Nitrite denitrification using biomass acclimatized with methanol as complementary carbon source: long-term performance and kinetics study†
Received
25th June 2020
, Accepted 5th October 2020
First published on 26th October 2020
Abstract
The objective of this study was to investigate the dynamic specific denitrification rates (SDNRs) from nitrite at various COD/N ratios in a system that used methanol as a complementary carbon source. An SBR fed with municipal wastewater (MWW), methanol and nitrite solution was operated at an HRT of 8.4 h and SRT of 16–17 days for 110 days. The SDNR from the SBR employing methanol as a complementary carbon source was compared with those determined in 23 batch reactors employing MWW, methanol, nitrite, and nitrate. The batch tests were conducted using the biomass acclimatized with nitrite and methanol as a complementary carbon source. The maximum SDNR was 0.52 mgN per mgVSS per d, corresponding to COD/N ratios of 5.9 in the SBR, which was about eight times higher than the literature reported value for systems fed with MWW. However, the maximum nitrite SDNR was lower than the observed batch nitrate 1.06 mgNO3−-N per mgVSS per d SDNR using the nitrite acclimatized biomass. In the batch test, a lower nitrite SDNR (0.38 mgNO2−-N per mgVSS per d) was achieved using methanol as a sole carbon source. The nitrite denitrifier yields were 0.39 and 0.34 mgVSS per mgCOD using methanol as a complementary and sole carbon source, respectively. The half-saturation coefficient of nitrite (KNO2) during post-denitrification with methanol was found to be 9.2 mg L−1, which confirmed the lower SDNR values at lower nitrite concentrations below the KNO2. The higher SDNR rates in methanol usage as a complementary carbon source versus sole carbon source could be translated into 37% capital and operating cost savings.
Water impact
Carbon, nitrogen, and phosphorus are major environmental stressors that pollute lakes, drinking water wells, and streams. They originate from human activities and come through municipal and home sewage systems. Wastewater treatment plants (WWTPs) must remove these stressors before discharge into water bodies. Typically biological wastewater treatment process is used to treat domestic wastewater using microorganisms to remove organic matter and nutrients. It requires added oxygen and therefore uses electrical energy from fossil fuels to drive conversion. Although robust, this process is often energy-intensive, with a large carbon footprint, therefore this paper provides fundamental to enhance our understanding in biological wastewater treatment. Ultimately, our effort is to protect the source water, and that is how the paper will impact society.
|
1. Introduction
Conventional nitrogen removal from domestic wastewater is a process that involves nitrification of ammonia and soluble biodegradable organic nitrogen to nitrate and nitrite. Then, these oxidized forms of nitrogen can be reduced to nitrogen gas by facultative heterotrophs when a carbon source is available. Most of the nitrogen removal treatment technologies in wastewater treatment plants employ nitrification and denitrification processes. This process is accompanied by the removal of organic carbon, which acts as an electron donor. As denitrification occurs under anoxic conditions, the process is affected by various parameters such as dissolved oxygen (DO), type and availability of organic carbon, COD-to-nitrogen ratio, temperature, and pH.1–5
Further, new processes are being developed for biological nitrogen removals (BNR) such as anaerobic ammonium oxidation (ANAMMOX) and partial nitrification–denitrification.6–8 Notably, ANAMMOX technology, which removes ammonia nitrogen with nitrite under anaerobic condition, received substantial attention as it significantly reduces aeration cost and external carbon use. In order to achieve a successful ANAMMOX process, partial nitrification technology, by converting part of the ammonia to nitrite, was also extensively studied.9–13 However, conventional biological nutrient removal (BNR) technologies are still widely used due to system stability and the scale-up issue of emerging technologies.14–19 One viable option for saving energy without significant modification of the system is the combination of partial nitrification and nitrite denitrification, the nitrite shunt process. Compared to denitrification from nitrates, nitrite shunt processes saves up to 40% reduction in carbon, 25% reduction aeration energy, and lower capital cost.
Nitrite denitrification naturally occurs in ammonia-rich wastewater (ammonia ≥100 mgN L−1) treatment processes such as side-stream wastewater treatment processes.20 However, cases for nitrite accumulation and further direct denitrification from nitrite is an emerging concept in mainstream municipal wastewater treatment observed either during nitrite shunt or partial denitrification processes. In the nitrite shunt processes, oxygen is limiting that creates out-competition of the nitrite-oxidizing bacteria by the denitrifying bacteria for nitrite.21,22 However, most of these studies, the nitrite accumulation and nitrite denitrification were not decoupled; hence the much-needed nitrite denitrification kinetics information was not available. Another application that reported nitrite accumulation was during denitrification from nitrate, a process now referred to as a partial denitrification process. In side-stream wastewater treatment, nitrite appears as an end product during nitrate denitrification. Mohan et al.23 studied denitrification of high strength wastewater with acetate and observed significant nitrite accumulation of 400, 1000, 2000, 2700 mgNO2−-N L−1 corresponding to 677, 1354, 2031, 2708 mgNO3−-N L−1 feed nitrate concentration, respectively. Partial denitrification from nitrate has also been reported in municipal wastewater treatment under limited carbon availability. Ge et al.24 observed nitrite accumulation of 22 mgNO2−-N L−1 with glucose during denitrification from nitrate (40 mgNO3−-N L−1) at COD/N of 15 and a maximum accumulation of 17 and 19 mgNO2−-N L−1 with acetate and methanol, respectively, at COD/N of 25. Similarly, a study by Li et al.25 showed more than 90% nitrite accumulation (90 mgNO2−-N L−1) during denitrification from nitrate using pyridine at 2, 4, 9, and 13 COD/N ratios. A high nitrate SDNR of 1.8 mg NO3−-N per mg MLSS d was obtained at all COD/N ratios. In the same process, the nitrite SDNR was 1.15 mgNO2−-N per mgMLSS per day, slightly lower than the nitrate SDNR for all COD/N ratios. However, the authors reported significant nitrite accumulation as the carbon source was exhausted before complete denitrification from nitrite took place. One option to achieve complete denitrification is by adding methanol as a complementary carbon source.
Methanol has been used as a supplementary carbon source for denitrification from nitrate in Europe since 1990 to reduce total nitrogen to 2.2 mg L−1, and in China, since 2003 to meet the environmental regulations for total nitrogen less than 5 mg L−1.26 The selection of methanol over other carbon sources is mainly related to the methanol cost as well as the environmental life cycle analysis (LCA). LCA is used to assess environmental impact parameters: ozone depletion, global warming, acidification, eutrophication, smog formation, ecotoxicity, particulate respiratory effects, human carcinogenic effects, and human non-carcinogenic effects. Methanol has the lowest impact on the LCA parameters compared to ethanol and acetic acid. For instance, the impact of methanol on CO2 emission was 1.4 kg CO2 produced per kg NO3− removed, lower than ethanol (2.07 kgCO2 per kgNO3−), and acetic acid (2.71 kgCO2 per kgNO3−).26 The acetate was found to have the highest impact on the ozone depletion; the ethanol impact was reported as 0.146 kg CFC-11 eq., which is lower than the methanol (0.165 kg CFC-11 eq.) and acetate (0.339 kg CFC-11 eq.) impact.
Ginige et al.27 studied the denitrification from nitrate with methanol as a complementary carbon source in an SBR with different COD/N ratios, i.e., methanol–COD to NO3−-N (MCOD/N) of 0, 0.5, 1.0, 3.3, and TCOD/N of 1.2, 1.3, 2, 4.5. The study showed that the maximum SDNR was 0.36 mgN per mgVSS per d after sludge acclimatization at COD/N of 4.5. The above and other previous denitrification studies addressed the optimization of carbon type and COD/N ratio for complete denitrification from nitrate. To the best of the authors' knowledge, none of the previous research studied the effect of methanol as a complementary carbon source for denitrification of municipal wastewater from nitrite. Such information is essential to optimize denitrification from nitrite. The objectives of this study were to (i) identify the long-term effect of methanol usage as a complementary carbon source on SDNR and nitrite denitrification kinetics parameters, (ii) characterize the short-term performance of biomass grown on complementary methanol addition when fed with either internal carbon source (MWW), methanol or glucose carbon sources and in the presence of nitrite/nitrate as an electron acceptor. The latter will address the biomass's short-term performance under shock conditions where, for example, nitrate, as oppose to nitrite, is the electron acceptor or when fed with other carbon sources for the short term.
2. Materials and methods
2.1 Bench scale setup and operation
A bioreactor (shown in Fig. 1) with a working volume of 10.5 L, equipped with a mechanical stirrer (60 rpm), dissolved oxygen sensor, ORP and pH meters, connected to a control unit, was used in the experiments to ensure anoxic conditions. The system included air diffuser and DO control units for operation at different dissolved oxygen levels; however, for this study, the system was anoxically operated without aeration. The volumes of the influent and effluent tanks were 60 and 50 L, respectively, while a 3.8 L tank was used for supplying nitrite (100 mL min−1 for 2 min) to control the initial nitrite concentration in the reactor.
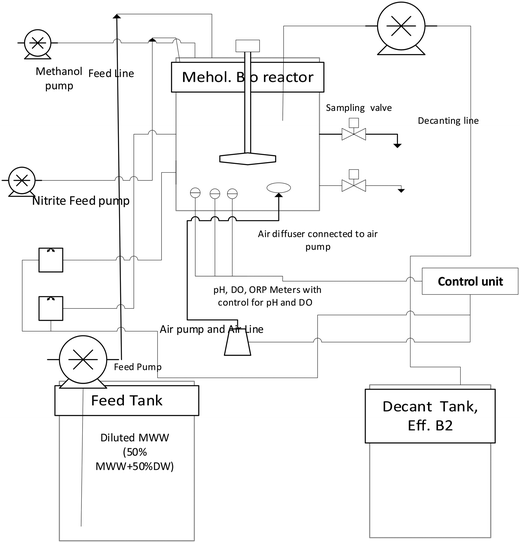 |
| Fig. 1 Bioreactor set up. | |
The influent wastewater was collected from the Greenway Pollution Control Plant (PCP) (London, ON, Canada) every 7–10 days and stored at 4 °C before using. Previous analysis of Greenway's wastewater showed high strength primary effluent with high COD up to 1000 mg L−1, Badia et al.;3 therefore, the reactors were fed with diluted MWW (1
:
1) representing the typical North American wastewater strength, nitrite, and methanol. High purity methanol (99.9%) was added to meet the desired MCOD/TCOD ratio, and the nitrite was added to maintain various COD/N ratios and to characterize the effect of COD/N on the SDNR. A synthetic nitrite stock solution was prepared using potassium nitrite with concentrations of 5000–17
500 mgNO2−-N L−1, corresponding to 20–71 mgNO2−-N L−1 in the SBR. A 99.9% purity methanol Optima™ Fisher Chemical (Fisher Scientific) was used as a complementary carbon source.
The SBR was operated for 110 days in which the system reached a pseudo-steady state (acclimatized biomass) after 73 days or four turnovers of the mean solids retention time (SRT). The SRT was maintained between 14–16 days, which was estimated based on the bioreactor-VSS divided by final effluent-VSS in the decant tank. During the pseudo-steady state period, biomass concentrations varied within 17%; however, despite the four turnovers and less variability of the biomass, a true steady state was not achieved due to the high variability of the influent wastewater. The SBR cycle length was 240 min (6 cycles per day) with 20 min diluted MWW feeding, 1 min nitrite feeding, 1 min pure methanol feed within the desired MCOD/N range, 110 min react phase under complete mix anoxic conditions, 90 min settling, and 20 min decanting time. The fill was around 48%; in each cycle, 5 L was decanted and replaced with 5 L of feed, corresponding to a daily feed flow of 30 L (8.4 h of hydraulic retention time, 10.5 L of effective bioreactor volume). Mixing in the SBR was only used during the reaction period. The bioreactor was operated at room temperature.
2.2 Sampling and analysis
Influent, bioreactor and effluent samples were collected one to two times a week and analyzed for total suspended solids (TSS), volatile suspended solids (VSS), total COD (TCOD), soluble COD (SCOD), ammonia, nitrite (NO2−), total nitrogen (TN), soluble nitrogen (SN), total phosphorus (TP), and soluble phosphorus (SP). Samples were filtered using 0.45 μm membrane filter papers (VWR International, Canada) for soluble component analysis, and 1.2 μm filters were used for TSS and VSS analyses in accordance with standard methods.28 HACH methods were used to measure total phosphorous (method 10127), ammonia (method 10031), total nitrogen (method 10072), nitrite (method 8153), and COD (method 8000). The pH and ORP in the reactor were monitored using Atlas Scientific Sensors.
The bioreactor feed concentration was determined by analyzing the SBR samples at the beginning of the SBR reaction time (time zero) and the end of the SBR cycle as per eqn (1) to (6). The influent nitrite concentration (NO2−-Ni) was the nitrite concentration fed to the system; it was calculated by subtracting effluent nitrite mass from the nitrite mass at time zero and dividing by the feed volume per cycle (eqn (1)). The total feed nitrogen (TNi) concentration was estimated based on the combined concentration of TN in the influent wastewater and the nitrite added in the SBR (eqn (2)). The feed soluble nitrogen concentration (SNi) was calculated similar to TNi, the concentration was estimated based on the combined soluble nitrogen (SN) in the influent wastewater and the nitrite dosed to the SBR (NO2−-Ni) (eqn (3))
|  | (1) |
| TNi = TN (measured in feed tank) + NO2−-Ni | (2) |
| SNi = SN (measured in feed tank) + NO2−-Ni | (3) |
where NO
2−-No and NO
2−-Ne represent the initial and final SBR nitrite concentration at the beginning (time zero) and end of the SBR cycle, respectively.
V1 represents the SBR volume, and
V2 represents the exchange volume or feed volume per cycle (5 L, 48% of SBR volume).
Similarly, the feed COD concentration, including the methanol COD (MCODi), the total MWW + methanol feed COD concentration (TCODi), and the soluble MWW COD (SCOD) + methanol feed COD concentration (SCODi) was calculated according to eqn (4)–(6). The added methanol COD concentration (MCODi) was determined by subtracting effluent SCOD mass (SCODe) and MWW SCOD mass from SCOD mass at time zero in the reactor (SCOD0) and dividing by the feed volume per cycle (eqn (4)).
|  | (4) |
| TCODi = TCOD (measured in feed tank) + MCODi | (5) |
| SCODi = SCOD (measured in feed tank) + MCODi | (6) |
where SCOD
0 and SCOD
e represent the initial and final SBR soluble COD concentration at the beginning (time zero) and end of the SBR cycle.
2.3 Batch tests
A total of 23 offline batch tests and 11 cyclic tests in the SBR were conducted. The cyclic tests were conducted in the SBRs to determine the maximum SDNR and kinetic parameters using carbon source similar to the long-term study (MWW + methanol) at different COD
:
N ratios, each lasting four hours. The offline batch tests were implemented to characterize the short-term performance of biomass grown on complementary methanol addition when fed with either internal carbon source (MWW), methanol or glucose carbon sources and in the presence of nitrite/nitrate as an electron acceptor (Table 1). The 23 offline batch experiments were conducted at the end of the long term SBR study, using a concentrated (centrifuged for 10 min at 1800g) nitrite-methanol + MWW acclimatized biomass from the SBR. The batch experiments were conducted in a 1 L flask, at room temperature and under complete mix (180 rpm) and anoxic condition. The batch tests were conducted in 7 phases; each phase consisted of 4 to 6 parallel experiments that were carried out at the same time. The batch tests were completed over two weeks, including a one day layover between the runs. One liter of distilled water was added to each flask for external carbon source experiments, containing NaHCO3 (120 mg L−1; alkalinity source), KH2PO4, MgSO4·7H2O (100 mg L−1), CaCl2 (100 mg L−1), 1 mL L−1 of trace elements solution (composition in g L−1 for all as: EDTA 15, ZnSO4 0.43, CoCl2 0.24, MnCl2 0.63, CuSO4 0.25, Na2MoO4 0.22, NiCl2 0.19, Na2SeO4 0.21, H3BO3 0.01, and NaWO4 0.05). For tests with the MWW, the design COD/N ratio was maintained by varying NO3−-N and NO2−-N concentrations. For external carbon source-based batch tests, the methanol and glucose varied in concentrations while maintaining a constant nitrite and nitrate concentration. The dissolved oxygen was removed by flushing the flasks for 5 minutes with N2 gas in all the batch experiments. Each test was conducted for 4–18 hours, depending on the time it takes to complete the removal of the substrate (Table 1). Samples were collected from each batch (cyclic and offline) at time zero, and then every 15 minutes for the first 2 hours; after that, samples were collected every 1 to 2 h for COD, nitrite, nitrate, and DO analysis. Alkalinity, ammonia, MLSS, and MLVSS were measured at the start and the end of each batch test. The results were then used to determine the SDNR and the corresponding specific substrate utilization rate (SSUR) at different COD/N ratios for the various test conditions.
Table 1 Batch test summary
Phases |
Electron acceptor |
Carbon source |
COD (mg L−1) |
NO2−-N or NO3−-N (mg L−1) |
C : N ratios |
Number of different C/N cases |
The sum of nitrite and nitrate concentration was maintained at 60 mg L−1, the nitrite and nitrate concentration used varied from 20–60 mg L−1.
A COD/NOX-N ratio of 3, COD/NO3−-N of 5 and COD/NO2−-N of 11 was maintained.
|
1 |
NO2−-N |
MWW |
144–326 |
15.6–85 |
3.6–9.3 |
6 |
2 |
NO3−-N |
MWW |
174–195 |
46–122 |
1.6–4.2 |
4 |
3 |
NO2−-N |
Methanol |
148–1346 |
54–57 |
2.6–24.5 |
5 |
4 |
NO3−-N |
Methanol |
133–1207 |
55–58 |
2.3–21.6 |
5 |
5 |
NO2−-N |
Glucose |
145–1430 |
56–61 |
2.6–23.4 |
6 |
6 |
NO3−-N |
Glucose |
135–1340 |
55–60 |
2.5–22.3 |
5 |
7 |
NO3 + NO2 |
MWW |
192–197 |
60 (20–40 each)a |
3 (5–11)b |
3 |
2.4 Kinetics calculations
The heterotrophic denitrifier yield (YHD – mgVSS per mgCOD) in the SBR and batch tests was calculated as per eqn (7).3,29 | 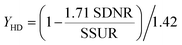 | (7) |
The theoretical yield was also calculated from half-reactions with ammonia as the nitrogen source for biomass, nitrite as the electron acceptor, and methanol as electron donor, as shown in the following overall stoichiometric reaction
0.167CH3OH + 0.167NO2− + 0.17H+ + 0.025HCO3− + 0.025NH4+ = 0.025C5H7O2N + 0.083N2 + 0.39H2O + 0.067CO2 |
The denitrifier yield (YN, mg VSS per mg NO2−-N) were also calculated based on nitrite removed as per eqn (8) | 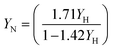 | (8) |
The Monod equation (eqn (9)) was used to determine the maximum specific growth rate (μhmax and half-saturation coefficient (KNO2).30 |  | (9) |
Eqn (9) can be simplified to eqn (10) because DO is close to zero in the study, and the heterotrophic biomass (denitrifiers) is completely anoxic (η = 1, DO ≃ 0.0). Additionally, unlimited COD-substrate (methanol) concentration significantly higher than the reported Ks of 3.8–15 mg L−1 for methanol32,39,41,42 was used in the batch test. |  | (10) |
where KNO2 is the nitrite half-saturation concentration (mg L−1), Ks is the half-saturation concentration for the carbon source-methanol (mg L−1), μhmax is biomass specific growth rate (d−1),
is DO denitrification–inhibition concentration (mg L−1), and Ss, SNO2, S0 are the concentrations of COD (mg L−1), NO2−-N (mg L−1), and DO (mg L−1), respectively.
3. Results and discussion
3.1 SBR denitrification performance overview
The pH and ORP in the reactor were monitored to ensure anoxic conditions during the study; the values remained between 8–9 and −250 to −350 mV, respectively. Although the influent diluted MWW had a pH in the range of 7.0–7.2, the reactor pH increased due to alkalinity production by the denitrification process. According to Metcalf & Eddy,30 the stoichiometric alkalinity removed during nitrification is about 7.14 mgCaCO3 per mgNH3-N-removed and 50% of this amount is recovered during denitrification. In this study, alkalinity produced per NO2−-N removed was 3.27, close to the theoretical value. This relationship between alkalinity and nitrogen removal can be found in the supporting document (Fig. S1†).
The SBR COD and nitrogen profile during the pseudo-steady state are presented in Fig. 2(a) and (b). The summary of influent and effluent quality parameters data can be found in the supporting document (Table S1†). The TCODi (MWW + methanol) varied from 237 to 724 mg L−1 (average 571 ± 332 mg L−1) with an average of 430 ± 134 and 141 ± 112 mg L−1 for municipal wastewater COD and methanol COD, respectively. Similarly, SCODi varied from 54 to 450 mg L−1 (average 214 ± 120 mg L−1), including methanol added to the system. The average primary effluent BOD5 during the study was 232 mg L−1 or 54% of the average measured wastewater TCOD before dilution and methanol addition. The average TCOD and sCOD removed were 68% and 57%, respectively, and the corresponding nitrite removal ranged from 67–100% with an average 96% removal. It can be observed that the carbon source removed was not only due to methanol removal but was also due to an internal carbon source (MWW). This can be observed by comparing the average difference between inf-MCOD and eff-SCOD of 42 mg L−1, and 122 mg L−1 between inf-SCOD and eff-COD.
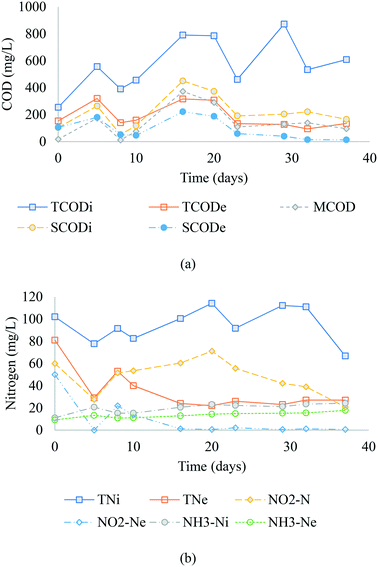 |
| Fig. 2 Influent and effluent (a) COD and (b) nitrogen compounds profile (during the last 40 days of operation, 25 °C). | |
The TNi varied between 66 and 111 mgN L−1 (with an average of 95 mgN L−1), including the 6 to 36 mg L−1 NO2−-N added to the SBR. The average influent soluble nitrogen (SNi) concentration was 72 mg L−1 with NH3-N of 19.2 mg L−1, NO2−-Ni of 48 mg L−1, and soluble organic nitrogen concentrations of 4.8 mg L−1. Conversely, the average effluent soluble nitrogen (SNe) was 23 mg L−1, including 11.5 mg L−1 NH3-N, 9.2 mg L−1 NO2−-N, and 2.3 mg L−1 soluble organic nitrogen. An average 13.5 mg L−1 of TKN (TKNi of 32.5 mg L−1 less TKNe of 19 mg L−1) including, 7.7 mg L−1 of NH3-N and 39 mg L−1 of nitrite, was removed due to anabolic metabolism (assimilation in biomass) and catabolic metabolism (dissimilation to N2), respectively. The average MLSS and MLVSS were 4439 and 3392, respectively (Table S1†). During the pseudo-steady-state period, the MLVSS was 4500 mg L−1 for seven days and stabilized at 3392 for the rest of the operating period.
3.2 Effect of complementary methanol COD to N ratio on nitrite removal efficiency
Fig. 3 and 4 present the relationship between the nitrite removal efficiency and the influent TCOD/N and MCOD/TCOD ratios in the SBR, respectively. The COD/N and MCOD/COD ratios represent the initial TCODi (254–871 mgCOD L−1), MCODi (17–372 mgCOD L−1), and NO2−-Ni (20–60 mgN L−1) in the tests. When the TCOD/N ratio approaches 8.3, the nitrite denitrification efficiency increased to 98%, even with low methanol-mixture content of 20% (Fig. 3). Further addition of methanol to make up 50% methanol–MWW mixture content showed no effect on the nitrite denitrification. However, COD/N lower than 7.5 reduced the denitrification efficiency to less than 70%. It can also be observed that the increase of COD/NO2−-N ratio using methanol as a complementary carbon source improves the denitrification efficiency due to the synergy between MWW and MCOD in denitrification. As discussed in the introduction, there is no previous work conducted on the evaluation of the impact of C
:
N ratio on denitrification from nitrite under combined MWW and methanol carbon source. However, the results from the current study are compared with the previous denitrification with external carbon studies. For example, Akunna et al.,33 found during their denitrification study with glucose (5318 mgCOD L−1), the optimum nitrite and nitrate (50–2500 mgN L−1) removal efficiency of 54 and 70% were at C
:
N ratios of 7.0 and 8.86, respectively. Previous research indicated that complete denitrification using synthetic wastewater required (methanol, ethanol, glycerol, acetate, starch, glucose, saccharose, propionate, lactase) a stable BOD/N ratio of 3 to 3.2 (COD/N of 6–6.5) during the process of denitrification of nitrite.34 Therefore, it is recommended when optimizing the usage of methanol as a complementary carbon source to consider the optimum COD/N ratio to maximize nitrite removal efficiency, which is considered in the following discussion.
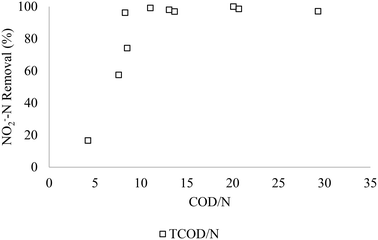 |
| Fig. 3 NO2−-N removal in relation to the COD/N ratio in the SBR. | |
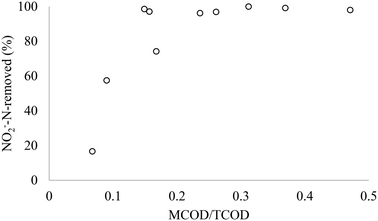 |
| Fig. 4 Effect of MCOD/TCOD on nitrite removal. | |
3.3 Impact of complementary methanol on nitrite denitrification kinetics
At the end of the continuous SBR study, 11 cyclic tests in the SBR were conducted to identify the impact of complementary methanol on nitrite denitrification kinetics parameters, including the denitrifier yield, maximum SDNR, and half-saturation coefficient. During this period, the biomass was considered to be fully acclimatized for nitrite. This was indicated through the stable MLVSS data shown in Fig. S2† and also reflected by the % NO2−-N removal, which indicates the stabilization of the denitrifying SBR and the % N removal at various COD/N ratios (Fig. 3) for methanol and the TCOD (Fig. 4).
3.3.1 Maximum specific denitrification rate (SDNR).
With the variation of internal COD concentrations of MWW during our experiments, we also varied the ratio of MCOD/TCOD in the cyclic test in order to examine the optimum ratio for the maximum SDNR. The COD/N ratios represent the initial TCOD, SCOD, NO3−-N, and NO2−-N in the tests. The SDNRs were calculated by dividing the slope of the line of nitrite concentration removed over time (mgNO2−-N L−1 per day) during the SBR anoxic cycle by the MLVSS concentration. Similarly, the specific substrate (sCOD) uptake rates (SSURs) were calculated by dividing the slope of the line of COD concentration removed over time (mgCOD L−1 per day) during the SBR anoxic cycle by the MLVSS concentration.
During the SBR cyclic tests, the initial SCOD/N ratios ranged from 1.8 to 9.5, and TCOD/N of 2–12.5 after considering particulate COD. It must be asserted that the SSUR was calculated based on SCOD removed, taking into consideration of the MCOD and the COD hydrolysed from particulate organics (average influent VSS) using a hydrolysis coefficient of 1.8 day−1.3,35,36 The adjusted TCOD/N was estimated by adding a COD of 1.42 gCOD per gVSS to the measured SCOD during each 4 h cycle, yielding the TCOD/N ratio of 2–12.5. In the range of COD/N ratios, SDNR varied between 0.01 and 0.5 mgN per mgVSS per day (Fig. 5). According to Fig. 5, the optimum SDNR occurred at COD/N ratio of 5.9. The SDNR with MWW and methanol linearly increased with the increase in TCOD/N ratio in the 2.0–5.9 range. There was an optimum TCOD/N ratio beyond which SDNR decreased. The main reason for the decrease of SDNR is related to the high KNO2 of 9.2 mgN L−1 derived from the kinetic studies (section 3.3.3), and therefore, the observed decline in SDNR with MWW can be explained by the low ambient NO2−-N concentrations relative to KNO2, as per the Monod model. The maximum SDNR of 0.52 mgN per mgVSS per day higher than the 0.03–0.3 mgN per mgVSS per d reported in the previous denitrification from nitrite studies using external carbon sources,3,24,37–39 and comparable to the value reported by Beccari et al.40 The maximum SDNR was achieved at MCOD/TCOD of 0.64, meaning with 64% of the organic carbon coming from methanol. The relatively higher SDNR in this study showed synergy between methanol as a supplementary carbon source and the MWW. In summary, the results showed that with an increase of the MCOD/TCOD to 50–60%, a 7.4 fold improvement of SDNR could be achieved compared to the SDNR in using MWW alone as a carbon source (SDNR = 0.07 mgN mgVSS−1 d, Badia et al.3), which can make huge savings in the anoxic bioreactor volume, mixing equipment and power.
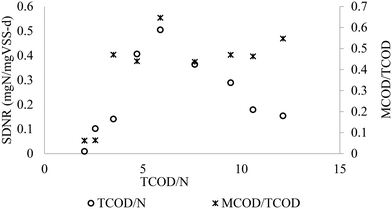 |
| Fig. 5 Effect of COD/N and MCOD/TCOD ratio on the SDNR. | |
3.3.2 Heterotrophic denitrifier yield (YHD).
The observed heterotrophic denitrifier yield (YHD) in the SBR was estimated after determining the slope of the SDNR/SSUR (data in ESI† document, Fig. S3) and substituting the value in eqn (7). The YHD was found to be 0.39 mgVSS per mgTCOD for methanol + MWW. Further, based on the estimated YHD of 0.39 mgVSS per mgTCOD for the mixture of methanol and municipal wastewater, the removed TCOD of 383 mg L−1 (from Table S1†), and the estimated nitrogen content of VSS (0.1 mgN per mgVSS), the TKN consumed for cell growth was estimated as 16 mgN L−1. On another note, the YHD, according to the 13.6 mg L−1 average ammonia removed (TKN used for biomass = (influent NH3 – effluent NH3)/0.6) in the SBR resulted in YHD = 0.35, close to the estimated value that is comparable to the yield of MWW.3 The YHD is lower than the 0.46 obtained for methanol when used in post denitrification (data not shown), which indicates that using methanol as a complementary carbon source improves not only the SDNR but also lowers the sludge produced compared with methanol usage in the post denitrification. The current nitrite denitrifier yield value was compared with the nitrate denitrifier yield using various carbon sources. Peng et al.2 estimated a yield 0.4, 0.42, and 0.65 mgVSS per mgCOD for methanol, ethanol, and acetate, respectively, which is close to the yield estimated in this study. Other yield studies by Guven et al.42 reported a yield coefficient of 0.45 mgVSS per mgCOD for a mixture of acetate, propionate, ethanol, and glucose, and Sobieszuk et al.43 reported 0.44 mgVSS per mgCOD for methanol. Both were studies for the denitrification from nitrate, which are in good agreement with the yield estimated in the current study. On another note, Bernat et al.44 reported a daily variation on yield (ranging from 0.27 to 0.44), which depended on the daily operation conditions and COD/N ratio. Using eqn (8), the denitrifier yield (YN, mgVSS per mgNO2−-N) was estimated as 2.27 and 1.48 mgVSS per mgN for methanol only (post denitrification) and methanol with MWW (pre-denitrification), respectively. The estimated values were higher than the estimated YN of 1.062 mgVSS per mgNO2−-N using MWW only,3 lower for values that made use of acetate 3.16 mgVSS per mgNO2−-N as a carbon source and comparable to Kornaros et al.45 that stated a yield YN of 1.41 mgVSS per mgNO2−-N in a kinetic study using glutamate. The literature showed that the acetate yield is higher than the methanol or MWW yield; in this study, the combination of methanol and MWW provide yield close to the MWW only yield (0.33 mgVSS per mgCOD, Badia et al.3).
3.3.3 Half saturation concentration and biomass specific growth rate.
The Monod equation (eqn (9)) was used to determine other kinetic parameters such as the maximum specific growth rate μhmax and half-saturation coefficient (KNO2).30 The analysis was based on 4 h-10 cyclic tests in a post-denitrifying-SBR with methanol as a sole carbon source and nitrite as an electron acceptor. The μhmax can be directly related to YN (2.27 mgVSS per mgNO2−-N) and post denitrification SDNRmax (maximum SDNR) of 0.62 mgNO2−-N per mgVSS per d, according to the relation of SDNRmax = μmax/YN. Hence, μhmax was obtained as 1.4 d−1 for methanol and nitrite in the post-denitrification SBR. The value is higher than the previously reported value from denitrification with nitrate, i.e., 0.5 d−1,46 however close to the estimated 1.3 d−1 (ref. 47) and 1.25 d−1.48 The obtained μhmax is in accordance with the reported values (0.5–4 d−1) for different external carbon sources.13,31,45,47,49 Using eqn (8) with the replacement YH by YN, the Lineweaver–Burk plot was developed (eqn (11) and Fig. 6) to determine KNO2. | 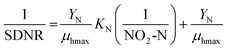 | (11) |
Substituting SDNRmax with μhmax/YN, KN was determined as 9.3 mg L−1. This explains why complete removal of NO2−-N was not possible in the SBR during the experimental period, and this was due to the switching function of NO2−-N/(KN + NO2−-N), effluent NO2−-N is 9.2 mg L−1 (Table S1†). Thus, in order to decrease the effluent nitrite concentration, the SBR system needs to be optimized by increasing reaction time, SRT, and biomass concentrations. The reported half-saturation coefficients (KNO2 and KNO3) are 0.9 mgNO2−-N L−1 and 1.4 mgNO3−-N L−1 for acetate50 and 0.28 mg NO2−-N L−1 and 0.77 mgNO3−-N L−1 for L-glutamic acid.45 However, Her and Huang51 reported KNO2 of 10.9 mg L−1 and KNO3 of 14.3 mg L−1 with methanol, which is comparable to the values reported in this work. Badia et al.3 determined the KNO2 value of 4.07 mgN L−1 for MWW. The high variability in KNO2 is mainly due to the testing conditions, including carbon source type and concentrations and COD/N range. The reported KNO2 for acetate and glutamic acid were much lower than the ones determined for methanol and MWW. It is important to note that the KNO2 reported for acetate and glutamic study were during denitrification from nitrate as oppose to nitrite as being the sole electron acceptor (this study). Thus, the much lower KNO2 values for acetate and glutamic acid could be a function of the carbon source and, to some extent, due to the nitrate denitrifier biomass diversity.
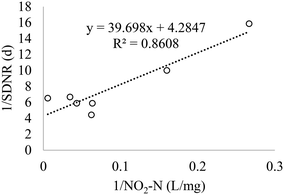 |
| Fig. 6 Lineweaver–Burk plot for kinetic parameters determinations. | |
3.4 Batch tests
The batch tests were conducted to characterize the performance of biomass acclimatized to nitrite as an electron acceptor and methanol as a complementary carbon source upon short-term exposure to a different carbon source or an electron acceptor. The carbon sources studied include MWW only, methanol, and glucose, whereas the alternative electron acceptor was nitrate. Any of these conditions could happen in a practical setting, and it would be of benefit to understand how the nitrite acclimatized biomass performs under such circumstances. There was no nitrite removal with glucose after 8–24 hours of sampling; therefore, results are not discussed further in this paper.
3.4.1 Short-term nitrite or nitrate denitrification using MWW as a sole carbon source.
Six batch tests were conducted at room temperature to investigate the acclimatized biomass denitrification potential from nitrite and the actual impact of carbon source change on SDNR from the combination of methanol and MWW to MWW as a sole carbon source (phase 1, Table 1). The tests were conducted by employing different nitrite concentrations (15.6, 22.8, 37, 58, 71 and 85 mgN L−1) to achieve COD/N ratios of 3.6, 4.3, 5.3, 7.7, 6.3, and 9.3 at MWW–TCOD concentrations 144, 144, 286, 307, 326 and 305 mg L−1, respectively. The maximum SDNR showed 0.47 mgN per mgVSS per d at COD/N of 5.3 (Table 2 and ESI† document Fig. S4a and b). It is of importance to mention that B1 and B2 experiments were conducted one week before the other (B3–B6) batch tests. The estimated yield coefficient and kinetic parameters were YH of 0.52 mgVSS per mgCOD, μmax of 1.23 d−1, and KNO2 of 6.48 mgN L−1. The denitrifier yield is within the range obtained in the SBR, which can be related to the biomass type developed within the SBR. The SDNR and the yield were as high as the values measured in the SBR even though the batch reactor was primarily fed with MWW. The half-saturation coefficient (KNO2) was higher than the one observed for MWW of 4.07 mg L−3 and lower than the one obtained using methanol (9.3 mg L−1). It is also clear that for the MWW-nitrite range, the optimum COD/N ratio for the highest SDNR was 5.3 (Table 2), which was close to the COD/N in the SBR (5.88). The ΔCOD/ΔN (SSUR/SDNR) showed an inverse relation to COD/N, while COD/N decreased from 9.3 to 3.6, ΔCOD/ΔN increased from 5.5 to 7.6. A similar trend was observed from the long-term performance with methanol + MWW as carbon source; however, while the trend is consistent, the magnitude of ΔCOD/ΔN seems to be more carbon source specific. The above results showed that the MWW as a sole carbon source behaved similarly to the SBR study that used MWW + methanol and better compared to the earlier studies that used MWW as a sole carbon source. The result implies that (i) the kinetics is highly dependent on the nature of the biomass: the biomass acclimatized with methanol + MWW behaved differently compared with the MWW acclimatized biomass, and (ii) a short term switch from the MWW + methanol to MWW as a sole carbon source, will not affect the denitrification performance.
Table 2 Batch tests: MWW with nitrite and nitrate
Parameter |
Nitrite and municipal wastewater |
Nitrate and municipal wastewater |
B1 |
B2 |
B3 |
B4 |
B5 |
B6 |
B1 |
B2 |
B3 |
B4 |
TCOD mg L−1 |
144 |
144 |
286 |
307 |
326 |
305 |
195 |
193 |
174 |
195 |
CODf mg L−1 |
72 |
63 |
132 |
154 |
162 |
213 |
43 |
47 |
38 |
70 |
NO2/NO3 mgN L−1 |
15.6 |
22.8 |
37 |
58 |
71 |
85 |
46 |
64 |
86 |
122 |
NO2f/NO3f mgN L−1 |
0.9 |
0.9 |
13 |
20 |
68 |
70 |
2 |
22 |
50 |
50 |
MLSS mg L−1 |
8500 |
6600 |
1030 |
1060 |
1010 |
1050 |
820 |
750 |
910 |
860 |
MVSS mg L−1 |
4080 |
3700 |
790 |
820 |
810 |
820 |
650 |
620 |
740 |
720 |
SDNR mgN per mgVSS d |
0.08 |
0.18 |
0.46 |
0.47 |
0.25 |
0.31 |
0.14 |
0.14 |
0.11 |
0.15 |
COD/N |
9.3 |
6.3 |
7.7 |
5.3 |
4.3 |
3.6 |
4.2 |
3.0 |
2.0 |
1.6 |
ΔCOD/ΔN |
5.5 |
2.8 |
6.3 |
6.2 |
8.1 |
7.6 |
4.4 |
5.2 |
4.5 |
5.6 |
Initial ALK. mgCaCO3 |
280 |
280 |
430 |
430 |
430 |
430 |
418 |
418 |
418 |
418 |
Final ALK. mgCaCO3 |
345 |
346 |
581 |
576 |
576 |
577 |
640 |
620 |
630 |
620 |
Similarly, four batch tests were conducted with MWW and nitrate (phase 2, Tables 1 and 2). The tests were conducted under varying COD/N ratios of 1.6, 2, 3, and 4.2 by employing various nitrate concentrations (46, 64, 86 and, 122 mgN L−1) and TCOD concentrations of 195, 193, 174 and 195 mg L−1 (Fig. S4†). All SDNR values were very close at the employed COD/N ratios of 1.6–4.2. The maximum nitrate SDNR (0.15 mgN per mgVSS per d) was lower than the corresponding nitrite SDNR (0.47 mgN per mgVSS per d); however, we attribute the decrease of SDNR could be due to the nature of the biomass and the low COD/N ratio. It is plausible that the optimum SDNR was not wholly achieved due to the lower COD/N data range (1.6–4.2) used in this test. Akunna et al.33 obtained the optimum SDNR with glucose at COD/N of 8.86 and 7 for nitrate and nitrite, respectively. The yield coefficient, according to the 4 data points, was found to be 0.3 ± 0.05 mgVSS per mgCOD, which agrees with Muller et al.52 The yield of 0.3 was lower than the nitrite yield; this is per the stoichiometry.
During the tests with nitrite and nitrate, dissimilatory nitrate or nitrite reduction to ammonium (DNRA) was observed, and ammonia concentration increased at the end of each test (5–11 mgNH4-N L−1). According to van den Berg et al.53 and Kraft et al.,54 the leading cause that affects the dissimilatory nitrite/nitrate reduction–denitrification to ammonia is the nirF enzyme. Baideme et al.55 who studied the condition of the ammonia restoration during denitrification reported a relative abundance of nirF with an accumulation of ammonia when sludge age (SRT) exceeded 12 days. In this study, alkalinity changes were related to the accumulation of ammonia, and alkalinity recovery per ammonia increase was 7.14 mgCaCO3 per mgN, higher than the theoretical recovery of 3.57 mgCaCO3 per mgN denitrified from denitrification. The DNRA ranged between 5–11 mgNH3-N, corresponding to 35–78 mgCaCO3 alkalinity restored.
3.4.2 Short-term nitrite and nitrate denitrification using methanol as a sole carbon source.
Additional batch tests were conducted to the ones discussed above in order to discern the short-term impact of carbon source change, from the combination of methanol and MWW to methanol as a sole carbon source, on the SDNR (phases 3 and 4, Tables 1 and 3). The maximum SDNR in the main SBR of 0.52 mgN per mgVSS per d occurred at optimum COD/NO2−-N of 5.88 based on biomass acclimatized for nitrite fed with a mixture of methanol and MWW. Using the acclimatized biomass, the nitrite denitrification with methanol as a sole carbon source showed a relatively lower SDNR ranging between 0.27 to 0.38 mgN per mgVSS d SDNR (Table 3) but a comparable yield coefficient of YH = 0.34 mgVSS per mgCOD. The half-saturation coefficient was not obtainable because the nitrite concentration used was higher than the KNO2, which was chosen based on the requirement of the SDNR data. The above result confirmed the observed synergy between methanol + MWW, which resulted in higher SDNR compared to the methanol addition as a sole carbon source. The main reason for the reduction in SDNR could be attributed to the biodiversity of biomass that is acclimatized to both carbon sources together in the SBR, and when exposed to a methanol carbon source (methanol) in the short term test led to the μmax reduction while YH remained the same. It can also be observed, the ΔCOD/ΔN (SSUR/SDNR) has an inverse relation to COD/N, while COD/N increased from 2.6 to 18.1, ΔCOD/ΔN decreased from 4 to 2.1 (Table 3). The highest SDNR of 0.38 mgN per mgVSS per d occurred at COD/N of 2.6 with a relative consumption of ΔCOD/ΔN (SSUR/SDNR) of 4.0 higher than the consumption in the SBR (2.8), which can be attributed to the lower SDNR while the COD consumption (SSUR) is almost similar. On the other hand, it can be observed that the relation between COD/N and SDNR is more carbon source-specific; in our case, the SBR-COD/N and the COD/N-batch are different.
Table 3 Batch tests: Methanol with nitrite and nitrate
Parameter |
Nitrite and methanol |
Nitrate and methanol |
B1 |
B2 |
B3 |
B4 |
B5 |
B1 |
B2 |
B3 |
B4 |
B5 |
TCOD mg L−1 |
148 |
459 |
723 |
996 |
1346 |
133 |
356 |
603 |
912 |
1207 |
CODf mg L−1 |
83 |
415 |
651 |
905 |
1234 |
45 |
281 |
557 |
751 |
1109 |
NO2/NO3 mgN L−1 |
57 |
55.2 |
54 |
55 |
55 |
57.1 |
57 |
58 |
55 |
56 |
NO2f/NO3f mgN L−1 |
31.2 |
33.6 |
31 |
31 |
32 |
33 |
36 |
34 |
34 |
31.4 |
MLSS mg L−1 |
820 |
750 |
910 |
860 |
880 |
750 |
720 |
850 |
800 |
830 |
MVSS mg L−1 |
650 |
620 |
740 |
720 |
740 |
660 |
640 |
750 |
700 |
740 |
SDNR mgN per mgVSS d |
0.38 |
0.32 |
0.28 |
0.30 |
0.27 |
0.34 |
0.37 |
1.06 |
0.36 |
0.38 |
COD/N |
2.6 |
8.3 |
13.4 |
18.1 |
24.5 |
2.3 |
6.3 |
10.4 |
14.0 |
21.6 |
ΔCOD/ΔN |
4.0 |
3.4 |
3.3 |
2.1 |
4.9 |
4.2 |
4.7 |
1.5 |
7.4 |
4.0 |
Initial ALK. mgCaCO3 |
66 |
64 |
64 |
64 |
64 |
64 |
62 |
64 |
64 |
64 |
Final ALK. mgCaCO3 |
126 |
124 |
112 |
124 |
124 |
84 |
88 |
124 |
96 |
94 |
Similarly, five batch tests were conducted with methanol and nitrate (phase 4, Table 3) as a carbon source and electron acceptor, respectively. The highest SDNR among the five nitrate batches were 1.06 mgN per mgVSS per d (Table 3) and in ESI† document Fig. S5) occurred at the typical optimum COD/NO3−-N of 10 and the minimum ΔCODconsumed/ΔNreduced of 1.5. The nitrate SDNR was much higher than the one reported in section 3.4.1 (0.15 mgN per mgVSS d), confirming that the lower SDNR has associated with the C
:
N ratio as opposed to the nature of the biomass. It indicates that biomass acclimatized with nitrite can also denitrify nitrate with high performance; however, the maximum SDNR was achieved at a higher COD/N (10) in the case of nitrate vs. 5.88 in the case of nitrite. The high COD/N can be attributed to the higher COD required for denitrification from nitrate due to extra 2-electrons transferred during the bioreaction. Though the COD/N ratio has a wide range (2.6–24.5), the removal rate ΔCOD/ΔN was high 4.0 and 4.9 at low COD/N (2.6) and high COD/N (24.5), which can be attributed to the higher nitrate-SDNR and SSUR. The yield coefficient parameter was estimated as YH = 0.28 mgVSS per mgCOD. The yield was also calculated according to the half-reactions (with nitrate as the nitrogen source for biomass and nitrate as the electron acceptor):
0.167CH3OH + 0.1NO3− + 0.1H+ = 0.0218C5H7O2N + 0.039N2 + 0.307H2O + 0.058CO2 |
From the above equation,
YH is 0.28 mgVSS per mgCOD, which matched exactly with the estimated experimental yield value. The lower nitrate yield was in agreement with the yield reported in section 3.4.2 and also comparable to the stoichiometric yield. The alkalinity produced during methanol/nitrate experiments matches the ratio of 3.57 mgCaCO
3 per N
denitrified.
Further, the alkalinity produced during methanol experiments did not show ammonia release, indicating that DNRA is not only affected by the sludge age but also the carbon source type. Various studies reported DNRA phenomena during denitrification at different carbon sources, i.e., organic soil56 and Nizzoli,57 glycerol,55 and glucose.58
3.4.3 Short-term combined nitrite and nitrate denitrification using MWW as a sole carbon source.
Three batch tests were also conducted to examine SDNR from a mixture of nitrate and nitrite with MWW with three different NO2
:
NO3 ratios of 1
:
2, 1
:
1, and 2
:
1, with nitrite concentrations of 20, 40 and 60 mgN L−1 (NO2−-N + NO3−-N = 60 mgN L−1), maintaining a COD/NOx ratio of 3 (Table 4). In the three batches, nitrite was reduced sharply while the nitrate removal was minimal. It can also be seen that the highest nitrite SDNR was 50% lower than the one observed when nitrite was used as the only electron acceptor, indicating that nitrite and nitrate existence partially inhibited the denitrification rate with decreasing both nitrite and nitrate SDNRs.
Table 4 Batch tests: MWW and nitrite/nitrate
Parameter |
Batches |
B1 |
B2 |
B3 |
TCOD, mg L−1 |
197 |
192 |
193 |
CODf, mg L−1 |
53 |
57 |
67 |
NO2−-N, mg L−1 |
20 |
30 |
40 |
NO2−-Nf, mg L−1 |
0 |
0 |
0.3 |
NO3−-N, mg L−1 |
40 |
30 |
20 |
NO3−-Nf, mg L−1 |
32.6 |
27.5 |
11.5 |
VSS, mg L−1 |
950 |
920 |
950 |
SDNR, mgNO2−-N per mgVSS d |
0.21 |
0.21 |
0.26 |
SDNR, mgNO3−-N per mgVSS d |
0.037 |
0.006 |
0.014 |
SDNR, mgNOx−-N per mgVSS d |
0.21 |
0.260 |
0.234 |
COD/NO2−-N |
11 |
7 |
5 |
COD/NO3−-N |
5 |
6 |
8 |
COD/NOx−-N |
3 |
3 |
3 |
ΔCOD/ΔNO2−-N |
9.7 |
8.4 |
6.2 |
ΔCOD/ΔNO3−-N |
54 |
275 |
112 |
ΔCOD/ΔNOx−-N |
9.7 |
6.7 |
6.9 |
Initial ALK, mgCaCO3 L−1 |
373.5 |
373.5 |
373.5 |
Final Alk, mgCaCO3 L−1 |
437 |
418 |
470 |
Fig. 7 shows the denitrification rates of nitrite and nitrate at the NO3/NO2 ratio of 2 (B1). The results indicate that when high nitrate and nitrite was present, nitrate denitrification was limited until nitrite concentration reduces to a lower level (below 6 mg L−1). Compared to observations in sections 3.4.1 and 3.4.2, a lower nitrate-SDNR was obtained (0.037mgN per mgVSS per d, Fig. 7 and S6,†Table 4). Mehrabi et al.21 reported similar, lower nitrate denitrification performance under the presence of nitrate and nitrite in a nitrite acclimatized biomass. The lack of nitrate denitrification could be due to the biomass that was acclimatized to nitrite resulting in a dominance of specific types of nitrite denitrifier species; however, this needs further investigation. Another reason for nitrate denitrification inhibition suggested by Tian et al.59 is that nitrite is very toxic to the denitrifying microorganisms, and due to this toxicity, the nitrite concentration inside the cells is much lower than the bulk liquid nitrite concentration. Due to this condition, microorganisms use nitrite as an electron acceptor instead of nitrate to reduce the bulk-liquid external toxicity stress from nitrite. However, recent partial denitrification results from nitrate and a rather accumulation of nitrite over nitrate do not support the latter claim.60
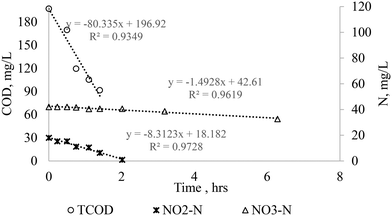 |
| Fig. 7 Denitrification rates of a mixture of nitrite and nitrate with MWW (B1). | |
3.5 Making a case for complementary carbon source addition: capital and operating cost analysis
Methanol, as a complementary carbon source, can be employed in low concentration in the pre-denitrification and with a high concentration in the post-denitrification. The methanol addition in the pre-denitrification is used when TCOD/N is lower than the pre anoxic zone denitrification capacity and the addition of methanol, i.e., the MCOD/TCOD must be adjusted to minimize the operation cost. Similarly, using methanol in the post-denitrification will result in higher capital cost for an extra anoxic tank (concrete and mixing equipment) in addition to the operational cost of methanol when MCOD/TCOD of 1. Based on the results in this study, the COD/N ratios can be categorized into three levels: low level of 0–3, a medium of 4–7, and a high level ≥8 (Table 5). The highest SDNR 0.4–0.52 mgN per mgVSS per d would occur when MCOD/TCOD in the range of 0.4–0.6 (medium range of TCOD/N), i.e., where methanol was used as a complementary carbon source in the pre-denitrification anoxic tank (Fig. 8 and Table 5). Moreover, high SDNR of 0.32 mgN per mgVSS d with methanol alone would be considered when methanol is used as the sole carbon source in the post denitrification.
Table 5 Cost analysis for denitrification with methanol as a complementary carbon source
Parameter |
Low COD/N |
Mid COD/N |
High COD/N |
Higher at MCOD/TCOD of 1.0.
Higher at MCOD/TCOD of 0.5.
Higher at MCOD/TCOD of 0.5 and COD/N of 8–9.
|
COD/N-range |
0–3.0 |
4.0–7.0 |
≥8.0 |
SDNR, mgN per mgVSS per d |
0.01–0.38a |
0.36–0.5b |
0.15–0.38c |
 |
| Fig. 8 Effect of MCOD/N on the SDNR. | |
According to the cost of methanol addition of 2.5 CAD per gallon,61 the cost comparison between using the methanol in the pre and post nitrite denitrification based on the methanol added to achieve the respective maximum SDNR's of 0.52 and 0.38 mgN per mgVSS d was similar. However, the higher SDNR in the pre nitrite denitrification is translated to 37% volume and mixing power reduction (ESI† document, Table S2), which can be translated to a capital cost reduction associated with civil infrastructure and operating cost reduction associated with mixing power. Furthermore, when we compare the cost of methanol as an external complementary carbon source in the pre-anoxic reactor (SDNR of 0.52 mgNO2−-N per mgVSS per d) to the internal carbon source of MWW (SDNR of 0.07 mgNO2−-N per mgVSS d), the anoxic volume will be reduced by 86% of reactor volume. However, the methanol supply shall be added to the operating cost, but its continuous addition may not be required, as demonstrated in the batch reactors that showed higher SDNRs even after switching the carbon source from MWW + methanol to just MWW.
Nomenclature
MWW | Municipal wastewater |
DO | Dissolved oxygen concentration, mgO2 L−1 |
FNA | Free nitrous acid, mgHNO2 L−1 |
K
NO2
| Half-saturation concentration for nitrite, mgN L−1 |
K
s
| Half-saturation concentration for COD, mgCOD L−1 |
| DO denitrification-inhibition concentration, mgO2 L−1 |
RSD | Relative standard deviation % |
MLSS | Mixed liquor suspended solids, mgSS L−1 |
MLVSS | Mixed liquor volatile suspended solids, mgVSS L−1 |
Nitrite Monod switching function |
|
SDNR | Specific denitrification rate, mgN per mgVSS per d |
SN | Soluble nitrogen, mgN L−1 |
SNi | Influent soluble nitrogen, mgN L−1 |
SNe | Effluent soluble nitrogen, mgN L−1 |
SN0 | Initial soluble nitrogen concentration measured at time zero in the SBR, mgN L−1 |
NO2−-Ni | Influent NO2−–N, mgN L−1 |
NO2−-N0 | Initial NO2−–N concentration measured at time zero in the SBR, mgN L−1 |
SCOD | Soluble COD, mgCOD L−1 |
SP | Soluble phosphorus, mgP L−1 |
SSUR | Specific substrate utilization rate, mgCOD per mgVSS d |
TCOD | Total COD, mgCOD L−1 |
TN | Total nitrogen, mgN L−1 |
TNi | Influent total nitrogen, mgN L−1 |
TNe | Effluent total nitrogen, mgN L−1 |
TN0 | Initial total nitrogen concentration measured at time zero in the SBR, mgN L−1 |
TP | Total phosphorous, mgP L−1 |
Y
HD
| Biomass yield coefficient based on COD removed, mgVSS per mgCOD |
Y
N
| Biomass yield coefficient based on nitrite removed, mgVSS per mgN |
μ
hmax
| Biomass specific growth rate, d−1 |
Conclusion
Due to the low carbon to nitrogen ratio in MWW, the denitrification process in full-scale wastewater treatment plants employs external carbon sources to minimize total nitrogen in the effluent. This comprehensive study demonstrated that compared to MWW as a sole carbon source, denitrifier's kinetics (the half-saturation nitrite denitrification coefficient, denitrifier yield, and maximum specific growth rate) and its activity (SDNR) was higher with MWW + methanol, which enhanced the denitrification performance. Batch studies also confirmed that a short-term switch from MWW + methanol to MWW as a sole carbon source would not affect the denitrification performance, creating the concept of intermittent methanol addition. Comparatively, upon using methanol as a sole carbon source, a lower SDNR (0.38 mgNO2−-N per mgVSS per d) was achieved, which implies that when methanol is supplemented with the MWW, a synergic effect occurs which enhances the SDNR. The observed higher SDNR rates and associated cost savings indicated that the addition of methanol as a complementary carbon source for nitrite denitrification could be considered as a mainstream process even for conditions carbon is not limiting in the MWW.
Conflicts of interest
There are no conflicts to declare.
References
- J.-J. Her and J.-S. Huang, Influences of carbon source and C/N ratio on nitrate/nitrite denitrification and carbon breakthrough, Bioresour. Technol., 1995, 54, 45–51 CrossRef CAS.
- Y. Peng, M. A. Yong and S. Wang, Denitrification potential enhancement by addition of external carbon sources in a pre-denitrification process, J. Environ. Sci., 2007, 19, 284–289 CrossRef CAS.
- A. Badia, M. Kim, G. Nakhla and M. B. Ray, Effect of COD/N ratio on denitrification from nitrite, Water Environ. Res., 2019, 91, 119–131 CrossRef CAS.
- J. S. Almeida, M. A. M. Reis and M. J. T. Carrondo, Competition between nitrate and nitrite reduction in denitrification by Pseudomonas fluorescens, Biotechnol. Bioeng., 1995, 46, 476–484 CrossRef CAS.
- H. W. Zhao, D. S. Mavinic, W. K. Oldham and F. A. Koch, Controlling factors for simultaneous nitrification and denitrification in a two-stage intermittent aeration process treating domestic sewage, Water Res., 1999, 33, 961–970 CrossRef CAS.
- M. Zhang, S. Wang, B. Ji and Y. Liu, Towards mainstream deammonification of municipal wastewater: Partial nitrification-anammox versus partial denitrification-anammox, Sci. Total Environ., 2019, 692, 393–401 CrossRef CAS.
- I. Schmidt, O. Sliekers, M. Schmid, E. Bock, J. Fuerst, J. G. Kuenen, M. S. Jetten and M. Strous, New concepts of microbial treatment processes for the nitrogen removal in wastewater, FEMS Microbiol. Rev., 2003, 27, 481–492 CrossRef CAS.
- J. L. Campos, J. Dumais, J. P. Pavissich, O. Franchi, D. Crutchik, M. Belmonte, M. Faúndez, L. Jorquera, A. Pedrouso and A. Mosquera-Corral, Predicting accumulation of intermediate compounds in nitrification and autotrophic denitrification processes: A chemical approach, BioMed Res. Int., 2019, 2051986 CAS.
- C. M. Castro-Barros, M. Jia, M. C. van Loosdrecht, E. I. Volcke and M. K. Winkler, Evaluating the potential for dissimilatory nitrate reduction by anammox bacteria for municipal wastewater treatment, Bioresour. Technol., 2017, 233, 363–372 CrossRef CAS.
- J. Liu, Y. Yang, S. Zhu, S. Kuang and K. Wang, An autotrophic nitrogen removal process: Short-cut nitrification combined with ANAMMOX for treating diluted effluent from an UASB reactor fed by landfill leachate, J. Environ. Sci., 2010, 22, 777–783 CrossRef CAS.
- W. Zeng, X. Bai, L. Zhang, A. Wang and Y. Peng, Population dynamics of nitrifying bacteria for nitritation achieved in Johannesburg (JHB) process treating municipal wastewater, Bioresour. Technol., 2014, 162, 30–37 CrossRef CAS.
- S. Fudala-Ksiazek, A. Luczkiewicz, K. Fitobór and K. Olanczuk-Neyman, Nitrogen removal via the nitrite pathway during wastewater co-treatment with ammonia-rich landfill leachates in a sequencing batch reactor, Environ. Sci. Pollut. Res., 2014, 21, 7307–7318 CrossRef CAS.
- Q. Yang, Y. Peng, X. Liu, W. Zeng, T. Mino and H. Satoh, Nitrogen removal via nitrite from municipal wastewater at low temperatures using real-time control to optimize nitrifying communities, Environ. Sci. Technol., 2007, 41, 8159–8164 CrossRef CAS.
-
A. Onnis-Hayden, D. Dair, C. Johnson, A. Schramm and A. Z. Gu, in Proceedings of the 80th Annual Water Environment Federation Technical Exhibition and Conference, San Diego, California, Oct, 2007, pp. 13–17.
-
G. T. Daigger, A. L. Carslon, X. Chen and B. R. Johnson, Coupled anoxic suspended growth and membrane aerated biofilm reactor process options, 2019, 12.
- R. Du, S. Cao, B. Li, H. Zhang, S. Wang and Y. Peng, synergy of partial-denitrification and anammox in continuously fed upflow sludge blanket reactor for simultaneous nitrate and ammonia removal at room temperature, Bioresour. Technol., 2019, 274, 386–394 CrossRef CAS.
- D. Gao, Y. Peng and W.-M. Wu, Kinetic model for biological nitrogen removal using shortcut nitrification-denitrification process in sequencing batch reactor, Environ. Sci. Technol., 2010, 44, 5015–5021 CrossRef CAS.
-
R. Fofana, B. Peng, H. Huynh, K. Jones, A. Al-Omari, C. Bott, S. Murthy and B. Wett, Media selection for enrichment of anammox in polishing filters, WEFTEC, 2019, 1–9.
- C. Wu, Z. Chen, X. Liu and Y. Peng, Nitrification–denitrification via nitrite in SBR using real-time control strategy when treating domestic wastewater, Biochem. Eng. J., 2007, 36, 87–92 CrossRef CAS.
- G. Ruiz, D. Jeison, O. Rubilar, G. Ciudad and R. Chamy, Nitrification–denitrification via nitrite accumulation for nitrogen removal from wastewaters, Bioresour. Technol., 2006, 97, 330–335 CrossRef CAS.
- S. Mehrabi, D. Houweling and M. Dagnew, Establishing Mainstream Nitrite Shunt Process in Membrane Aerated Biofilm Reactors: Impact of Organic carbon and biofilm scouring intensity, J. Water Process Eng., 2020, 37, 101460, DOI:10.1016/j.jwpe.2020.101460.
- J. Gu, Q. Yang and Y. Liu, A novel strategy towards sustainable and stable nitritation-denitritation in an A-B process for mainstream municipal wastewater treatment, Chemosphere, 2018, 193, 921–927 CrossRef CAS.
- T. K. Mohan, Y. V. Nancharaiah, V. P. Venugopalan and P. S. Sai, Effect of C/N ratio on denitrification of high-strength nitrate wastewater in anoxic granular sludge sequencing batch reactors, Ecol. Eng., 2016, 91, 441–448 CrossRef.
- S. Ge, Y. Peng, S. Wang, C. Lu, X. Cao and Y. Zhu, Nitrite accumulation under constant temperature in anoxic denitrification process: The effects of carbon sources and COD/NO3-N, Bioresour. Technol., 2012, 114, 137–143 CrossRef CAS.
- Y.-M. Li, J. Li, G.-H. Zheng, J.-F. Luan, Q. S. Fu and G.-W. Gu, Effects of the COD/NO3-N ratio and pH on the accumulation of denitrification intermediates with available pyridine as a sole electron donor and carbon source, Environ. Technol., 2008, 29, 1297–1306 CrossRef CAS.
-
T. Theis and A. Hicks, Methanol use in wastewater denitrification, Exponent, Alexandria, USA, 2012 Search PubMed.
- M. P. Ginige, J. C. Bowyer, L. Foley, J. Keller and Z. Yuan, A comparative study of methanol as a supplementary carbon source for enhancing denitrification in primary and secondary anoxic zones, Biodegradation, 2009, 20, 221 CrossRef CAS.
-
M. Beutler, K. H. Wiltshire, B. Meyer, C. Moldaenke, C. Luring, M. Meyerhofer and U. P. Hansen, APHA (2005), Standard Methods for the Examination of Water and Wastewater, Washington DC: American Public Health Association. Ahmad, SR, and DM Reynolds (1999), Monitoring of water quality using fluorescence technique: Prospect of on-line process control, Water Research, 33 (9), 2069–2074. Arar, EJ and GB Collins (1997), In vitro determination of chlorophyll a and pheophytin a in, Dissolved Oxygen Dynamics and Modeling-A Case Study in A Subtropical Shallow Lake, 2014, 217, 95.
- A. Rahman, R. Riffat, S. Okogi, I. Takacs, A. Al-Omari and S. Murthy, Evaluation of anoxic heterotrophic Yield using multiple calculation methods, Int. J. Environ. Res., 2016, 10, 255–264 CAS.
-
M. & Eddy, M. Abu-Orf, G. Bowden, F. L. Burton, W. Pfrang, H. D. Stensel, G. Tchobanoglous, R. Tsuchihashi and AECOM (Firm), Wastewater engineering: treatment and resource recovery, McGraw Hill Education, 2014 Search PubMed.
- H. D. Stensel, R. C. Loehr and A. W. Lawrence, Biological kinetics of suspended-growth denitrification, Journal, 1973, 45, 249–261 CAS.
-
V. Torres Ortiz, MSc, The George Washington University, 2013 Search PubMed.
- J. C. Akunna, C. Bizeau and R. Moletta, Denitrification in anaerobic digesters: Possibilities and influence of wastewater COD/N-NOX ratio, Environ. Technol., 1992, 13, 825–836 CrossRef CAS.
- V. Rocher, A. M. Laverman, J. Gasperi, S. Azimi, S. Guérin, S. Mottelet, T. Villières and A. Pauss, Nitrite accumulation during denitrification depends on the carbon quality and quantity in wastewater treatment with biofilters, Environ. Sci. Pollut. Res., 2015, 22, 10179–10188 CrossRef CAS.
- J. Kappeler and W. Gujer, Estimation of kinetic parameters of heterotrophic biomass under aerobic conditions and characterization of wastewater for activated sludge modelling, Water Sci. Technol., 1992, 25, 125–139 CrossRef CAS.
- M. Drolka, I. Plazl and T. Koloini, The Results of Mathematical Model and Pilot Plant Research of Wastewater Treatment, Chem. Biochem. Eng. Q., 2001, 4 Search PubMed.
- S. S. Adav, D.-J. Lee and J. Y. Lai, Enhanced biological denitrification of high concentration of nitrite with supplementary carbon source, Appl. Microbiol. Biotechnol., 2010, 85, 773–778 CrossRef CAS.
- J. Chung and W. Bae, Nitrite reduction by a mixed cultureunder conditions relevant to shortcut biologicalnitrogen removal, Biodegradation, 2002, 13, 163–170 CrossRef CAS.
- J. C. Akunna, C. Bizeau and R. Moletta, Nitrate and nitrite reductions with anaerobic sludge using various carbon sources: glucose, glycerol, acetic acid, lactic acid and methanol, Water Res., 1993, 27, 1303–1312 CrossRef.
- M. Beccari, R. Passino, R. Ramadori and V. Tandoi, Kinetics of dissimilatory nitrate and nitrite reduction in suspended growth culture, J. – Water Pollut. Control Fed., 1983, 55, 58–64 CAS.
- H. Poutiainen, S. Laitinen, S. Pradhan, M. Pessi and H. Heinonen-Tanski, Nitrogen reduction in wastewater treatment using different anox-circulation flow rates and ethanol as a carbon source, Environ. Technol., 2010, 31, 617–623 CrossRef CAS.
- H. Guven, M. S. Akca, E. Iren, F. Keles, I. Ozturk and M. Altinbas, Co-digestion performance of organic fraction of municipal solid waste with leachate: Preliminary studies, Waste Manage., 2018, 71, 775–784 CrossRef CAS.
- P. Sobieszuk and K. W. Szewczyk, Estimation of (C/N) ratio for microbial denitrification, Environ. Technol., 2006, 27, 103–108 CrossRef CAS.
- K. Bernat, D. Kulikowska and K. Żuchniewski, Glycerine as a carbon source in nitrite removal and sludge production, Chem. Eng. J., 2015, 267, 324–331 CrossRef CAS.
- M. Kornaros, C. Zafiri and G. Lyberatos, kinetics of denitrification by pseudomonas denitrificans under Growth conditions limited by carbon and/or nitrate or nitrite, Water Environ. Res., 1996, 68, 934–945 CrossRef CAS.
- Y. Mokhayeri, R. Riffat, I. Takacs, P. Dold, C. Bott, J. Hinojosa, W. Bailey and S. Murthy, Characterizing denitrification kinetics at cold temperature using various carbon sources in lab-scale sequencing batch reactors, Water Sci. Technol., 2008, 58, 233–238 CrossRef CAS.
- P. Dold, I. Takács, Y. Mokhayeri, A. Nichols, J. Hinojosa, R. Riffat, C. Bott, W. Bailey and S. Murthy, Denitrification with carbon addition--kinetic considerations, Water Environ. Res., 2008, 80, 417–427 CrossRef CAS.
-
A. Nichols, J. Hinojosa, R. Riffat and S. Murthy, Maximum methaol-utilizer growth rate: Impact of temperature on denitrification, WEFTEC, 2007, 15, 3511–3525.
-
C. de Barbadillo, J. Barnard, S. Tarallo and M. Steichen, Got carbon? Widespread biological nutrient removal is increasing the demand for supplemental sources, WE&T, www.wef.org/magazine Search PubMed.
- J. Dosta, A. Galí, T. Benabdallah El-Hadj, S. Macé and J. Mata-Álvarez, Operation and model description of a sequencing batch reactor treating reject water for biological nitrogen removal via nitrite, Bioresour. Technol., 2007, 98, 2065–2075 CrossRef CAS.
- J.-J. Her and J.-S. Huang, Denitrifying kinetics involving the distributed ratio of reductases, J. Chem. Technol. Biotechnol., 1995, 62, 261–267 CrossRef CAS.
- A. Muller, M. C. Wentzel, R. E. Loewenthal and G. A. Ekama, Heterotroph anoxic yield in anoxic aerobic activated sludge systems treating municipal wastewater, Water Res., 2003, 37, 2435–2441 CrossRef CAS.
- E. M. van den Berg, U. van Dongen, B. Abbas and M. C. van Loosdrecht, Enrichment of DNRA bacteria in a continuous culture, ISME J., 2015, 9, 2153–2161 CrossRef CAS.
- B. Kraft, H. E. Tegetmeyer, R. Sharma, M. G. Klotz, T. G. Ferdelman, R. L. Hettich, J. S. Geelhoed and M. Strous, The environmental controls that govern the end product of bacterial nitrate respiration, Science, 2014, 345, 676–679 CrossRef CAS.
-
M. Baideme, L. Plante, M. Butkus and K. Chandran, Impact of kinetic-limitation on the partitioning between glycerol-driven dissimilatory nitrate reduction to ammonium and denitrification, WEFTEC, 2019, 2369–2371.
- J. Friedl, D. De Rosa, D. W. Rowlings, P. R. Grace, C. Müller and C. Scheer, Dissimilatory nitrate reduction to ammonium (DNRA), not denitrification dominates nitrate reduction in subtropical pasture soils upon rewetting, Soil Biol. Biochem., 2018, 125, 340–349 CrossRef CAS.
- D. Nizzoli, E. Carraro, V. Nigro and P. Viaroli, Effect of organic enrichment and thermal regime on denitrification and dissimilatory nitrate reduction to ammonium (DNRA) in hypolimnetic sediments of two lowland lakes, Water Res., 2010, 44, 2715–2724 CrossRef CAS.
- W.-W. Lu, H.-L. Zhang and W.-M. Shi, Dissimilatory nitrate reduction to ammonium in an anaerobic agricultural soil as affected by glucose and free sulfide, Eur. J. Soil Biol., 2013, 58, 98–104 CrossRef CAS.
-
W. Tian, MSc, The biological metabolism of nitrate and nitrite in Pseudomonas fluorescens K27 amended with tellurium, Thesis Presented to The Faculty of the Department of Chemistry Sam Houston State University, p. 82 Search PubMed.
-
A. Badia, P. Yuehe and M. Dagnew, Effect of low COD/N ratio on denitrification from nitrite and nitrate: the case for complete versus partial nitrite denitrification, Under preparation, 2020 Search PubMed.
-
M. Eleanor Key, Economic analysis for nitrogen and phosphorus removal in Washington, Department of ecology state of washington, 2018, 1–50 Search PubMed.
Footnote |
† Electronic supplementary information (ESI) available. See DOI: 10.1039/d0ew00604a |
|
This journal is © The Royal Society of Chemistry 2021 |
Click here to see how this site uses Cookies. View our privacy policy here.