 ± 10 |
−19 ± 20 |
Losartan |
1.2 ± 0.4 |
98 ± 1.4a |
31 ± 14 |
|
Citalopram |
0.2 ± 0.1 |
97 ± 1.6a |
30 ± 44 |
|
Propranolol |
0.1 ± 0.02 |
96 ± 0.4a |
46 ± 21 |
|
Mirtazapine |
0.1 ± 0.01 |
96 ± 1.3a |
46 ± 8.4 |
|
Venlafaxine |
1.4 ± 0.3 |
95 ± 2.0a |
10 ± 13 |
|
Irbesartan |
0.1 ± 0.1 |
93 ± 5.6a |
33 ± 29 |
27 ± 75 |
Trimethoprim |
0.8 ± 0.4 |
86 ± 12 |
45 ± 14 |
−11 ± 37 |
Diazepam |
0.03 ± 0.01 |
75 ± 13a |
16 ± 41 |
|
Oxazepam |
1.9 ± 0.4 |
73 ± 8.0 |
13 ± 6.4 |
−16 ± 8.7 |
Phenazone |
0.4 ± 0.2 |
72 ± 26 |
72 ± 26 |
−136 ± 229 |
Ifosfamide |
0.3 ± 0.04 |
71 ± 6.6a |
8.7 ± 35 |
|
Lamotrigine |
9.1 ± 1.5 |
58 ± 10 |
40 ± 19 |
14 ± 10 |
Bicalutamide |
1.7 ± 0.6 |
53 ± 11 |
19 ± 15 |
−12 ± 9.3 |
Fluconazole |
1.5 ± 0.5 |
43 ± 14 |
16 ± 10 |
−209 ± 262 |
|
PFASs |
PFOS |
2.5 ± 0.7 |
17 ± 29 |
0.6 ± 32 |
−83 ± 125 |
PFNA |
0.9 ± 0.2 |
8.1 ± 36 |
−44 ± 82 |
−47 ± 104 |
|
Other OMPs |
Tolyltriazole |
13 ± 6.9 |
97 ± 1.7 |
48 ± 8.4 |
5.9 ± 33 |
Caffeine |
22 ± 3.8 |
95 ± 5.1 |
15 ± 11 |
−46 ± 44 |
Sulisobenzone |
1.2 ± 0.5 |
87 ± 5.8a |
40 ± 44 |
8.7 ± 78 |
DEET |
1.9 ± 0.5 |
61 ± 12 |
16 ± 8.2 |
−19 ± 18 |
Tris(2-butoxylethyl) phosphate |
1.5 ± 0.6 |
60 ± 33 |
32 ± 8.9 |
|
Organic carbon.
TOC concentration was measured on unfiltered water samples using Multi N/C 3100 (Analytik Jena AG. Jena, Germany) and DOC concentration was measured using the same instrument, but after filtration with polypropylene filters (0.45 μm, rinsed with 120 mL MilliQ). The difference in organic carbon concentration between filtered and unfiltered samples was 0.1 mg L−1 for raw water and 0.04 mg L−1 for post-RSF samples. The difference for the latter was smaller than the precision of the instrument, i.e. negligible, justifying the use of DOC as proxy where applicable. The DOC concentration of the MilliQ-blank was 0.07 mg L−1. Ultraviolet absorbance at 254 nm was measured on filtered (0.45 μm PP-filters, rinsed with 120 mL MilliQ) water in 5 cm cuvettes, using a Hitachi U.1100 Spectrophotometer (Hitachi Ltd. Tokyo, Japan). Five fractions of TOC were measured monthly using size exclusion LC-OCD (Het Waterlaboratorium, Harleem, Netherlands). The fractions obtained were biopolymers (molecular weight ≫20
000 g mol−1), humic substances (∼1000 g mol−1), building blocks (300–500 g mol−1), neutrals (<350 g mol−1) and low-molecular-weight acids (<350 g mol−1).
Target analytes and analytical standards.
A set of 99 OMPs was selected for the chemical analysis (Table S1 in ESI†). These comprised painkillers (n = 6), antibiotics (n = 8), antidepressants (n = 8), other pharmaceuticals (n = 44), personal care products (n = 2), industrial chemicals (n = 5), PFASs (n = 13) and others (n = 13). For quantification and quality control, a mixture of individual mass-labelled internal standards was used (Table S1 in ESI†). For purity and other details of the reference compounds and internal standards, see Sörengård et al.3 Physico-chemical properties of the target compounds are given in Table S2 in ESI.† Molecular weight, topological polar surface area and aqueous solubility were obtained from PubChem, octanol–water partition coefficient (log
KOW) and normalised organic carbon to water partition coefficient (log
KOC) were calculated using EPI Suite (EPI Suite™ 4.11, US EPA), and distribution coefficient (log
D) (at pH = 7) was taken from Dürig et al.(2019).43 Log
KOC was calculated from the predicted log
KOW value.
Analysis of organic micropollutants (OMPs).
Sample preparation and analysis of the OMPs were performed on the dissolved aqueous phase as in Sörengård et al.3 In short, the samples were filtered through glass fibre filters. A volume of 500 mL of filtered sample was transferred to a 1 L PP bottle and then spiked with 50 ng of the internal standard mixture. Solid phase extraction (SPE) was performed using HLB cartridges (200 mg, Waters Oasis, MA, USA) and final extracts were analysed by a DIONEX UltiMate 3000 ultra-performance liquid chromatography (UPLC) system (Thermo Scientific, Waltham, MA, USA) coupled to a triple quadrupole mass spectrometer (MS/MS) (TSQ Quantiva, Thermo Fisher Scientific, Waltham, MA, USA). The MS data were evaluated using TraceFinder™ (Thermo Fisher Scientific, MA, USA).
Quality assurance/quality control.
A 10-point calibration curve from 0.01 ng mL−1 to 250 ng mL−1 was prepared and used for data evaluation. Limit of quantification (LOQ) was calculated as one quarter of the lowest calibration point in the calibration curve, where the relative standard deviation of the average response factor was <30% (all LOQs are given in Table S1 in ESI†). Fortified samples were prepared by spiking the water samples with native and internal standards before extraction (100 ng absolute per sample of native compounds and 50 ng absolute per sample of internal standards). The precision of the method was evaluated using duplicates for every 10th sample. The matrix effect was assessed for each OMP, and correction for ion suppression or ion enhancement was made using matrix-matched standards. Matrix-matched standards were prepared from water SPE extract (from raw water and after ozonation), which was spiked with internal standards and native compounds at concentration levels of 50 ng L−1 and 100 ng L−1, respectively. The matrix effect was assessed as the difference between the relative response factor of the matrix-matched standards and the average relative response factor obtained from the calibration curve.44 The blanks were prepared and extracted in the same way as the samples. Method blanks (n = 2 per batch, each comprising 14 samples) consisted of MilliQ water and methanol 1/1 (v/v). No target analytes were detected in the method blanks.
Calculations.
The removal efficiency of the different OMPs during ozonation was calculated as: | 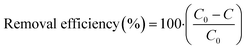 | (1) |
where C0 is the feed water concentration and C the concentration after ozonation. Where effluent concentrations were below LOQ, LOQ/2 were used. Breakthrough of OMPs in the GAC columns was calculated as: | 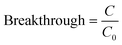 | (2) |
where C is the concentration after GAC filtration and C0 is the feed water concentration. Breakthrough of a compound was defined as when the compound was continuously detected in outgoing water. Full breakthrough was defined as when >95% of the compounds slipped through. The number of bed volumes (BV) was calculated as: |  | (3) |
where t is the time from start to sampling (h), r is the flow rate (mL h−1) and Vadsorbent is the volume of the adsorbent (mL). Empty bed contact time (EBCT) was calculated as: | 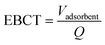 | (4) |
where Vadsorbent is the volume of the bed (L) and Q is the flow (L day−1).
The DOC load (g) was calculated as the difference in concentration (C0–C in g L−1) times the flow rate (L d−1) divided by the time between sampling occasions (days). The removed DOC load is a measure of the cumulative amount of DOC that is sequestered by the GAC material, over a certain time, including both adsorption and biological degradation. DOC can be removed by GAC in different ways, mainly by adsorption and biological degradation. Initially, when starting up a GAC filter, adsorption will be the dominant process, dictated by the abundance of available adsorption sites, physical straining and clogging of pores.45 Over time, accumulation of attached biomass on the surface of the grains inevitably forms a biofilm, a process that is rapid in the beginning and slows down as the filter reaches maturity. In a study with a similar set up, this rapid accumulation phase lasted 90 days.45 Eventually the GAC surface is no longer able to adsorb effectively (i.e. has reached saturation). After this, biological degradation becomes more important and is likely the dominating process.45–47
3 Results and discussion
Removal of OMP and TOC in full-scale treatment
In total, 29 of the targeted 99 OMPs were detected in the raw water on two or more occasions of the 12 sampling occasions over the 12-month period (Table S5 in ESI†). The average (± standard deviation) concentration ranged from 0.03 ± 0.01 ng L−1 (diazepam) to 13 ± 6.2 ng L−1 (tolyltriazole) and amounted to an average sum of 57 ± 16 ng L−1 for the 29 compounds. There was a seasonal trend in the OMP levels in the raw water, with higher levels during summer and lower in winter (Fig. S1 and S2 in ESI†). The seasonal trends in OMP concentrations in the same raw water are described in our recent study.48 They are suggested to be due to hydrological processes in the lake basin, lake stratification in late autumn and the fact that the summer of 2018 was unusually warm and dry (high evaporation), with mean temperature in May–July being 3.4 °C higher than the average for the previous 30-year period (Fig. S3 in ESI†).49 During the full-scale treatment study, it was evident that neither total concentrations of the studied OMPs nor any of the OMP groups was removed to a great extent in any of the treatment steps (Fig. 2A). The GAC material in the full-scale filter (Fig. 1, point 3) had not been replaced or reactivated for 14 years at the time of sampling, and the EBCT was short (∼5–6 min). The GAC filter was thus principally acting as a BAC filter at the time of sampling, mainly protecting against taste and odour compounds, which are known to be effectively reduced in drinking water by either fresh or mature biologically active GAC filters.42,50 These results agree well with previous findings of no or low removal efficiencies of OMPs in the same DWTP.10,42 Other studies have found removal of OMPs in conventional DWTPs to be highly variable and compound-specific22,42,51,52 and, in the case of GAC filters, highly dependent on GAC age, with longer run time resulting in poorer removal efficiency of most OMPs.9 However, it is clear that the bulk of commonly analysed OMPs generally have low removal efficiency in DWTPs lacking treatment processes such as membrane filtration (nanofiltration or reverse osmosis), fresh or newly reactivated GAC or powdered active carbon (PAC) or oxidation, i.e. using only conventional treatment techniques.9,51,52 Another study conducted on the same DWTP as in the present study tested effect-based methods (in vitro cell-based bioassays) and concluded that the bioactivity (used as an indicator for toxicity) was low, but persisted during the treatment process.53
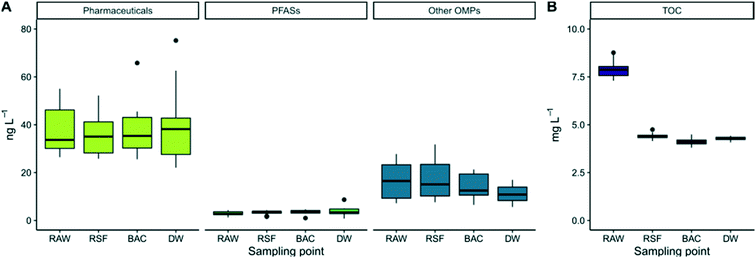 |
| Fig. 2 Boxplots in A showing sum concentrations of organic micropollutants (OMPs) detected in the full-scale process: pharmaceuticals (22 compounds, n = 12), per- and polyfluoroalkyl substances (PFASs) (2 compounds, on n = 12 occasions), others (4 compounds, n = 12, caffeine excluded) and boxplot B showing total organic carbon concentration (TOC) in: raw water intake, n = 51; after rapid sand filtration (RSF), n = 51; after full-scale BAC filter, n = 46, sampled between 1 March 2016 and 7 August 2018; and finished drinking water (DW), n = 62. | |
Only four of the 29 compounds detected showed relatively consistent removal between water intake and finished drinking water (Fig. 1, point 1–4) over the whole sampling period (Table 1 and Table S3 in ESI†). Clindamycin was reduced to below the detection limit (>96 ± 2% removal), which primarily occurred after the GAC filter, presumably by UV-irradiation or by monochloramine oxidation. Lamotrigine was removed by 27% (±19%), metoprolol by 51% (±27%) and tolyltriazole by 27% (±30%). Twelve of the 29 OMPs showed an unexpected seasonal pattern in their removal rate, with relatively consistent removal (on average 22 ± 11%) during the first six months of the experiment (July–December), followed by negative removal (on average −21%) in the last six months (January–June) (Fig. S2 and Table S3 in ESI†), indicating desorption of previously adsorbed chemicals. No major changes were made in the treatment process at the time of the shifting dynamics, and the basic water chemistry data (internal DWTP data) were relatively consistent, including the incoming concentration of DOC (relative standard deviation; RSD 2.7%). However, the water temperature and DOC composition showed seasonal trends, with raw water temperatures in the 7–15 °C range the first six months of the experiment and 0.5–8 °C for the last six (Fig. S4 in the ESI†), and a slight dip in the concentration of the humic substances fraction occurred during the midpoint of the experiment. Another factor to consider is lake stratification with lake turnover occurring in November possibly leading to a new mix of water both from above and below the intake depth. Such temperature ranges changes could promote sorption/desorption processes since sorption equilibria are related to temperature.54,55,69 Further investigations of seasonal trends observed in the full-scale system were beyond the scope of this work.
In contrast to the OMPs, ∼50% of TOC was removed during the coagulation, flocculation, sedimentation and rapid sand filtration steps (Fig. 1, point 1 and 2, from 7.9 ± 0.4 mg L−1 to 4.3 ± 0.1 mg L−1) (Fig. 2d). The subsequent steps (Fig. 1, point 3 and 4, BAC filtration, UV-radiation and monochloramine addition) did not further remove TOC (Fig. 2d). A study assessing NOM removal in DWTPs in three Nordic capitals found similar removal efficiencies for similar treatment processes (chemical precipitation, sedimentation, filtration and disinfection processes), namely 55% (Oslo), 48% (Stockholm DWTP using the same raw water reservoir as in this study) and 76% (Helsinki).56
Removal of NOM fractions by ozonation
Removal of NOM fractions by ozonation was studied in detail, as the treatment is known to change NOM composition and consequently may affect sorption/desorption to adsorbents such as GAC. The sum concentration of NOM (which closely corresponds to DOC), as measured by LC-OCD, did not show a large relative decrease, but still decreased significantly, from 4.21 ± 0.09 mg L−1 to 4.06 ± 0.10 mg L−1 (Welch two-sample t-test, p = 0.0013), which corresponds to an average decrease of 0.15 ± 0.07 mg L−1 or 3.6% (see Table S3 in ESI†). LC-OCD analyses showed that the major fraction of the removed DOC (0.10 mg L−1) consisted of humic substances (∼1000 g mol−1) and the remaining (0.05 mg L−1) of neutrals (∼350 g mol−1), while low-molecular-weight (LMW) acids (<350 g mol−1) were generally not detected in either water. The removal rates in our study are in line with findings in other studies that removal (mineralisation) of TOC/DOC by ozonation is limited. Other studies have found that the biodegradable fraction (neutrals and LMW acids) tends to increase after ozonation, but this was not apparent in our study.39,57,58 A comparison of breakthrough of DOC and sum of LC-OCD fractions is displayed in Fig. S5 in the ESI.†
Formation of smaller NOM molecules during ozonation is likely to lead to increased adsorption competition between NOM constituents and OMPs.59 Large humic compounds tend to bind more efficiently to GAC due to their higher hydrophobicity, which in turn may lead to blocking of smaller adsorbent pores. In contrast, smaller NOM components may directly bind to internal pores and decrease the removal efficiency of smaller OMPs.59 As the humic substances fraction was reduced in this study without smaller molecules being formed (indicating mineralisation), low adsorption competition between NOM constituents and OMPs in the GAC filters could be expected in the experimental pilot-scale columns using ozonated waters.
Ultraviolet absorption at 254 nm (UV) decreased by on average 49% (±6%) after ozonation, indicating a pronounced reduction in NOM aromaticity. Since lower NOM aromaticity leads to reduced adsorption competition,60,61 this observation also indicates lowered adsorption competition for ozonated waters in the GAC columns.
Removal of OMPs by ozonation
As ozonation is a destructive treatment technique that may remove target compounds but also create stable transformation products, the concept “removal of OMPs” by ozonation may be misleading. In the following, it should be kept in mind that when we refer to removal, we only consider the target compounds, while potential co-existing transformation products are not considered. Table 1 shows average removal efficiency of the detected OMPs by the ozonation treatment over the 12-month experimental period (Fig. 1, between point 2 and 5, water subsequently fed to columns Nor-O3-a, Nor-O3-b and Filt-O3). Thirteen of the 29 OMPs detected (citalopram, clindamycin, ifosfamide, irbesartan, lidocaine, losartan, metoprolol, mirtazapine, propranolol, tramadol, venlafaxine and O-desmethyl-venlafaxine) were degraded to levels below LOQ. Concentrations of an additional six substances (carbamazepine, fexofenadine, tolyltriazole, cetirizine, caffeine and sulisobenzone) decreased by more than 90%. These data agree well with findings in similar studies. However, generally, reaction kinetics are highly dependent on many experimental parameters such as water matrix, initial pollutant concentrations, concentration of competing reactants and reactor dimensions. It is therefore difficult to compare reaction kinetics observed in this study with previous studies experiencing different experimental conditions.62 For example, Huber et al.63 found that the reactivity of carbamazepine is high and argued that it would be completely transformed during ozonation. A study by Lee et al.32 tested ozonation at different doses for wastewater treatment and found that DEET was removed by 25% (±8.9%), trimethoprim by 83% (±19%) and carbamazepine by 82% (±19%) from 10 different wastewaters when using a dose in the same order of magnitude as ours. Perfluorooctane sulfonate (PFOS) and perfluorononanoic acid (PFNA) were not removed during ozonation in our experiment, and their concentrations were occasionally even higher in the water leaving the ozonation contact tanks than in incoming water. This could be due to that levels of PFOS and PFNA were close to LOQ and therefore measured with relatively high uncertainty. The C–F bond is highly stable and for ozonation to be effective, it needs to be in conjunction with catalysts and higher reaction doses than in this set-up, a direction that cannot be recommended because of other limiting factors such as bromate formation and decreased biostability.64,65 If PFAS levels are of concern, which they were not in this case, the proposed treatment could be e.g. fresh activated carbon, anion exchange resin or nano-membranes.66
Breakthrough NOM in GAC columns
Many previous studies have used only DOC and/or UV absorbance to characterise DOC (e.g. ref. 27 and 65). The LC-OCD measurements in our study enabled more detailed characterisation of this diverse and heterogeneous compound group. Fig. 3A shows breakthrough (C/C0) curves of DOC for the different experimental columns, where the GAC columns fed with ozonated sand filtrate (Nor-O3-a/b and Filt-O3) showed slower breakthrough, and a slightly higher consistent removal for the second half of the experiment than the GAC column fed with sand filtrate without ozonation. Fig. 3C shows breakthrough (C/C0) curves for the total NOM fractions derived from LC-OCD analysis as a function of throughput (BV). A value close to zero means that the feed water had a higher concentration than the outgoing water, a value of 1.0 indicates complete breakthrough and values above 1.0 mean that the outgoing water had a higher concentration than the feed water, indicating e.g. desorption or biological production/release or chemical desorption of certain DOC fractions within the column. LC-OCD data were not recorded for the duplicate column, Nor-O3-b.
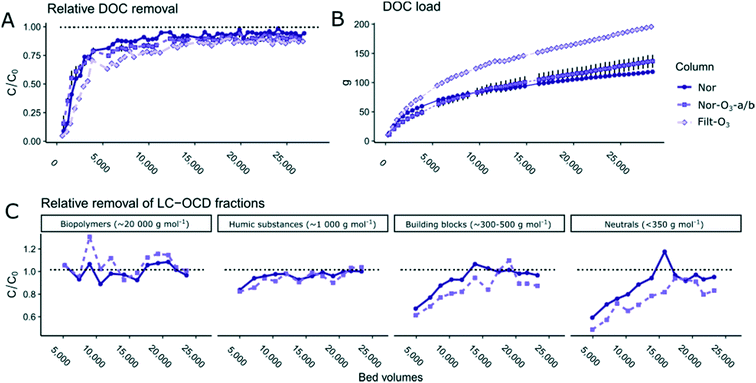 |
| Fig. 3 A. Relative breakthrough of DOC per column, B. cumulative amount [g] of DOC load removed, and C. breakthrough curves of LC-OCD fractions over the Norit 1240 W columns with ozonation (Nor-O3-a) and without ozonation (Nor). Low molecular weight (LMW) acids were close to method detection limit and therefore excluded from the diagram. All figures (A–C) are plotted as a function of throughput (bed volumes). Error bars represent standard deviation of duplicate columns. Dotted line represents full breakthrough (=1). | |
The biopolymer fraction (≫20
000 g mol−1) showed complete breakthrough already at the time of the first sampling (2800 BV) and the humic substances (∼1000 g mol−1) reached complete breakthrough (C/C0 = 0.96) at some point between 7700 and 9600 BV, for both Nor-O3-a and Nor columns. The building blocks fraction (∼300–500 g mol−1) showed complete breakthrough between 12
700 and 14
000 BV in the Nor column, but maintained removal throughout the experiment in Nor-O3-a. The neutrals fraction (<350 g mol−1) showed the greatest difference between the columns.
Breakthrough of OMPs in GAC columns
The sum concentrations of detected OMPs in effluent from the four pilot-scale GAC columns (Nor, Nor-O3 (duplicate) and Filt-O3) are shown by compound group as a function of BV in Fig. 4A. The inflow concentrations of the individual OMPs were varying over time (RSD 115% for non-ozonated water and 10–69% for ozonated water). As the variation in OMP concentrations in inflow water did not show a consistent time trend for all compounds detected and as several compounds decreased to levels below LOQ in the effluent water, visualisation of the breakthrough using the sum concentrations over time was considered a better option than using the treatment efficiency, which would lead to many missing values.
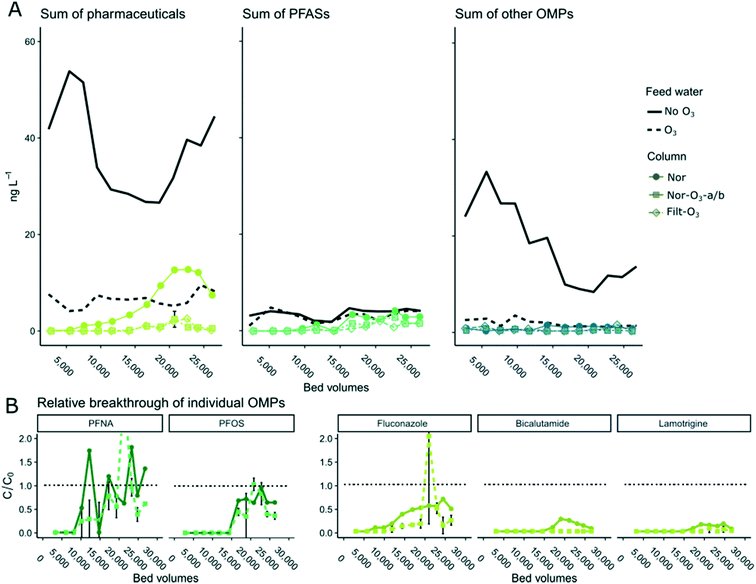 |
| Fig. 4 A. Sum concentrations (ng L−1) of detected pharmaceuticals (22 compounds) in GAC effluents, per- and polyfluoroalkyl substances (PFASs) (2 compounds) and other organic micropollutants (OMPs) (4 compounds), alongside influent concentrations and B. breakthrough curves of five selected OMPs. Where effluent concentrations were below LOQ, “0” is shown in the figures. All graphs are plotted as a function of throughput (bed volumes). Error bars represent standard deviation of duplicate columns. Caffeine was excluded from the sum concentration. Dotted lines represent full breakthrough (=1). The spike in PFNA at 20 700 BV (not displayed in the graph) was 3.0 ng L−1. | |
For most OMP groups in all columns, the breakthrough pattern showed a pulse shape, with a peak and then lower concentrations at the end of the experiment. This pattern was seen only in the GAC effluents (and not in ozonated and non-ozonated feed waters) as temporary breakthrough values >1. The recovery in OMP removal capacity towards the end of the experiment might be due to a shift in binding mechanisms, possibly explained by OMPs binding to sorbed NOM molecules on the GAC grains or displacement processes within the pore systems. It could also be due to a chromatographic roll-off, meaning that adsorbed analytes are being re-introduced to the water as a result of an unobserved inflow concentration pulse or change in temperature, just as observed in the full scale DWTP process. Sorption/desorption of DOC and OMPs in systems using natural water or other DOC-containing water is complex and may lead to changes in performance and dynamics over time, as demonstrated in previous studies (e.g. ref. 53, 65, 66 and 70). The throughput volume at which the bulk of OMPs started breaking through in the columns receiving ozonated water was similar (around 16
000 BV). However, for the non-ozonated column, pharmaceuticals started breaking through earlier, at around 7500 BV (Fig. 4A).
Adsorption competition
Comparing Fig. 3A with Fig. 4, it is clear that there was earlier breakthrough of DOC and later breakthrough of all OMPs for all compound groups (PFASs, pharmaceuticals and other OMPs) for GAC filtering both ozonated and non-ozonated feed water. With the ozonated water containing fewer large humic molecules (without measurable formation of LMW compounds), more polar compounds and less aromatic NOM (indicated by a large decrease in UV absorption), the adsorption competition can be expected to be smaller than for non-ozonated water, presumably leading to the later breakthrough. In addition, it is possible that differences in total OMP concentrations in the experimental waters affected the adsorption site competition in favour of more efficient adsorption when using ozonated water. However, it should be kept in mind that differences in total OMP concentrations may be apparent, as transformation products are formed in the ozonation process, but were not targeted and are not included in the total OMP levels.
The neutrals fraction, with molecular weights in the same range as the OMPs studied in this experiment, showed the strongest retention, with values of 78–90% after 26
000 BV. The column receiving ozonated water (Nor-O3-a) maintained a higher removal rate over time for both NOM and OMPs, which suggests less adsorption competition between OMPs and weakly adsorbable NOM fractions (mainly neutrals). An explanation for this could be more available pores due to less pore blocking from smaller/less UV-absorbing compounds. To evaluate the adsorption competition between NOM fractions and OMPs, the top five most breakdown persistent OMPs in the ozonation treatment were chosen (see Table 1) for comparison. These were effectively fed to the columns at similar concentration levels.
Breakthrough of these compounds (C/C0) are shown in Fig. 4B. For fluconazole and lamotrigine, later breakthrough was seen in the experiment using ozonated water (6300 vs. 7500 BV later, respectively). For bicalutamide, no breakthrough occurred in the ozonated GAC effluents, whereas breakthrough occurred between 14
200 and 16
700 BV in the non-ozonated GAC effluents. The breakthrough patterns for PFOS and PFNA were irregular and not clear, likely due to concentrations close to LOQ both in incoming and outgoing water. Our overall observations support the hypothesis that ozone treatment decreases adsorption competition by NOM, and therefore enables longer run time of the GAC filter with respect to OMPs. A recent study arrived at a similar conclusion for 2-methylisoborneol adsorption to PAC in ozonated water.61
Comparison of GAC types
When only considering sum concentrations of OMPs, start time of breakthrough and number of BV, there were no evident differences between the Norit and Filtrasorb activated carbon materials (Fig. 4A). On the other hand, when considering removal of DOC, the Filtrasorb material performed better in the initial part of the experiment (up to 5000 BV), which is evident as an initially flatter slope of DOC concentrations compared with Norit (both ozonated and non-ozonated; Fig. 3A). On plotting the cumulative amount (g) of removed DOC against BV (Fig. 3B), it was clear that the Filtrasorb column removed more DOC than Norit (169 g vs. 118 ± 5 g at the time of breakthrough at 16
500 BV), without losing capacity to remove OMPs, as well as a higher total amount of DOC during the experiment (206 g vs. 146 ± 6 g). A portion of this DOC, especially toward the second half of the experiment, is likely due to biological degradation rather than adsorption to GAC particles. The advantage of Filtrasorb cannot be explained by a difference in average surface area per weight unit, as this is similar or even lower (approx. 10%) in Filtrasorb than in Norit. Instead, other surface characteristics, such as the distribution of nano-, micro- and mesopores, appear to be of importance for the difference. Other studies46,71 have identified the amount of pores in the 5–10 nm range (lower end of mesopores) as most important for DOC removal. Filtrasorb has more pores in this range than Norit, which might explain the observed differences in our results. Organic matter competes with OMPs adsorption sites on the GAC surface, in ways that are not trivial to predict.35 Our study suggests that this competition is less effective for Filtrasorb 400 than for Norit 1240 W, implying that a Filtrasorb 400 filter can be operated for a longer time before replacement or regeneration. This result supports findings in a previous study on the same source water of higher removal rate of DOC for the Filtrasorb 400 material.42
Time to breakthrough as a function of log
Koc
A better understanding of the relationship between physico-chemical properties of individual OMPs and their sorption/desorption behaviour towards GAC can help in prediction of breakthrough patterns and is of value in risk management processes, where it is important to understand which compounds might slip through from source to tap.8 Sorption characteristics are commonly assessed with partitioning coefficients, such as the distribution coefficient (D) and the octanol–water partitioning coefficient (Kow), which describe hydrophobicity, and the partitioning coefficient for organic carbon and water (Koc), which commonly is calculated as a function of Kow. All these partitioning coefficients thus describe the partitioning between octanol/organic carbon and pure water. They do not describe any other relevant additional interactions, in our case e.g. interaction with counter-ions in the water and with ionisable or ionic sites on the GAC surface. Log
Koc has recently been suggested to be a conservative but preferred descriptor of compound mobility in the environment.4,8,72 In the present case examining the adsorption of GAC filters, it was also the most relevant parameter. A heat map plot (Fig. 5) was used to investigate the relationship between log
KOC of individual OMPs and their breakthrough (C/C0) in this study over time (BVs treated). The data for the non-ozonated GAC effluents were used, as it contained the highest levels (and presumably the lowest uncertain values), and only OMPs that were detected in the feed water on >50% of sampling occasions were included. As seen in Fig. 5, there was a weak trend of earlier GAC filter breakthrough for compounds with lower log
KOC (i.e. weaker adsorption and lower affinity towards organic carbon). As stated earlier, it is challenging to predict mobility based on a partition coefficient because of the complexity in molecular interactions. Besides limitations in the prediction model, it should also be kept in mind that the water used here was natural, and contained low levels of OMPs, and that other KOC prediction methods than that used here may produce different results.
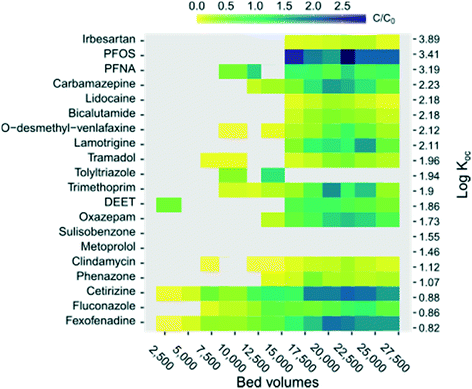 |
| Fig. 5 Relationship between bed volumes, breakthrough and log Koc for the non-ozonated column (Nor in Fig. 1). Grey tiles represent effluent concentrations <LOQ. | |
4 Conclusions
We found no overall systematic removal of OMPs in the current conventional treatment process except for four of 29 OMPs detected (metoprolol, lamotrigine, tolyltriazole and clindamycin), which were consistently removed throughout the year. Ozonation was shown to be an efficient measure to reduce the concentrations of a wide range of micropollutants, with reduction >70% for most OMPs (21 of 29 compounds), but ozone treatment only reduced DOC concentration by around 4%. The reduction in DOC concentration was mainly attributed to decreasing levels of humic substances and the neutral NOM fractions, as measured by LC-OCD. Breakthrough of OMPs occurred on average later in ozonated GAC effluents. This difference could be due to decreased adsorption competition by NOM, as UV-absorbance data showed decreased aromaticity by ozonation. NOM fractions broke through the GAC columns in the order of the size fractions, with the smaller fraction emerging last. We found no tangible difference between OMP breakthrough patterns of Filtrasorb 400 and Norit 1240 W under the same operating conditions. However, Filtrasorb showed 25% higher cumulative removal of DOC without losing the capacity to remove OMPs. Compounds with low KOC tended to break through earlier than those with higher KOC. We conclude that if OMPs and particularly substances unreactive to ozone (e.g. PFASs) are present in the raw water at a DWTP, an efficient post-treatment (fresh GAC in this context) is important for the final water quality, although the targeted OMP concentrations measured in this study do not point towards a need for action at this specific DWTP. Future research should investigate novel, cost-efficient treatment techniques for PFASs and desorption patterns in GAC filters and throughout the whole DWTP process over time, the role of biodegradation within GAC/BAC filters, both for OMPs and NOM as well as the effect on human health of chronic exposure of low concentrations of OMP.
Conflicts of interest
There are no conflicts to declare.
Acknowledgements
This work was funded by the Formas; Research Council for Environment, Agricultural Sciences and Spatial Planning (contract number 201600681), and municipal water treatment company Norrvatten (the pilot set-up, analyses, experimental planning and practical work with the pilot). The authors would like to thank Daniel Malnes for technical support.
Notes and references
- E. Archer, B. Petrie, B. Kasprzyk-hordern and G. M. Wolfaardt, Chemosphere The fate of pharmaceuticals and personal care products (PPCPs), endocrine disrupting contaminants ( EDCs ), metabolites and illicit drugs in a WWTW and environmental waters, Chemosphere, 2017, 174, 437–446 CrossRef CAS
.
- B. Kasprzyk-Hordern, R. M. Dinsdale and A. J. Guwy, The removal of pharmaceuticals, personal care products, endocrine disruptors and illicit drugs during wastewater treatment and its impact on the quality of receiving waters, Water Res., 2008, 43, 363–380 CrossRef
.
- M. Sörengård, H. Campos-Pereira, M. Ullberg, F. Y. F. Y. Lai, O. Golovko and L. Ahrens, Mass loads, source apportionment, and risk estimation of organic micropollutants from hospital and municipal wastewater in recipient catchments, Chemosphere, 2019, 234, 931–941 CrossRef
.
- T. Reemtsma, U. Berger, H. P. H. Arp, H. Gallard, T. P. Knepper and M. Neumann,
et al., Mind the Gap: Persistent and Mobile Organic Compounds - Water Contaminants That Slip Through, Environ. Sci. Technol., 2016, 50(19), 10308–10315 CrossRef CAS
.
- R. P. Schwarzenbach, B. I. Escher, K. Fenner, T. B. Hofstetter, C. A. Johnson and U. von Gunten,
et al., The Challenge of Micropollutants in Aquatic Systems, Science, 2006, 313(5790), 1073–1077 CrossRef
.
- J. L. Domingo and M. Nadal, Human exposure to per- and polyfluoroalkyl substances (PFAS) through drinking water: A review of the recent scientific literature, Environ. Res., 2019, 177, 108648 CrossRef CAS
.
-
Water JPI Knowledge Hub, Water JPI Policy Brief on Contaminants of Emerging Concern – an emerging risk in our waters, 2019 Search PubMed
.
-
M. Neumann and I. Schliebner, Protecting the sources of our drinking water: The criteria for identifying persistent, mobile and toxic (PMT) substances and very persistent and very mobile (vPvM) substances under EU Regulation REACH (EC). Dessau-Roßlau, 2019.
- R. Tröger, S. J. Köhler, V. Franke, O. Bergstedt and K. Wiberg, A case study of organic micropollutants in a major Swedish water source – Removal efficiency in seven drinking water treatment plants and influence of operational age of granulated active carbon filters, Sci. Total Environ., 2020, 706, 135680 CrossRef
.
- R. Tröger, P. Klöckner, L. Ahrens and K. Wiberg, Micropollutants in drinking water from source to tap - Method development and application of a multiresidue screening method, Sci. Total Environ., 2018, 627, 1404–1432 CrossRef
.
- M. F. Rahman, S. Peldszus and W. B. Anderson, Behaviour and fate of perfluoroalkyl and polyfluoroalkyl substances (PFASs) in drinking water treatment: A review, Water Res., 2014, 50, 318–340 CrossRef CAS
.
- P. Falås, H. R. Andersen, A. Ledin and J. La Cour Jansen, Occurrence and reduction of pharmaceuticals in the water phase at Swedish wastewater treatment plants, Water Sci. Technol., 2012, 64(4), 783–791 CrossRef
.
-
EFSA, Scientific Opinion on Dietary Reference Values for water, EFSA J, 2010 Mar 23, vol. 8, (3), Available from: DOI:10.2903/j.efsa.2010.1459
.
-
The council of the European Union, Position of the Council at first reading with a view to the adoption of a DIRECTIVE OF THE EUROPEAN PARLIAMENT AND OF THE COUNCIL on the quality of water intended for human consumption (recast) 6230/20. Vol. 6230/20. Brussels, 2020.
-
US EPA, National Primary Drinking Water Regulations, 2009, [cited 2021 Jan 5], Available from: https://www.epa.gov/sites/production/files/2016-06/documents/npwdr_complete_table.pdf Search PubMed
.
- M. I. Vasquez, A. Lambrianides, M. Schneider, K. Kümmerer and D. Fatta-Kassinos, Environmental side effects of pharmaceutical cocktails: What we know and what we should know, J. Hazard. Mater., 2014, 279, 169–189 CrossRef CAS
.
- S. Webb, T. Ternes, M. Gibert and K. Olejniczak, Indirect human exposure to pharmaceuticals via drinking water, Toxicol. Lett., 2003, 142(3), 157–167 CrossRef CAS
.
- T. Backhaus, Medicines, shaken and stirred: a critical review on the ecotoxicology of pharmaceutical mixtures, Philos. Trans. R. Soc., B, 2014, 369, 20130585 CrossRef
.
- P. E. Stackelberg, J. Gibs, E. T. Furlong, M. T. Meyer, S. D. Zaugg and R. L. Lippincott, Efficiency of conventional drinking-water-treatment processes in removal of pharmaceuticals and other organic compounds, Sci. Total Environ., 2007, 377(2), 255–272 CrossRef CAS
.
- M. Sillanpää, M. C. Ncibi, A. Matilainen and M. Vepsäläinen, Removal of natural organic matter in drinking water treatment by coagulation: A comprehensive review, Chemosphere, 2018, 190, 54–71 CrossRef
.
- S. Chan, K. Pullerits, J. Riechelmann, K. M. Persson, P. Rådström and C. J. Paul, Monitoring biofilm function in new and matured full-scale slow sand filters using flow cytometric histogram image comparison (CHIC), Water Res., 2018, 138, 27–36 CrossRef CAS
.
- H. Ren, R. Tröger, L. Ahrens, K. Wiberg and D. Yin, Screening of organic micropollutants in raw and drinking water in the Yangtze River Delta, China, Environ. Sci. Eur., 2020, 32(1), 67 CrossRef CAS
.
- T. Lin, S. Yu and W. Chen, Occurrence, removal and risk assessment of pharmaceutical and personal care products (PPCPs) in an advanced drinking water treatment plant (ADWTP) around Taihu Lake in China, Chemosphere, 2016, 152(Supplement C), 1–9 CrossRef CAS
.
- S. J. Köhler, E. Lavonen, A. Keucken, P. Schmitt-Kopplin, T. Spanjer and K. Persson, Upgrading coagulation with hollow-fibre nanofiltration for improved organic matter removal during surface water treatment, Water Res., 2016, 89, 232–240 CrossRef
.
- C. Teodosiu, A. F. Gilca, G. Barjoveanu and S. Fiore, Emerging pollutants removal through advanced drinking water treatment: A review on processes and environmental performances assessment, J. Cleaner Prod., 2018, 197, 1210–1221 CrossRef CAS
.
- J. Pohl, O. Golovko, G. Carlsson, J. Eriksson, A. Glynn and S. Örn,
et al., Carbamazepine Ozonation Byproducts: Toxicity in Zebrafish (Danio rerio) Embryos and Chemical Stability, Environ. Sci. Technol., 2020, 54, 2913–2921 CrossRef CAS
.
- A. Sharma, J. Ahmad and S. J. S. Flora, Application of advanced oxidation processes and toxicity assessment of transformation products, Environ. Res., 2018, 167, 223–233 CrossRef CAS
.
- P. Du Pisani and J. G. Menge, Direct potable reclamation in Windhoek: A critical review of the design philosophy of new Goreangab drinking water reclamation plant, Water Sci. Technol.: Water Supply, 2013, 13(2), 214–226 CAS
.
- M. Bourgin, B. Beck, M. Boehler, E. Borowska, J. Fleiner and E. Salhi,
et al., Evaluation of a full-scale wastewater treatment plant upgraded with ozonation and biological post-treatments: Abatement of micropollutants, formation of transformation products and oxidation by-products, Water Res., 2018, 129, 486–498 CrossRef CAS
.
- H. Sontheimer, E. Heilker, M. R. Jekel, H. Nolte and F. H. Vollmer, The Mülheim Process, J. - Am. Water Works Assoc., 1978, 70(7), 393–396 CrossRef CAS
.
- A. M. Kennedy, A. M. Reinert, D. R. U. Knappe, I. Ferrer and R. S. Summers, Full- and pilot-scale GAC adsorption of organic micropollutants, Water Res., 2015, 68, 238–248 CrossRef CAS
.
- Y. Lee, D. Gerrity, M. Lee, A. E. Bogeat, E. Salhi, S. Gamage, R. A. Trenholm, E. C. Wert, S. A. Snyder and U. von Gunten,
et al., Prediction of Micropollutant Elimination during Ozonation of Municipal Wastewater Effluents: Use of Kinetic and Water Specific Information, Environ. Sci. Technol., 2013, 47, 5872–5881 CrossRef CAS
.
- S. Jamil, P. Loganathan, A. Listowski, J. Kandasamy, C. Khourshed and S. Vigneswaran, Simultaneous removal of natural organic matter and micro-organic pollutants from reverse osmosis concentrate using granular activated carbon, Water Res., 2019, 155, 106–114 CrossRef CAS
.
- C. Corwin and R. S. Summers, Controlling trace organic contaminants with GAC adsorption, J. - Am. Water Works Assoc., 2012, 104, E36–E47 CrossRef
.
- F. Zietzschmann, C. Stützer and M. Jekel, Granular activated carbon adsorption of organic micro-pollutants in drinking water and treated wastewater – Aligning breakthrough curves
and capacities, Water Res., 2016, 92, 180–187 CrossRef CAS
.
- J. C. Crittenden, D. W. Hand, H. Arora and B. W. Lykins, Design considerations for GAC treatment of organic chemicals, J. - Am. Water Works Assoc., 1987, 79(1), 74–82 CrossRef CAS
.
- K. Yapsakli and F. Çeçen, Effect of type of granular activated carbon on DOC biodegradation in biological activated carbon filters, Process Biochem., 2010, 45(3), 355–362 CrossRef CAS
.
- J. Lohwacharin, A. Phetrak, S. Takizawa, Y. Kanisawa and S. Okabe, Bacterial growth during the start-up period of pilot-scale biological activated carbon filters: Effects of residual ozone and chlorine and backwash intervals, Process Biochem., 2015, 50, 1640–1647 CrossRef CAS
.
- Y. Zhang, X. Zhao, X. Zhang and S. Peng, A review of different drinking water treatments for natural organic matter removal, Water Sci. Technol.: Water Supply, 2015, 15(3), 442–455 CAS
.
- F. Zietzschmann, R. L. Mitchell and M. Jekel, Impacts of ozonation on the competition between organic micro-pollutants and effluent organic matter in powdered activated carbon adsorption, Water Res., 2015, 84, 153–160 CrossRef CAS
.
- C. Boucherie, C. Lecarpentier, N. Fauchon, M. Djafer and V. Heim, “Ozone” and “GAC filtration” synergy for removal of emerging micropollutants in a drinking water treatment plant?, Water Sci. Technol., 2010, 10(5), 860–868 CAS
.
- O. Golovko, L. de Brito Anton, C. Cascone, L. Ahrens, E. Lavonen and S. J. Köhler, Sorption Characteristics and Removal Efficiency of Organic Micropollutants in Drinking Water Using Granular Activated Carbon (GAC) in Pilot-Scale and Full-Scale Tests, Water, 2020, 12(2053), 1–14 Search PubMed
.
- W. Dürig, R. Tröger, P. L. Andersson, A. Rybacka, S. Fischer and K. Wiberg,
et al., Development of a suspect screening prioritization tool for organic compounds in water and biota, Chemosphere, 2019, 222, 904–912 CrossRef
.
- A. Borik, A. Vojs Stanova, R. Kodesova, B. W. Brooks, K. Grabicova and P. Novakova,
et al., Ultrafast laser diode thermal desorption method for analysis of representative pharmaceuticals in soil leachate samples, Talanta, 2020, 208, 120382 CrossRef CAS
.
- S. Velten, M. Boller, O. Köster, J. Helbing, H.-U. Weilenmann and F. Hammes, Development of biomass in a drinking water granular active carbon (GAC) filter, Water Res., 2011, 45(19), 6347–6354 CrossRef CAS
.
- S. Velten, D. R. U. Knappe, J. Traber, H.-P. Kaiser, U. von Gunten and M. Boller,
et al., Characterization of natural organic matter adsorption in granular activated carbon adsorbers, Water Res., 2011, 45(13), 3951–3959 CrossRef CAS
.
- O. Gibert, B. Lefèvre, M. Fernández, X. Bernat, M. Paraira and M. Calderer,
et al., Characterising biofilm development on granular activated carbon used for drinking water production, Water Res., 2013, 47(3), 1101–1110 CrossRef CAS
.
- A. L. Rehrl, O. Golovko, L. Ahrens and S. Köhler, Spatial and seasonal trends of organic micropollutants in Sweden's most important drinking water reservoir, Chemosphere, 2020, 1, 249 Search PubMed
.
-
A. Moberg, Stockholm Historical Weather Observations — Daily mean air temperatures since 1756. Dataset version 2.0. Bolin Centre Database., 2020
.
- R. Srinivasan and G. A. Sorial, Treatment of taste and odor causing compounds 2-methyl isoborneol and geosmin in drinking water: A critical review, J. Environ. Sci., 2011, 23(1), 1–13 CrossRef CAS
.
- Y. Yang, Y. S. Ok, K.-H. Kim, E. E. Kwon and Y. F. Tsang, Occurrences and removal of pharmaceuticals and personal care products (PPCPs) in drinking water and water/sewage treatment plants: A review, Sci. Total Environ., 2017, 596–597(Supplement C), 303–320 CrossRef CAS
.
- C. F. Couto, L. C. Lange and M. C. S. Amaral, Occurrence, fate and removal of pharmaceutically active compounds (PhACs) in water and wastewater treatment plants—A review, J. Water Process. Eng., 2019, 32, 100927 CrossRef
.
- A. K. Rosenmai, J. Lundqvist, T. le Godec, Å. Ohlsson, R. Tröger and B. Hellman,
et al., In vitro bioanalysis of drinking water from source to tap, Water Res., 2018, 139, 272–280 CrossRef CAS
.
- G. Aschermann, L. Neubert, F. Zietzschmann and M. Jekel, Impact of different DOM size fractions on the desorption of organic micropollutants from activated carbon, Water Res., 2019, 161, 161–170 CrossRef CAS
.
- J. T. Alexander, F. I. Hai and T. M. Al-aboud, Chemical coagulation-based processes for trace organic contaminant removal: Current state and future potential, J. Environ. Manage., 2012, 111, 195–207 CrossRef CAS
.
- P. Krzeminski, C. Vogelsang, T. Meyn, S. J. Köhler, H. Poutanen and H. A. de Wit,
et al., Natural organic matter fractions and their removal in full-scale drinking water treatment under cold climate conditions in Nordic capitals, J. Environ. Manage., 2019, 241, 427–438 CrossRef CAS
.
- A. A. Yavich, K. H. Lee, K. C. Chen, L. Pape and S. J. Masten, Evaluation of biodegradability of NOM after ozonation, Water Res., 2004, 38(12), 2839–2846 CrossRef CAS
.
-
J. C. Crittenden, R. Rhodes Trussel, D. W. Hand, K. J. Howe and G. Tchobanoglous, MWH's water treatment: principles and design, John Wiley & Sons, 2012, p. 1901 Search PubMed
.
- G. Newcombe, J. Morrison, C. Hepplewhite and D. R. U. Knappe, Simultaneous adsorption of MIB and NOM onto activated carbon II, Competitive effects, Carbon, 2002, 40(12), 2147–2156 CrossRef CAS
.
- I. Lerman, Y. Chen, B. Xing and B. Chefetz, Adsorption of carbamazepine by carbon nanotubes: Effects of DOM introduction and competition with phenanthrene and bisphenol A, Environ. Pollut., 2013, 182, 169–176 CrossRef CAS
.
- Q. Wang, F. Zietzschmann, J. Yu, R. Hofman, W. An and M. Yang,
et al., Projecting competition between 2-methylisoborneol and natural organic matter in adsorption onto activated carbon from ozonated source waters, Water Res., 2020, 173, 115574 CrossRef CAS
.
- J. Gomes, R. Costa, R. M. Quinta-Ferreira and R. C. Martins, Application of ozonation for pharmaceuticals and personal care products removal from water, Sci. Total Environ., 2017, 586, 265–283 CrossRef CAS
.
- M. M. Huber, S. Canonica, G.-Y. Park and U. Gunten, Oxidation of Pharmaceuticals during Ozonation and Advanced Oxidation Processes, Environ. Sci. Technol., 2003, 37, 1016–1024 CrossRef CAS
.
- K. H. Kucharzyk, R. Darlington, M. Benotti, R. Deeb and E. Hawley, Novel treatment technologies for PFAS compounds: A critical review, J. Environ. Manage., 2017, 204, 757–764 CrossRef CAS
.
- V. Franke, M. D. Schäfers, J. J. Lindberg and L. Ahrens, Removal of per- And polyfluoroalkyl substances (PFASs) from tap water using heterogeneously catalyzed ozonation, Environ. Sci.: Water Res. Technol., 2019, 5(11), 1887–1896 RSC
.
- D. Lu, S. Sha, J. Luo, Z. Huang and X. Zhang, Treatment train approaches for the remediation of per- and polyfluoroalkyl substances (PFAS): A critical review, J. Hazard. Mater., 2020, 386, 121963 CrossRef CAS
.
- D. N. Kothawala, S. J. Köhler, A. Östlund, K. Wiberg and L. Ahrens, Influence of dissolved organic matter concentration and composition on the removal efficiency of perfluoroalkyl substances (PFASs) during drinking water treatment, Water Res., 2017, 121(Supplement C), 320–328 CrossRef CAS
.
- P. McCleaf, S. Englund, A. Östlund, K. Lindegren, K. Wiberg and L. Ahrens, Removal efficiency of multiple poly- and perfluoroalkyl substances (PFASs) in drinking water using granular activated carbon (GAC) and anion exchange (AE) column tests, Water Res., 2017, 120(Supplement C), 77–87 CrossRef CAS
.
- G. Aschermann, F. Zietzschmann and M. Jekel, Influence of dissolved organic matter and activated carbon pore characteristics on organic micropollutant desorption, Water Res., 2018, 133, 123–131 CrossRef CAS
.
- J. Gustavsson, L. Ahrens, M. A. Nguyen, S. Josefsson, D. Berggren Kleja and K. Wiberg, Influence of natural organic matter on the extraction efficiency of flame retardants from surface waters, J. Chromatogr. A, 2017, 1524(Supplement C), 74–86 CrossRef CAS
.
- D. M. Golea, P. Jarvis, B. Jefferson, G. Moore, S. Sutherland and S. A. Parsons,
et al., Influence of granular activated carbon media properties on natural organic matter and disinfection by-product precursor removal from drinking water, Water Res., 2020, 174, 115613 CrossRef CAS
.
-
F. Kalberlah, J. Oltmanns, M. Schwarz, J. Baumeister and A. Striffler, Guidance for the precautionary protection of raw water destined for drinking water extraction from contaminants regulated under REACH. Dessau, 2014.
Footnote |
† Electronic supplementary information (ESI) available. See DOI: 10.1039/d0ew00933d |
|
This journal is © The Royal Society of Chemistry 2021 |