Algae-laden water treatment with ultrafiltration: effects of moderate oxidation by Fe(II)/permanganate on hydraulically irreversible fouling and deposition of iron and manganese oxides†
Received
10th September 2020
, Accepted 23rd October 2020
First published on 23rd October 2020
Abstract
Application of ultrafiltration (UF) in algae-laden water treatment is hindered by severe fouling and low retention of soluble organics. In this study, moderate oxidation by Fe(II)/permanganate was investigated as a pretreatment for algae-laden water before UF in comparison with permanganate oxidation alone and Fe(II) coagulation alone. Filtration tests were performed to assess the impacts of moderate oxidation on fouling reversibility, fouling mechanisms and compositions of the fouling layers. The results showed that Fe(II)/permanganate substantially alleviated the flux reduction by 65% and reduced reversible resistances by nearly 2 orders of magnitude, because the agglomerates formed by foulants and in situ formed iron and manganese oxides were larger in size than the algal cells, resulting in more porous fouling layers. The fouling mechanism shifted from the dual mechanism of successive pore blocking and cake filtration to single standard blocking over the whole duration investigated. However, irreversible fouling was aggravated by Fe(II)/permanganate, because the cake filtration was weakened and the adhesion of EOM on the membrane was aggravated in the presence of in situ formed iron and manganese oxides. The iron and manganese oxides deposited in the external fouling layer rather than inside the membrane. The amounts of Fe and Mn in the fouling layer substantially increased by more than 4 times with Fe(II)/permanganate than with permanganate or Fe(II). Fe(II)/permanganate increased the removal of EOM (67%) by UF, attributing the enhanced adsorption of EOM to both the membrane and the agglomerates.
Water impact
Algae-laden water treatment is a challenge in the water industry and ultrafiltration can effectively retain algal cells and some algal organics. However, membrane fouling is an obstacle. This study investigated a moderate oxidation strategy using Fe(II)/permanganate for fouling control and emphasis was placed on fouling reversibility, fouling mechanism and Fe/Mn deposition. This work may help to promote the application of UF in algae-laden water treatment.
|
1. Introduction
Low-pressure membrane filtration, in particular ultrafiltration (UF), has been increasingly applied in potable water treatment to fill the gap between more stringent water quality standards and the deteriorating water environment. UF membranes, which have a pore size less than 0.02 μm, can retain particles, colloids, protozoa (3–15 μm), bacteria (0.5–10 μm) and virus (0.02–0.08 μm), substantially increasing the safety of drinking water.1,2 Likewise, UF is efficient in removing various algae which is ubiquitous in eutrophic lakes and reservoirs. Algal cells can be completely retained and more than 45% of algal organics can be excluded from produced water.3,4 However, membrane fouling caused by algal cells and by organic matter excreted from the cells boost the energy consumption and shorten the lifespan of membranes.4–6 Hence, application of UF for algae-laden water treatment is significantly hindered by membrane fouling.
Membrane fouling during algae-laden water treatment using UF has been extensively investigated and both algal cells and extracellular organic matter (EOM) are identified as culprits for the fouling. Algal cells deposit on the membrane surface and a thick and compressible fouling layer is formed, resulting in a sharp decrease in membrane permeability.7–9 Even worse is that EOM, which is comprised of proteins and polysaccharides and has broad distributions of molecular weight (MW) and heterogeneity in hydrophilicity, can strongly adhere to the membrane, resulting in severe irreversible fouling.4,10,11 Membrane fouling by algae is typically governed by a dual mechanism with pore blocking and cake filtration successively dominating the fouling process.9 To overcome the fouling problem, a variety of pretreatments has been investigated such as coagulation, adsorption and oxidation. In contrast, oxidation, which is capable of inactivating algal cells and degrading algal metabolites, is much more attractive than other pretreatments, because low retention of soluble metabolites is another disadvantage of algae-laden water treatment by UF.
Performance of oxidation pretreatment in fouling control depends on both oxidant types and doses. Chlorination, which is typically used for disinfection, is capable of inactivating algae and reducing membrane fouling, especially with coagulation.12 Ozone, which is a very strong oxidant, proves to be efficient in not only fouling mitigation but also degradation of algal metabolites. Wei et al.13,14 have demonstrated that ozonation, either pre-dosed or in situ dosed, substantially decreased membrane fouling during algae-laden water treatment with ceramic UF membranes via selective oxidation of EOM, especially macromolecular fractions. In recent years, free radical-based oxidation pretreatments have also been used to alleviate fouling caused by algae. Pretreatments using UV/H2O2 and UV/Cl2, in which hydroxyl radicals are generated, can simultaneously reduce membrane fouling and degrade toxins and disinfection by-product precursors during algae-laden water treatment by UF.15,16 Moreover, stronger oxidation with persulfate radicals, which are generated by activated persulfate (PS) and peroxymonosulfate (PMS) with ultraviolet (UV) or Fe(II), were also used to pretreat algae-laden water, and superb performance was observed in both fouling control and degradation of EOM.17–19 However, every coin has two sides. Strong oxidation can necessarily aggravate the risk of algal cell breakage and release of intracellular organics and toxins, especially under over-dosed circumstances.20 For instance, a quick and complete loss of viability was observed for Microcystis aeruginosa and Anabaena flosaquae after ozone exposure of 0.2 mg min L−1.21 In the UV/Cl2 process, the intact cells decreased by 52% in 6 min at a chlorine dose of 0.2 mg L−1 and completely disappeared at a chlorine dose of 2.0 mg L−1.22 In the presence of sulfate radicals, the raptured cells took a ratio of 25% even at a low PMS dose of 50 μM.23 In contrast, permanganate, which is a moderate oxidant, imposes less oxidant stress to algal cells; more than 95% of Microcystis cells would maintain cell integrity as the concentration of KMnO4 did not exceed 2 mg L−1.24
To overcome the problem of cell breakage caused by oxidation pretreatments, a concept of moderate oxidation has been proposed for algae-laden water treatment. Permanganate is a typical moderate oxidant and proves to be effective in inactivating algal cells.25 The combination of permanganate and Fe(II), in which hydrous manganese dioxide and ferric oxides are in situ formed, achieves a balance between efficient algae removal and cell breakage. In situ formed Fe(III) is very strong in activity and is able to simultaneously strengthen organic adsorption and control of residual coagulants.26,27 Moreover, the flocs formed during coagulation of algae-laden water are rigid and readily precipitate in the presence of hydrous manganese dioxide.28 Regarding fouling caused by algae, permanganate oxidation was demonstrated to improve membrane permeability and fouling reversibility via reinforcing aggregation of cells and interception of EOM in the fouling layer, especially when the generation of hydrous manganese dioxide was enhanced by some reducing reagents. Ma et al.29 have investigated the performance of moderate oxidation by Fe(II)/permanganate in alleviating membrane fouling during filtration of natural algae-laden water, and found that the increase in transmembrane pressure (TMP) was only 25.1 kPa over a long duration (90 d) at a Fe(II)/permanganate dose of 6/18 μmol, much less than that with Fe(III) coagulation only (42.8 kPa). Moreover, it is concluded that in situ formed Fe(III) and manganese dioxide play a significant role in algae flocculation and fouling control. However, the impacts of Fe(II)/permanganate on fouling caused by algae are not systematically assessed with respect to fouling reversibility and mechanisms yet. Moreover, the membrane is usually covered by a brown layer due to deposition of iron and manganese oxides.30 The reversibility of the fouling layer and its impact on organic retention are still unclear.
In this study, moderate oxidation by Fe(II)/permanganate was investigated as a pretreatment for algae-laden water before UF in comparison with permanganate oxidation alone and Fe(II) coagulation alone. Impacts of pretreatments were compared in terms of fouling characteristics and organic rejections. The objective was to reveal the mechanisms for changes in membrane fouling and organic rejection in the presence of in situ formed iron and manganese oxides.
2. Materials and methods
2.1 Algae culture
The algae-laden water was prepared with lab-cultured Microcystis aeruginosa, which is one of the most commonly occurring and problematic algae species in eutrophic lakes and reservoirs. The algae seed (PCC7820) was purchased from the Institute of Hydrobiology, Chinese Academy of Sciences. The algae culture was performed in a self-built incubator with BG-11 medium under lab conditions, of which the composition is shown in Table S1 in the ESI.† A water bath, in which culture flasks were placed, was used to provide a constant temperature of 25 °C for algae growth. Moreover, an illumination at 5000 lx was provided by a fluorescent lamp for 14 h every day. The algae were harvested in the stationary phase with a culture time of 35 d. For the oxidation experiments and filtration tests, the harvested algae samples were diluted to a concentration of 2.0 × 106 cells per mL. The dilution factor was calculated according to the optical density at 685 nm (OD685), and the detailed instruction could be found in the ESI.† The diluent contained CaCl2 (0.5 mM), NaHCO3 (1.0 mM), and NaClO4 (15.0 mM) to simulate the natural ionic strength of an aquatic environment.
2.2 Oxidation processes
The chemical reagents, i.e., permanganate (KMnO4) and ferrous sulfate (FeSO4·7H2O), were of analytical grade and provided by Guangzhou Chemicals Co. Ltd (Guangzhou, China). Stock solutions were prepared with Milli-Q water and the concentrations of permanganate and ferrous sulfate were 10 mmol and 30 mmol, respectively. The oxidation was performed in a jar-test reactor (MY6000-6F, Meiyu, Wuhan, China) with 6 beakers (1 L). Mixing was provided by an electromotive stirrer in each beaker. In consideration of cell integrity and residual manganese, permanganate and Fe(II) were dosed at a stoichiometric ratio of 1
:
3.29 The dose ranges were 10–50 μmol and 30–150 μmol for permanganate and Fe(II), respectively. The typical range of ferric coagulants for algae-laden water is 1–15 mg Fe per L.31 The Fe(II) dose in this study varied in the range of 1.7–8.4 mg Fe per L. Three types of pretreatments, i.e. permanganate oxidation alone, Fe(II) coagulation alone and combined Fe(II)/permanganate, were investigated. For the permanganate oxidation, the algae-laden water was mixed for 10 min at 300 rpm after adding permanganate at the predetermined doses. For the Fe(II) coagulation, dual-stage mixing was used with fast mixing (300 rpm) for 3 min and slow mixing (100 rpm) for 7 min. For the combined Fe(II)/permanganate, the reagents were simultaneously added into the algae-laden water and then successively mixed at 300 rpm for 3 min and at 100 rpm for 7 min. After the pretreatment, 400 mL algae-laden water was filled into the UF cell for filtration tests.
2.3 Filtration tests and fouling assessment
The flat sheet UF membrane used in this work was purchased from Mosu Co. Ltd (Shanghai, China). The UF membrane was made of polyether sulfone (PES) and had a molecular weight cut-off (MWCO) of 100 kDa. The effective area of the membrane was 4.5 × 10−3 cm2. The UF membrane exhibited strong hydrophilicity with a contact angle of 56°. The new membranes were rinsed to remove protective reagents (glycerin) by filtering Milli-Q water until the dissolved organic carbon (DOC) in permeate was less than 0.2 mg L−1. The filtration test was performed with a lab-built UF system as shown in Fig. 1. The UF system consisted of a nitrogen gas cylinder to provide a constant pressure, 2 filtration cells (Amicon 8400, Millipore, USA), and 2 electronic balances linked to a computer to automatically log weight data every 5 seconds. The filtration was performed in a dead-end filtration mode.
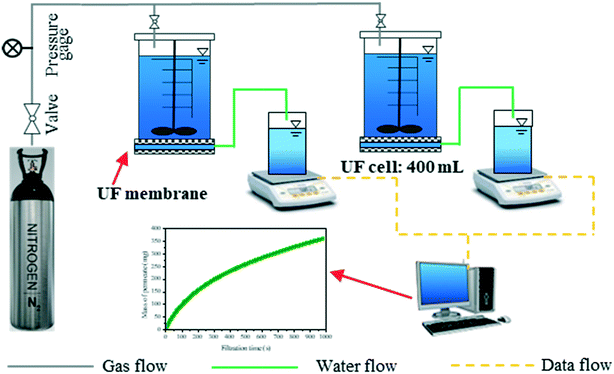 |
| Fig. 1 A schematic diagram of the bench-scale UF system. | |
In each filtration test, 400 mL algae-laden water was fed and the filtration was terminated as the permeate volume reached 350 mL. The initial flux of the UF membranes used varied in the range of 180–300 L m−2 h−1. The normalized flux J/J0 as a function of time was used to evaluate membrane fouling, with J0 representing the initial membrane flux. The UF membrane was placed at the bottom of the cell, and the feed water was driven through the membrane at a constant pressure (60 kPa). To assess fouling reversibility, hydraulic cleaning was performed with the membranes turned over and flushed for 2 min with Milli-Q water in the cell at a pressure of 100 kPa. The reversible fouling was defined as the fouling which could be removed by hydraulic backwashing, and the remnant fouling was regarded as the irreversible fouling.32 No stirring was provided during filtration. The filtration tests were conducted in duplicate. The resistance-in-series model (eqn (1)) was used to calculate the different types of fouling resistances.
| 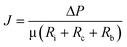 | (1) |
where
J is the permeate flux (L m
−2 h
−1), Δ
P is the operating pressure (Pa),
μ is the dynamic viscosity (Pa·s),
Ri is the intrinsic membrane resistance (m
−1),
Rc is the reversible resistance (m
−1), and
Rb is the irreversible resistance (m
−1).
To determine the intrinsic membrane resistance, 300 mL Milli-Q water was filtered to obtain the initial flux (J0). During algae-laden water filtration, the flux at the end of the filtration was denoted as J1. After the hydraulic cleaning, filtration of 300 mL Milli-Q water was performed again to determine the flux (J2). Finally, the reversible and irreversible resistances were obtained viaeqn (2) and (3).
|  | (2) |
|  | (3) |
To analyze fouling mechanisms, a combined model proposed in ref. 33 was used to analyze the filtration data. The J/J0 data versus time, t, plot was fitted to eqn (1)via non-linear optimization using the curve fitting tool in MATLAB. To determine the dominant mechanism in the different filtration phases, d2t/dV2versus dt/dV curves of all the filtration data were plotted, and the dominant mechanism was identified according to the following equation. Specific instructions could be found elsewhere.34,35
| 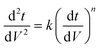 | (4) |
where
t is the filtration time,
V is the total filtered volume, and
n characterizes the filtration mechanism, with
n = 0 for cake filtration,
n = 1 for intermediate blocking,
n = 1.5 for standard blocking, and
n = 2 for complete pore blocking.
36 The required derivatives in
eqn (4) were evaluated in terms of the filtrate flux.
2.4 Analytical methods and fouling assessment
Zeta potentials were evaluated using a Zetasizer Nano S90 (Marvin instruments Ltd, Worcestershire, UK). Particle size distributions of algal cells and their agglomerates were studied using a laser particle analyzer (BT-9300S, Better Instruments Ltd, Liaoning, China). DOC concentrations of water samples were measured using a total organic carbon analyzer (TOC-L, Shimadzu, Tokyo, Japan). A linear range of 0–20 mg L−1 (R2 = 0.999) was obtained via calibration with potassium hydrogen phthalate. The ultraviolet absorbance at 254 nm (UV254), which was also adopted to assess organic removal, was determined using a UV spectrometer (T6, Puxi Instruments Ltd, Beijing, China). The samples were filtered through a 0.45 μm cellulose filter to remove suspended particles before the measurements. Moreover, a fluorescence spectrophotometer (RF-6000, Shimadzu, Tokyo, Japan) was used to determine the fluorescence characteristics of the algae-laden water and UF permeate. Three-dimensional excitation–emission matrix (EEM) fluorescence spectra were obtained at excitation wavelengths of 220–450 nm by 5 nm increments and emission wavelengths of 250–550 nm by 1 nm increments.37 The photomultiplier tube (PMT) voltage was set at 700 V and a scanning speed of 2400 nm min−1 was adopted. A Raman normalized Milli-Q EEM was subtracted from the data to remove most Raman scatter peaks.38 The pH values of the samples were adjusted to 7.0 ± 0.1 prior to fluorescence analyses.
The morphological characteristics of fouling layers on the membranes were characterized by scanning electron microscopy (SEM, FEI Quanta 200FEG, Eindhoven, Netherlands). Three types of samples were collected including fouled membranes, physically cleaned membranes and chemically cleaned membranes. The physical cleaning was performed by sponge scrubbing until no visible foulants were observed on the membrane.39 The sponge scrubbing could remove the external fouling layer on the membrane. The chemical cleaning was comprised of successively soaking the samples in a NaOH solution (0.1 mol L−1) for 30 min and a HCl solution (1%) for 60 min. The samples were cut into small rectangle pieces (2.0 cm × 1.0 cm) and pasted on an aluminum sheet. Then, the samples were naturally dried and coated with gold prior to SEM analysis. Moreover, energy-dispersive X-Ray spectroscopy (EDX) was used to assess the deposition of Fe and Mn on the membrane.
3. Results and discussion
3.1 Effect of Fe(II)/permanganate on the characteristics of algae-laden water
Fig. 2 shows variations in zeta potentials and particle sizes of the algae-laden water when permanganate and Fe(II) were added at increasing doses. As shown in Fig. 2(a), the permanganate oxidation did not cause significant changes in zeta potentials, regardless of doses. For Fe(II) coagulation alone, the zeta potential increased with increasing Fe(II) doses, but the increase was not significant. In contrast, the zeta potentials substantially increased in the presence of Fe(II)/permanganate with a zeta potential of −14.2 mV at a dose of 50/150 μmol. This is attributed to the presence of in situ formed Fe(III), which may adsorb on algal cells and neutralize the negative charge on the surface.40 The particle size of algal cells mainly distributed in the range of 2–10 μm and the permanganate oxidation caused no change to the size distribution of algal cells (Fig. 2(b)). The presence of Fe(II) did not induce substantial aggregation of algal cells either, with the peak value (2–10 μm) only slightly decreased (Fig. 2(c)) at doses higher than 30 μmol. This is attributed to the high concentration of algal cells used and the presence of EOM which has a very high coagulant demand.41 However, the combination of Fe(II) and permanganate caused significant flocculation of algal cells and more algae agglomerates were observed in the range of 10–100 μm (Fig. 2(d)), particularly at higher doses. The results indicate that the presence of in situ formed manganese oxides and Fe(III) greatly accelerated the coagulation of algae laden water. On the one hand, in situ formed manganese oxides may adsorb on the algal cells and induce changes in interfacial characteristics. In previous work, in situ formed manganese oxide particles were shown to promote the aggregation of the cells and improve their settleability.24 On the other hand, in situ formed Fe(III), which is trivalent and has strong activity, can readily react with particles and induce formation of flocs.27 In another study on coagulation of natural organics, the in situ formed Fe(III) was demonstrated to produce larger flocs than the pre-dosed Fe(III).42
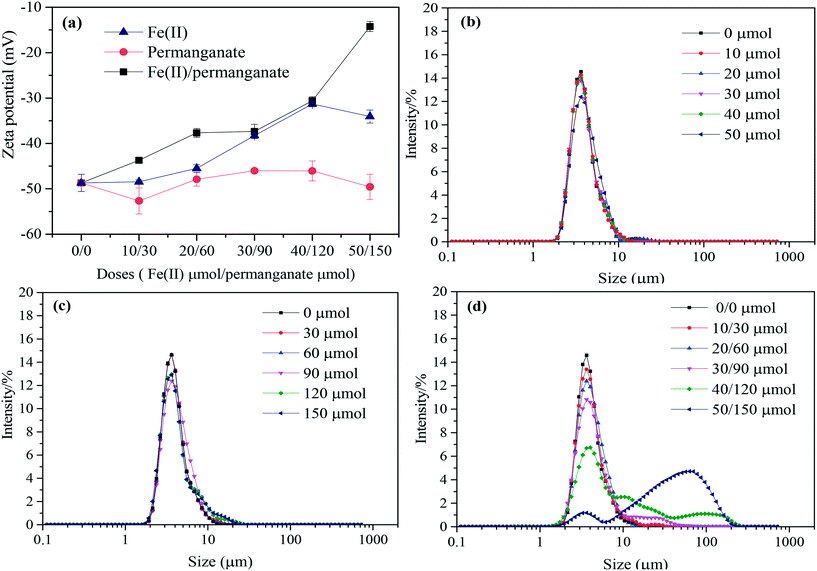 |
| Fig. 2 Zeta potentials (a) and size distributions (b–d) of algal cells in the presence of permanganate and Fe(II): permanganate alone (b), Fe(II) alone (c) and Fe(II)/permanganate (d). The reaction time was 10 min. The dose range of Fe(II) and permanganate was 0–50 μmol and 0–150 μmol, respectively. The X-axis in (a) denotes the corresponding doses of the chemical used alone. | |
3.2 Effect of Fe(II)/permanganate on UF membrane fouling by algae
Fig. 3 shows the specific flux and fouling resistances during algae-laden water filtration. As shown in Fig. 3(a), the UF membrane was severely fouled during filtration of the untreated algae-laden water with the specific flux decreasing by 60% in the initial 100 mL. The flux decline gradually slowed down in the later filtration phase until a plateau occurred with a specific flux of 0.14 at the end of filtration. The pretreatments with Fe(II) or permanganate were able to slightly alleviate the fouling caused by algae. In contrast, the Fe(II) coagulation was slightly more efficient in alleviating flux reduction than the permanganate oxidation. When the combined Fe(II)/permanganate was used to pre-treat the algae-laden water, the membrane fouling was substantially alleviated with the specific flux slowly and gradually decreasing over the whole filtration. The flux reduction was less than 22% at the end of filtration. As shown in Fig. 3(b), the reversible and irreversible resistances after filtration of the untreated algae-laden water were 9.1 × 1011 m−1 and 7.2 × 109 m−1, respectively, implying that fouling by algae was dominated by reversible fouling. The reversible resistance decreased to 7.6 × 1011 m−1 when Fe(II) or permanganate was used to pretreat the algae-laden water. With the combined Fe(II)/permanganate, the reversible resistance was only 1.0 × 1010 m−1, nearly 2 orders of magnitude lower than that in filtration of the untreated algae-laden water. The results indicate that Fe(II)/permanganate is most efficient in alleviating flux decline and reversible fouling caused by algae. There are two interpretations for reduced reversible fouling in the presence of Fe(II)/permanganate. On the one hand, Fe(II) is able to accelerate reduction of permanganate and generation of manganese dioxide which can adsorb soluble organics, resulting in less organic deposition on the membrane surface and inside membrane pores. On the other hand, in situ formed Fe(III), which proves to have higher floc activity than dosed Fe(III), is capable of coagulating algal cells and organics, resulting in large flocs and a more porous cake layer as the flocs arrive at the membrane surface. Moreover, manganese dioxide may serve as a condensation nucleus,43 further promoting the formation of larger flocs. Ma et al.29 have performed a long-time investigation into fouling control during natural reservoir water treatment by UF, and lower TMP increases were observed with Fe(II)/permanganate over the whole duration (90 d) than with Fe(III) coagulation.
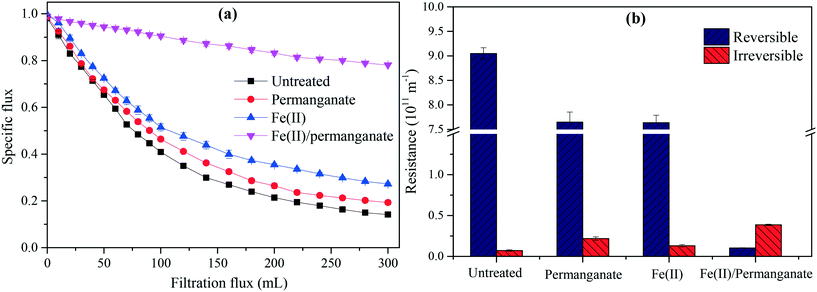 |
| Fig. 3 Flux decline curves (a) and fouling resistances (b) during algae-laden water treatment using UF with different pretreatments. The doses of permanganate and Fe(II) were 50 μmol and 150 μmol, respectively. The reaction time was 10 min. | |
However, as shown in Fig. 3(b), the irreversible resistances were not reduced but increased when the pretreatments were applied. The pretreatments with permanganate only and Fe(II) only increased the irreversible resistances to 2.1 × 1010 m−1 and 1.3 × 1010 m−1, respectively. In particular, the irreversible resistance increased to 3.8 × 1010 m−1, when Fe(II)/permanganate was used. The results indicate that permanganate oxidation and Fe(II) coagulation, regardless of being applied individually or together, caused adverse impacts on the fouling reversibility in the short-term filtration of the algae-laden water. As previous studies showed that permanganate oxidation was able destroy algal cells at doses exceeding 20 μmol, the adverse impacts on fouling reversibility associated with permanganate (50 μmol) can be attributed to the release of intracellular organic matter (IOM), which proves to cause irreversible fouling.5 Regarding Fe(II) coagulation, the reduced electrostatic repulsion between the organics and membrane surface may play a role in aggravating the irreversible fouling. In the case of Fe(II)/permanganate, cake filtration is weakened due to agglomeration of foulants in the presence of in situ formed manganese dioxide and Fe(III), resulting in more organics deposited on the membrane surface. In previous work, irreversible fouling was also aggravated during algae-laden water filtration when Fe(II)/persulfate was applied, even though strong oxidation by sulfate radicals was involved.19 Overall, Fe(II)/permanganate may adversely affect the hydraulic reversibility of membrane fouling in algae-laden water treatment with UF.
Fig. 4 shows the results from fitting the flux data to the combined blocking and cake filtration model. The filtration data were replotted as d2t/dV2versus dt/dV with the exponent n indicating the dominant mechanism. Pictures of the fouled membranes after filtration were also provided. For the untreated algae-laden water, the exponent n was 1.274 in the filtration volume range of 0–100 mL, indicating that the standard blocking and intermediate blocking dominated the initial fouling process. As the filtration volume exceeded 100 mL, the exponent n was changed to 0.021, implying that the fouling layer was formed and that the principal fouling mechanism shifted to cake filtration. The dual fouling mechanism has been reported in our previous work for reservoir water treatment using a pilot scale UF system.44 When the filtration was finished, a thick and bright-green cake layer was observed on the membrane surface. Meanwhile, it can be observed that the algal cells escaped from the cake layer to some extent, leaving lots of voids and exposed areas on the membrane surface. In the filtration process, the TMP imposes a high permeate drag on the foulants;45 as the permeate drag overcomes the electrostatic repulsion and hydration force, the algal cells were stacked in the fouling layer. When the permeate drag was retarded, the compressed fouling layer became loose due to the electrostatic repulsion and hydration force, resulting in the aforementioned escaped cells. As shown in Fig. 4(b) and (c), the membrane fouling was still governed by the dual fouling mechanism with pore blocking and cake filtration successively dominating the fouling process, although permanganate oxidation and Fe(II) coagulation were used. However, the cake layers were dense and did not deform when the TMP was not imposed after filtration. In contrast, the cake layer was still bright-green in the case of Fe(II) coagulation, whereas the cake layer became dark-green in the presence of permanganate, because manganese dioxides deposited on the inactivated algal cells. In our previous work, deposition of manganese dioxides proved to decrease the zeta potentials of algal cells. Fe(II) is also a typical divalent ion which is able to facilitate agglomeration of algal cells.19 Hence, the cake layer did not deform after filtration in the presence of permanganate or Fe(II), attributed to reduced electrostatic repulsions among the cells. This is consistent with the increased hydraulically irreversible fouling (Fig. 3(b)) in the presence of permanganate or Fe(II). However, when the combined Fe(II)/permanganate was used to pretreat the algae-laden water, the fouling process was completely changed with the exponent n of 1.486 over the whole filtration duration, indicating that the standard blocking dominated the entire fouling process. The brown agglomerates, which were comprised of inactivated cells and iron and manganese oxides, loosely distributed on the membrane surface after filtration. The void areas on the membrane surface also looked yellowish-brown, implying direct deposition of iron and manganese oxides on the membrane. In our previous work, standard blocking was also identified as the principal mechanism for fouling during filtration of algae-laden when Fe(II)/persulfate was used as a pretreatment.42 As is known, irreversible fouling during algae-laden water filtration is mainly attributed to deposition of algal organics on or inside membrane pores.46In situ formed Fe(III) can reduce the electrostatic repulsion between the membrane and the foulants, and the adhesion of small EOM inside the membrane pores is enhanced and contributes to the increased role of the standard blocking mechanism. Moreover, the fouling layer formed by the agglomerates was much more porous than that by untreated algal cells, resulting in a less significant role of cake filtration. Hence, the hydraulically irreversible fouling was not reduced but aggravated in this study when Fe(II)/permanganate was applied to treat algae-laden water.
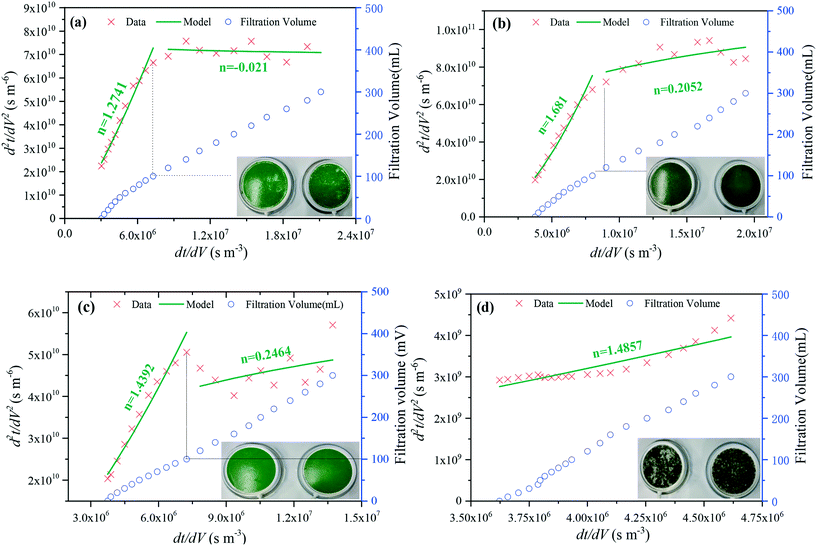 |
| Fig. 4 d2t/dV2versus dt/dV curves for the filtration of algae-laden water pretreated by different pretreatments: (a) UF, (b) permanganate/UF, (c) Fe(II)/UF and (d) Fe(II)/permanganate/UF. The doses of permanganate and Fe(II) were 50 μmol and 150 μmol, respectively. The reaction time for the pretreatment was 10 min. | |
3.3 Effect of Fe(II)/permanganate doses on performance in UF membrane fouling control
Fig. 5 shows flux profiles and resistances during filtration of the algae-laden water which was pretreated by Fe(II)/permanganate at increasing doses. The impacts of permanganate alone and Fe(II) alone are shown in Fig. S3 and S4 in the ESI.† Generally, the flux decline during filtration of the algae-laden water was decreased with increasing doses, regardless of being used alone or in a combined manner. When the Fe(II)/permanganate dose increased from 10/30 μmol to 30/90 μmol, the specific flux at the end of filtration increased from 0.21 to 0.30. As Fe(II)/permanganate was used at higher doses of 40/120 μmol and 50/150 μmol, the specific flux at the end of filtration substantially increased to 0.56 and 0.78, respectively. The most insignificant increase occurred at the Fe(II)/permanganate dose of 40/120 μmol, which is well consistent with the threshold dose from the zeta potential tests. In contrast, the improvements in the membrane permeability were less significant with permanganate alone and Fe(II) alone. For Fe(II) alone, the flux decline over the whole filtration was still as high as 73% at the highest Fe(II) dose of 150 μmol. As shown in Fig. 5(b), the reversible fouling after filtration of the pretreated algae-laden water also substantially decreased with increasing Fe(II)/permanganate doses. The reversible resistance at the highest Fe(II)/permanganate dose was 1.0 × 1010 m−1, almost 2 orders of magnitude lower than that caused by untreated algae-laden water. In another similar study on algae fouling control by Fe(II)/permanganate, the flux reduction generally decreased with increasing Fe(II)/permanganate doses and the optimum dose was identified as 6/18 μmol, which was much less than the doses used in this work.29 This is attributed to different algae-laden water used. The former study used natural reservoir water with a low algae concentration (1.99 × 107 to 2.67 × 107 cells per L), whereas the lab-prepared algae-laden water in this study had an algae concentration as high as 2.0 × 106 cells per mL. The irreversible resistances with Fe(II)/permanganate were generally higher than that caused by the untreated algae-laden water, regardless of the doses. However, the irreversible resistances did not significantly vary with increasing Fe(II)/permanganate doses (P > 0.05). The lowest irreversible fouling occurred at the Fe(II)/permanganate dose of 30/90 μmol, whereas the most severe irreversible resistance was observed at the highest Fe(II)/permanganate dose (50/150 μmol). As aforementioned, Fe(II)/permanganate may generate in situ formed manganese oxides and Fe(III), resulting in the reduced role of cake filtration and enhanced adhesion of EOM onto the membrane. However, the cake filtration depends on not only porosity but also interaction between the foulants. With increasing Fe(II)/permanganate doses, the porosity of the fouling layer increased, resulting in less cake interception, but the interaction between the agglomerates and EOM, which contributed to cake interception, was enhanced in the presence of in situ formed Fe(III). Hence, multiple mechanisms are involved in the formation of irreversible fouling during algae-laden water treatment with Fe(II)/permanganate used as the pretreatment. The doses of Fe(II)/permanganate should be optimized with both membrane permeability and fouling reversibility taken into consideration.
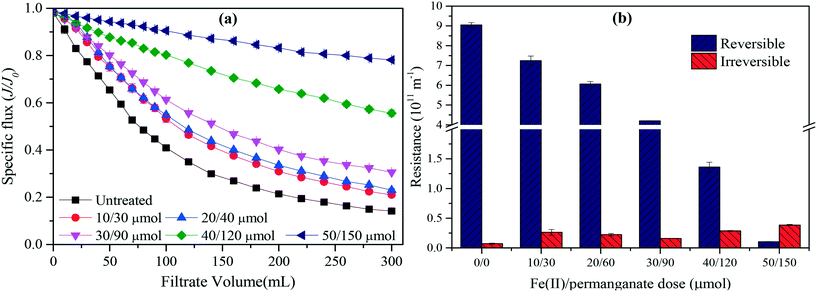 |
| Fig. 5 Flux curve profile (a) and membrane resistance (b) during filtration of algae-laden water with Fe(II)/permanganate pretreatments at increasing doses. The reaction time for the pretreatment was 10 min. | |
3.4 Morphological characteristics of fouled membranes and deposition of Fe and Mn
Fig. 6 shows SEM images of fouled membranes before and after cleaning. Thick cake layers are observed on the fouled UF membrane regardless of the pretreatments. The algal cells compactly stacked on the membrane for the untreated algae-laden water, forming a thick cake layer. Neither permanganate oxidation nor Fe(II) coagulation brought apparent changes to the morphological characteristics of the cake layers. In contrast, the agglomerates of algal cells and iron/manganese oxides loosely stacked with lots of void spaces, when Fe(II)/permanganate was used to pretreat the algae-laden water. This result conforms with the improved membrane permeability during filtration after the Fe(II)/permanganate pretreatment. When the fouled membrane was physically cleaned by sponge scrubbing, the external fouling layers were completely removed. Only a few foulants could be observed on the membrane in the case of Fe(II)/permanganate. After the chemical cleaning, no foulants could be found on the membrane surface. As the UF membrane was covered by a brown layer after the Fe(II)/permanganate pretreatment, the EDX analysis of fouled membranes was performed to investigate whether there were iron/manganese oxides deposited on or inside the organic matrix of the membrane. The EDX spectra are shown in Fig. S4 in the ESI,† and the ratios of Mn and Fe in terms of weight are summarized in Table 1. The elements Mn and Fe were detected in neither fouled nor cleaned membranes after filtering the untreated algae-laden water. When permanganate was added into the algae-laden water, Mn accounted for 1.08% of the total elements detected. In the case of Fe(II), the weight ratio of Fe was 2.89%. In contrast, both Mn and Fe were detected in the fouled membrane in terms of Fe(II)/permanganate, and the weight ratios of Mn and Fe increased to 16.54% and 4.83%, respectively. However, when the external fouling layers were removed by sponge scrubbing, neither Mn nor Fe was detected. The results confirm that the combination of Fe(II) and permanganate could enhanced the generation of iron and manganese oxides. Moreover, both iron and manganese oxides only deposited in the external fouling layer. Hence, application of Fe(II)/permanganate would not induce the risk of augmentation in membrane weight due to deposition of the iron and manganese oxides inside the membrane.
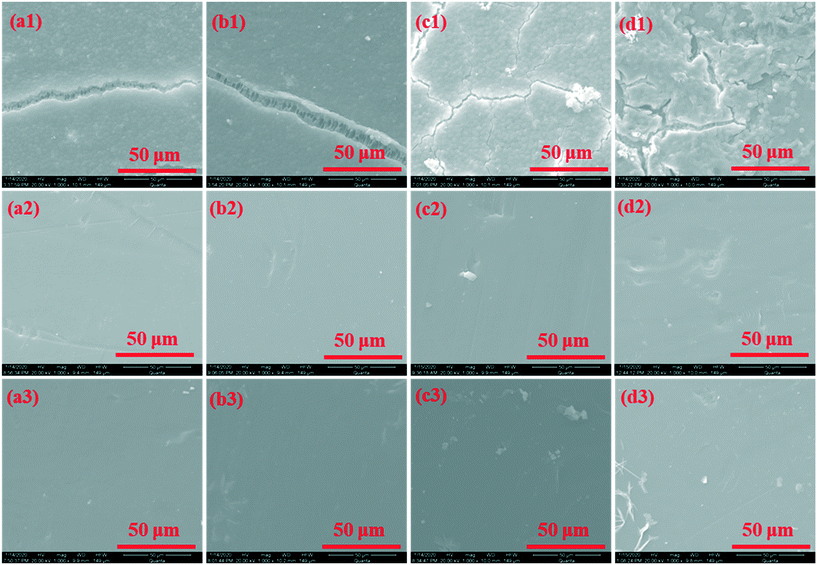 |
| Fig. 6 SEM images of the membranes fouled by the algae-laden water: (a) untreated, (b) permanganate only, (c) Fe(II) only and (d) Fe(II)/permanganate. 1, 2 and 3 represent the fouled membrane, the physically cleaned membrane and the chemically cleaned membrane. The doses of permanganate and Fe(II) were 50 μmol and 150 μmol, respectively. | |
Table 1 EDX analysis of the top surface of fouled membranes in treatment of the algae-laden water. The doses of permanganate and Fe(II) were 50 μmol and 150 μmol, respectively
Pretreatment |
Samples |
Element (weight, %) |
Fe |
Mn |
None |
Fouled |
ND |
ND |
Physically cleaned |
ND |
ND |
Chemically cleaned |
ND |
ND |
Permanganate |
Fouled |
ND |
1.08 |
Physically cleaned |
ND |
ND |
Chemically cleaned |
ND |
ND |
Fe(II) |
Fouled |
2.89 |
ND |
Physically cleaned |
ND |
ND |
Chemically cleaned |
ND |
ND |
Fe(II)/permanganate |
Fouled |
16.54 |
4.83 |
Physically cleaned |
ND |
ND |
Chemically cleaned |
ND |
ND |
3.5 Effect of Fe(II)/permanganate on retention of algal organics
Fig. 7 shows the removal rates of DOC and UV254 during algae-laden water filtration with and without pretreatments. As shown in Fig. 7(a), when the algae-laden water was treated by UF without pretreatments, the DOC and UV254 removal rates were 57% and 37%, respectively. This is consistent with a large fraction of macromolecular organics in EOM. However, the DOC removal rate decreased to 42% in the presence of permanganate, which was attributed to algal cell breakage and release of intracellular organics. Besides, the presence of permanganate disturbed the measurement of UV254 and thus the UV254 removal for permanganate only was not presented. In the case of Fe(II), a lower DOC removal rate (46%) was observed whereas the UV254 removal (60%) was considerably improved. When Fe(II)/permanganate was used as the pretreatment, the organic removal performance significantly increased, with the DOC and UV254 removal rates of 67% and 81%, respectively. The results indicate that the combination of permanganate and Fe(II) could offset the negative impacts related to oxidant exposure and structural changes in the cake layer. On the one hand, permanganate preferentially reacts with Fe(II), and thus the inactivation of algae ceased, resulting in limited release of IOM. On the other hand, Fe(II) could be oxidized into in situ formed Fe(III), which is more efficient than pre-formed Fe(III) with regard to removal of EOM, especially those with unsaturated bonds.26Fig. 7(b) shows variations in organic removal performance with increasing permanganate and Fe(II) doses. It can be observed that the UV254 removal significantly increased with increasing Fe(II)/permanganate doses, attributed to the adsorption of in situ formed manganese dioxide and Fe(III).The DOC rejection by UF did not regularly vary with increasing Fe(II)/permanganate doses. The increase in DOC rejection was only observed at Fe(II)/permanganate doses within 40/120 μmol. However, the DOC rejection decreased to 67% at Fe(II)/permanganate doses of 50/150 μmol. The DOC removal depends on membrane adsorption, size exclusion and cake interception when the fouling layer is formed, and release of IOM due to oxidant exposure may bring adverse impacts. As the permanganate was used together with Fe(II), algal cell breakage and release of IOM can be excluded from the reasons for decreased DOC removal at the highest Fe(II)/permanganate dose.26In situ formed Fe(III) may enhanced foulant-membrane interactions via reducing electrostatic repulsion, resulting in enhanced membrane adsorption. However, as shown in Fig. 2(d), the algal cells tended to agglomerate when Fe(II)/permanganate was used as the pretreatment. The agglomerates, which were formed by algal cells and iron/manganese oxides, loosely stacked on the membrane (Fig. 6(d1)), resulting in increased porosity and weakened cake interception of the fouling layer. This is verified by the invalid role of cake filtration in the fouling mechanism with Fe(II)/permanganate (Fig. 4(d)). Hence, the organic rejection during filtration of the algae-laden water can be compromised by weakened cake interception in the presence of iron and manganese oxides, especially at high Fe(II)/permanganate doses.
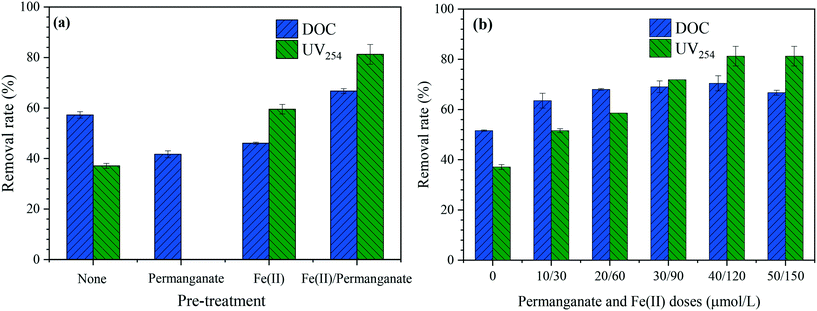 |
| Fig. 7 Removal performance for DOC and UV254 in pre-treatment units (a) and in pre-treatment coupled with UF processes (b). | |
Fig. 8 shows three-dimensional EEM spectra of the algae-laden water and UF permeate. An EEM spectrum can be divided into five regions: regions I (Ex/Em: 220–250 nm/280–330 nm) and II (Ex/Em: 220–250 nm/330–380 nm) are associated with aromatic proteins (such as tyrosine and tryptophan); region III (Ex/Em: 220–250 nm/380–550 nm) is attributed to fulvic acid-like materials; region IV (Ex/Em: 250–450 nm/280–380 nm) and region V (Ex/Em: 250–450 nm/380–550 nm) are related to soluble microbial products (SMPs) and humic acid-like materials, respectively.47 Because algal cells can secrete EOM which is comprised of proteins, there are strong fluorescent peaks in regions II and IV (Fig. 8(a)). In our previous work, the humic acid-like substances, which originated from the decomposition of dead cells and macromolecular organics by epiphytic heterotrophic bacteria, were less than 10 kDa in molecular weight.4 When the algae-laden water was treated with the UF membrane, the fluorescence peaks in regions II and IV substantially decreased in intensity due to the high molecular weight of proteins. However, the fluorescence peaks in regions II and IV were not eradicated, because some small amino acids, peptides and enzymes, which also have fluorescence characteristics, can penetrate across the membrane.44 As shown in Fig. 8(c) and (d), when permanganate or Fe(II) was used to pretreat the algae-laden water, the fluorescence intensities in the five regions all substantially decreased. In particular, the protein-like substances were almost totally removed. Although permanganate oxidation can destroy some algal cells, resulting in release of IOM, the removal of fluorescent components was not weakened. This is attributed to cake filtration by the thick and compacted fouling layer (Fig. 4(b)). Moreover, the intermediate product, hydrous manganese dioxide, may also contribute to adsorption of organics to some extent. In the case of Fe(II), the improved retention of fluorescent components is associated with the reduced electrostatic repulsion which enhances interception of organics in cake layers. For Fe(II)/permanganate, the rejection of fluorescent organics during filtration was also improved (Fig. 8(e)), attributed to occurrence of in situ formed manganese dioxide and Fe(III). In our previous work on algae-laden water treatment with Fe(II)/persulfate, in situ formed Fe(III) substantially improved removal of not only EOM (60–69%) but also small odor compounds.19
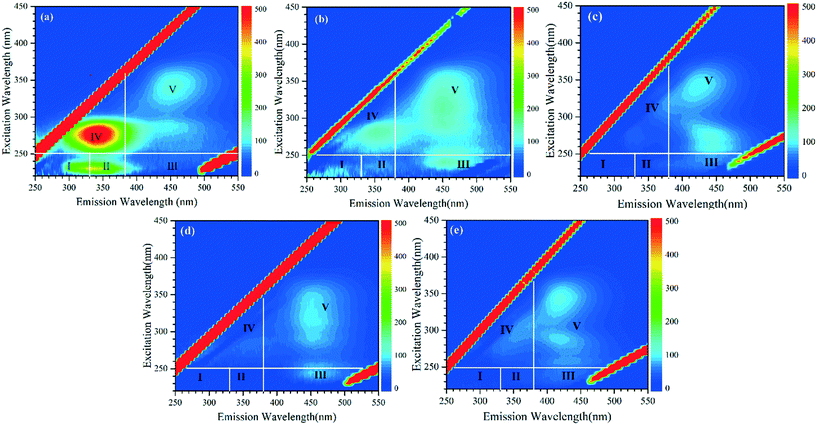 |
| Fig. 8 Fluorescence EEM spectra of algae-laden water: (a) no treatment, (b) UF, (c) permanganate/UF, (d) Fe(II)/UF and (e) Fe(II)/permanganate/UF. The doses of permanganate and Fe(II) were 50 μmol and 150 μmol, respectively. The contact time was 10 min. | |
4. Conclusions
In this study, Fe(II)/permanganate was used as a pretreatment of algae-laden water before UF in comparison with permanganate alone and Fe(II) alone. The following conclusions can be drawn:
(1) Fe(II)/permanganate was able to enhance agglomeration of algal cells and removal of algal organics (67%) by UF, attributed to generation of in situ formed manganese oxides and Fe(III). However, permanganate alone and Fe(II) alone adversely affected organic removal due to cell breakage and weakened cake filtration, respectively.
(2) Compared to Fe(II) or permanganate, the combined Fe(II)/permanganate substantially decreased the flux reduction by 65% and reduced the reversible resistance by nearly 2 orders of magnitude during filtration of algae-laden water, and the performance was improved with increasing doses. The fouling mechanism shifted from the dual mechanism of successive pore blocking and cake filtration to the single standard blocking over the whole duration investigated.
(3) The irreversible fouling during algae-laden water filtration was aggravated by Fe(II)/permanganate. The cake filtration was weakened in the presence of iron and manganese oxides and in situ formed Fe(III) aggravated adhesion of EOM on the membrane.
(4) The individual use of permanganate and Fe(II) resulted in deposition of Fe and Mn, respectively, on the membrane surface. The amounts of Fe and Mn substantially increased by more than 4 times when Fe(II)/permanganate was used. These iron and manganese oxides deposited in the external fouling layer rather than inside the membrane.
Conflicts of interest
There are no conflicts to declare.
Acknowledgements
This work was financially supported by the Natural Science Foundation of China (Grants 51878211, 51778155), the Natural Science Foundation of Guangdong Province (2019A1515012232) and the Open Project of State Key Laboratory of Urban Water Resource and Environment (QA201816).
References
- J. G. Jacangelo, R. R. Trussell and M. Watson, Desalination, 1997, 113, 119–127 CrossRef CAS.
- H. Huang, T. A. Young, K. J. Schwab and J. G. Jacangelo, J. Membr. Sci., 2012, 409–410, 1–8 CrossRef CAS.
- C. W. K. Chow, S. Panglisch, J. House, M. Drikas, M. D. Burch and R. Gimbel, Aqua, 1997, 46, 324–334 CAS.
- F. Qu, H. Liang, Z. Wang, H. Wang, H. Yu and G. Li, Water Res., 2012, 46, 1490–1500 CrossRef CAS.
- L. Li, Z. Wang, L. C. Rietveld, N. Gao, J. Hu, D. Yin and S. Yu, Environ. Sci. Technol., 2014, 48, 14549–14557 CrossRef CAS.
- X. Zhang, L. Fan and F. A. Roddick, J. Membr. Sci., 2013, 425–426, 23–29 CrossRef CAS.
- S. Babel and S. Takizawa, Desalination, 2011, 274, 171–176 CrossRef CAS.
- W. Huang, M. Hu, X. Qin, W. Zhou, W. Lv and B. Dong, Algal Res., 2017, 25, 252–262 CrossRef.
- B. Liu, F. Qu, H. Liang, B. Van der Bruggen, X. Cheng, H. Yu, G. Xu and G. Li, Water Res., 2017, 112, 83–92 CrossRef CAS.
- R. K. Henderson, N. Subhi, A. Antony, S. J. Khan, K. R. Murphy, G. L. Leslie, V. Chen, R. M. Stuetz and P. Le-Clech, J. Membr. Sci., 2011, 382, 50–59 CrossRef CAS.
- Z. Yan, F. Qu, H. Liang, H. Yu, H. Pang, H. Rong, G. Fan and B. Van der Bruggen, J. Membr. Sci., 2021, 617, 118638 CrossRef CAS.
- B. J. Deka, J. Guo, S. Jeong, M. Kumar and A. K. An, Environ. Sci.: Water Res. Technol., 2020, 6, 935–944 RSC.
- D. Wei, Y. Tao, Z. Zhang, L. Liu and X. Zhang, J. Membr. Sci., 2016, 498, 116–124 CrossRef CAS.
- D. Wei, Y. Tao, Z. Zhang and X. Zhang, Chem. Eng. J., 2016, 294, 157–166 CrossRef CAS.
- X. Zhang, L. Fan and F. A. Roddick, J. Membr. Sci., 2015, 493, 683–689 CrossRef CAS.
- J. Xing, H. Liang, S. Xu, C. J. Chuah, X. Luo, T. Wang, J. Wang, G. Li and S. A. Snyder, Water Res., 2019, 159, 283–293 CrossRef CAS.
- X. Cheng, D. Wu, H. Liang, X. Zhu, X. Tang, Z. Gan, J. Xing, X. Luo and G. Li, Water Res., 2018, 145, 39–49 CrossRef CAS.
- Y. Wan, P. Xie, Z. Wang, J. Ding, J. Wang, S. Wang and M. R. Wiesner, Water Res., 2019, 158, 213–226 CrossRef CAS.
- B. Liu, F. Qu, H. Yu, J. Tian, W. Chen, H. Liang, G. Li and B. Van der Bruggen, Environ. Sci. Technol., 2018, 52, 765–774 CrossRef CAS.
- G. Fan, X. Zheng, J. Luo, H. Peng, H. Lin, M. Bao, L. Hong and J. Zhou, Chem. Eng. J., 2018, 351, 782–790 CrossRef CAS.
- L. A. Coral, A. Zamyadi, B. Barbeau, F. J. Bassetti, F. R. Lapolli and M. Prevost, Water Res., 2013, 47, 2983–2994 CrossRef CAS.
- J. Sun, L. Bu, L. Deng, Z. Shi and S. Zhou, Chem. Eng. J., 2018, 349, 408–415 CrossRef CAS.
- J. Zhou, J. Liu, Z. Zhao, W. Peng, F. Cui and Z. Liang, Chem. Eng. J., 2020, 382 Search PubMed.
- F. Qu, X. Du, B. Liu, J. He, N. Ren, G. Li and H. Liang, Chem. Eng. J., 2015, 279, 56–65 CrossRef CAS.
- J. J. Chen, H. H. Yeh and I. C. Tseng, Chemosphere, 2009, 74, 840–846 CrossRef CAS.
- M. Ma, R. Liu, H. Liu and J. Qu, Water Res., 2014, 65, 73–84 CrossRef CAS.
- J. Qi, H. Lan, H. Liu, R. Liu, S. Miao and J. Qu, Water Res., 2016, 105, 551–558 CrossRef CAS.
- W. Yu and N. J. D. Graham, J. Membr. Sci., 2015, 473, 283–291 CrossRef CAS.
- B. Ma, J. Qi, X. Wang, M. Ma, S. Miao, W. Li, R. Liu, H. Liu and J. Qu, Water Res., 2018, 142, 96–104 CrossRef CAS.
- X. Du, G. Liu, F. Qu, K. Li, S. Shao, G. Li and H. Liang, Desalination, 2017, 403, 97–106 CrossRef CAS.
- M. Pivokonsky, J. Naceradska, I. Kopecka, M. Baresova, B. Jefferson, X. Li and R. K. Henderson, Crit. Rev. Environ. Sci. Technol., 2015, 46, 291–335 CrossRef.
- B. Liu, T. Zhu, W. Liu, R. Zhou, S. Zhou, R. Wu, L. Deng, J. Wang and B. Van der Bruggen, Water Res., 2020, 187, 116435 CrossRef CAS.
- C. C. Ho and A. L. Zydney, J. Colloid Interface Sci., 2000, 232, 389–399 CrossRef CAS.
- J. Hermia, Trans. Inst. Chem. Eng., 1982, 60, 183–187 CAS.
- F. Qu, H. Wang, J. He, G. Fan, Z. Pan, J. Tian, H. Rong, G. Li and H. Yu, Environ. Sci.: Water Res. Technol., 2019, 5, 672–683 RSC.
- C.-C. Ho and A. L. Zydney, J. Colloid Interface Sci., 2000, 232, 389–399 CrossRef CAS.
- H. Yu, F. Qu, Z. Wu, J. He, H. Rong and H. Liang, Water Res., 2020, 187, 116452 CrossRef CAS.
- H. Yu, F. Qu, X. Zhang, S. Shao, H. Rong, H. Liang, L. Bai and J. Ma, Chemosphere, 2019, 228, 35–43 CrossRef CAS.
- S. Shao, Y. Wang, D. Shi, X. Zhang, C. Y. Tang, Z. Liu and J. Li, Sci. Total Environ., 2018, 644, 306–314 CrossRef CAS.
- M. Ma, R. Liu, H. Liu and J. Qu, J. Hazard. Mater., 2012, 217–218, 279–285 CrossRef CAS.
- M. Pivokonsky, J. Naceradska, T. Brabenec, K. Novotna, M. Baresova and V. Janda, Water Res., 2015, 84, 278–285 CrossRef CAS.
- W.-z. Yu, N. Graham, H.-j. Liu, H. Li and J.-h. Qu, J. Membr. Sci., 2013, 431, 47–54 CrossRef CAS.
- J. Qi, H. Lan, S. Miao, Q. Xu, R. Liu, H. Liu and J. Qu, Water Res., 2016, 88, 127–134 CrossRef CAS.
- F. Qu, Z. Yan, H. Wang, X. Wang, H. Liang, H. Yu, J. He and G. Li, Environ. Sci.: Water Res. Technol., 2018, 4, 315–324 RSC.
- W. Huang, H. Chu and B. Dong, Colloids Surf., B, 2014, 122, 447–456 CrossRef CAS.
- F. Qu, H. Liang, J. Tian, H. Yu, Z. Chen and G. Li, Desalination, 2012, 293, 30–37 CrossRef CAS.
- W. Chen, P. Westerhoff, J. A. Leen and K. Booksh, Environ. Sci. Technol., 2003, 37, 5701–5710 CrossRef CAS.
Footnote |
† Electronic supplementary information (ESI) available. See DOI: 10.1039/d0ew00833h |
|
This journal is © The Royal Society of Chemistry 2021 |