Denitrifying biofilm processes for wastewater treatment: developments and perspectives
Received
18th June 2020
, Accepted 5th October 2020
First published on 6th October 2020
Abstract
Biofilms can retain microorganisms with very different growth kinetics and different electron acceptor preferences, due to their natural redox zonation. Denitrifying biofilm processes are crucial biological processes for the treatment of nitrogen-polluted water. Heterotrophic, autotrophic, and methane-supported denitrifying biofilms have been widely utilized for removing nitrogenous contaminants in recent years. Heterotrophic denitrification is a conventional approach to conduct a respiratory process with nitrate or nitrite as the terminal electron acceptor and organic carbon serving as the main electron donor. Autotrophic denitrification is a cost-effective process for nitrogen removal with reduced inorganic compounds acting as the electron donor, such as hydrogen and sulfur. Methane could also be used directly as an electron donor to drive denitrification in the methane-based denitrification process. While the controlled biofilm-based systems are capable of enhancing the nitrogen removal efficiency by these denitrifiers, this review aimed to provide a comprehensive and up-to-date summary of these important denitrifying biofilm processes in attached-growth reactors from the perspectives of microbiology, reactor configurations, controlling factors, nitrous oxide emissions, and coexistence with other microorganisms. The technical challenges and associated strategies towards improving the performance of each denitrifying biofilm process are then discussed. The outlook for the larger engineering scalability of these processes is also put forward to facilitate their applications for higher treatment capacities.
Water impact
While the controlled biofilm-based systems are capable of enhancing the nitrogen removal efficiency by different denitrifiers, this review provides a comprehensive and up-to-date summary of several important denitrifying biofilm processes from the perspectives of microbiology, reactor configurations, controlling factors, nitrous oxide emissions, and coexistence with other microorganisms in order to facilitate their applications for high wastewater treatment capacities.
|
1 Introduction
The increase of nitrate (NO3−) concentration in waters during the past 40 years has become an environmental problem of international interest.1 Although NO3− itself is not a harmful compound, it is the direct precursor of nitrite (NO2−) which has been linked to several diseases such as methemoglobinemia.2 Additionally, NO3− loading to surface water is commonly deemed as a crucial contributor to water quality deterioration and eutrophication.3,4 Given that the maximum contaminant level for NO3− stipulated by the World Health Organization is below 11.29 mg N per L in drinking water,5 removing nitrogenous pollutants (NOx−) from wastewater via cost-effective ways is desirable.
The biological denitrification process is an economical and environmentally-friendly strategy to remove NO3− or NO2− from wastewater.6,7 The complete denitrification process consists of NO3− reduction to nitrogen gas (N2), with NO2−, nitric oxide (NO), and nitrous oxide (N2O) as the inevitable intermediates.8 Four enzymes are generally involved in this reduction as follows: nitrate reductase (NAR), nitrite reductase (NIR), nitric oxide reductase (NOR), and nitrous oxide reductase (NOS).9 Depending on the type of electron donor for nitrate respiration, the biological denitrification process is generally classified as heterotrophic denitrification, autotrophic denitrification and methane-driven denitrification.10
Biofilms mainly consist of diverse microbial consortia and their secreted extracellular polymeric substances. The formation and growth of biofilms is more complex than suspended sludge, including attachment, maturing and ageing. To harness the extraordinary synthetic and degradation abilities of bacteria in environmental biotechnology, efficient and stable microbial communities should be engineered. Because multiple biochemical conversions are expected in environmental technologies, the aim is to select and manage the best microbial community rather than the best individual strains. Thus, controlled biofilm-based systems are suitable to harness different microbial prospects for nitrogen removal. Attached-growth systems could generally immobilize more denitrifying organisms than suspended sludge, which indirectly prolongs the solid retention time; biofilm systems have been therefore widely adopted to enhance nitrogen removal via different denitrifying biofilm processes.11 Aside from the general operational conditions including pH, temperature, etc., the factors controlling the biofilm structure such as the mixing regime and biofilm thickness can also lead to variations of NO2−/NO3− reduction rates and enzyme activities.
In heterotrophic denitrification, organic carbon compounds such as glucose can be utilized by denitrifiers as biosynthetic sources and electron donors.12 Although inorganic carbon can also act as a carbon source for heterotrophs, nitrogen removal with this sort of carbon is limited. Heterotrophic denitrification has been the most popular process to remove nitrogenous pollutants from wastewater for decades owing to its high efficiency and the simplicity of the reactors required.4 Multiple types of biofilm reactors have been employed to perform heterotrophic denitrification. In an attempt to better understand the development of this nitrogen removal process, the knowledge on heterotrophic denitrifying biofilm systems from the aspects of microbiology, biofilm reactor configuration, affecting factors, N2O emissions and existed barriers for practical application should be synthesized. However, secondary pollution might occur due to the unused organic in the effluent.13 Therefore, to prevent the contamination caused by organic residues, a mixotrophic system combining heterotrophic and autotrophic denitrification has been successfully established when treating wastewater with a poor organic carbon source.14
The organisms involved in autotrophic denitrification use hydrogen (H2), sulfur, iron, pyrite or arsenite as an energy source and carbon dioxide (CO2) or bicarbonate (HCO3−) as a carbon source.15,16 H2-Based and sulfur-driven autotrophic denitrification are the most widely applied processes to remove NOx from wastewater with low carbon to nitrogen (C/N) ratios.17,18 Studies regarding other autotrophic denitrification processes with iron, pyrite or arsenite as an electrode donor in biofilm systems are relatively limited. Unlike heterotrophic denitrification, there are no concerns regarding additional dosage of an exogenous carbon source in the autotrophic denitrifying process.19 The N2O emissions via autotrophs were also reported to be much less than those via heterotrophs.20,21 To further popularize autotrophic denitrification for treating wastewater rich in NOx but poor in organic matter, a critical synthesis of H2-based and sulfur-dependent autotrophic denitrifying processes in biofilm systems is indeed essential.
The discovery of the denitrifying anaerobic methane oxidation (DAMO) process showed the possibility to directly use methane (CH4) as a carbon source for denitrification in the absence of oxygen (O2).22 This finding provides a new option for nitrate removal from wastewater. Given that CH4 is considered to be widely-applicable since that it can be generated onsite by the anaerobic digestion of waste activated sludge,23 DAMO is therefore a potential nitrogen-control process to be applied on an industrial-scale.24 To further improve the robustness of a CH4-driven denitrifying biofilm system, a synergistic process consisting of DAMO and anaerobic ammonium oxidation (anammox) has been successfully established.25,26 Therefore, great potential regrading environmentally-friendly nitrogen removal lies in a CH4-based denitrification process.
The denitrifying process in biofilm reactors has aroused significant attention in recent years. Several researchers have tried to give an overview of the use of microbial biofilm reactors for conducting denitrification. Specifically, Di Capua et al.1 (2015) reviewed the most used biofilm reactor configurations for maintaining autotrophic denitrification from the perspectives of performances, costs, potential, and drawbacks. Martin et al.27 (2012) summarized the potential of membrane biofilm reactors (MBfRs) for treating nitrogen-laden wastewater by presenting the key aspects of MBfR behaviour, summarizing applications, and discussing design considerations. Abdelfattah et al. (2020)28 aimed to provide an overview of microbial biofilm reactors for their applications in high-strength wastewater. Guo et al.29 (2019) lately published a relatively comprehensive review regarding opportunities and challenges for DAMO. However, these published review papers only discussed one or two denitrifying processes in the biofilm reactors from certain perspectives. It is thus necessary to systematically evaluate and compare the feasibility of heterotrophic, autotrophic, and methane-based denitrification in biofilm systems for their further development. This review article will review the denitrification processes in biofilm reactors through: 1) summarizing the microbial cultures involved; 2) presenting the important aspects of various attached growth systems; 3) analysing the key controlling factors for achieving higher denitrification efficiency; 4) discussing N2O production from both heterotrophic and autotrophic denitrification processes; 5) exploring the co-culturing of anammox bacteria and DAMO organisms in CH4-based and sulfur-dependent denitrification; and 6) identifying future research needs and directions of each denitrifying biofilm process.
2 Organic carbon-driven heterotrophic denitrifying biofilm processes
Heterotrophic denitrification is the sequential reduction of NO3− to NO2−, NO, N2O, and finally N2 with organic carbon acting as the main electron donor.30 Biofilms have been reported to have a lower negative surface charge and are more hydrophilic than S-sludge flocs due to the difference in microbial compositions and extracellular polymeric substances.31 The underlying nitrogen removal mechanism in the biofilm is more complex than that in suspended sludge as a result of microbial stratification (see more in section 7.0). Compared to activated sludge, the heterotrophic denitrifying biofilm is a better biomass aggregate type for nitrogen removal because of its longer solid retention time.11 Heterotrophic denitrification has been applied extensively due to its high energy efficiency and the simplicity of the reactor required.4 However, independent of the high denitrifying rates and treatment capacity, the application of this biological process might be hindered by the problem of clogging induced by the high growth rates of bacteria and toxic residual organic carbon left in the effluent.15,19
2.1 Microorganisms
As the development of new molecular biology techniques including 16S rDNA-based methods makes the characterization and evaluation of the bacterial community in a biofilm system possible, a significantly diversified microbial community in the heterotrophic biofilm was observed. It is difficult to summarize the community composition of heterotrophic denitrifiers by existing molecular technology, since such a microbial consortium presents high taxonomic diversity.32 Generally, the heterotrophic biofilm is dominated by the Proteobacteria phylum,33 as the bacteria belonging to this phylum tend to survive in an anaerobic environment utilizing organic nutrients.34 Specifically, the genera pertaining to β-Proteobacteria (e.g. Thauera-like bacteria) or γ-Proteobacteria (e.g. Enterobacter-like organisms) are ubiquitously found to be dominant in the biofilm reactor dedicated to remove nitrogen heterotrophically.14,32,33Thauera has been widely reported in denitrifying systems, and most of the Thauera have the ability to decompose NO3− or NO2−.35Enterobacter exhibits the ability to simultaneously denitrify nitrogen contaminants and remove phosphorus in the presence of organic matter.32
With the change of operational conditions, the dominant denitrifying organisms in the biofilm reactor might be different. For instance, the denitrifying genera thriving in the membrane bioreactor (MBBR–MBR) operated under anoxic–aerobic conditions were completely different from the systems which were run under constant anaerobic conditions.36Ottowia was deemed to be the major heterotrophic denitrifier in the MBBR–MBR, which exhibited capability in denitrification.36,37 Abundant Thermomonas species living on the metabolites yielded by AOB and NOB were also detected in the MBBR–MBR, which was again not widely observed in other heterotrophic denitrifying systems.36,38 However, given that the species of heterotrophic denitrifiers are diverse and the interactions among dominant denitrifiers and operational conditions are complex, a comprehensive summary of the existing associated organisms is challenging. Therefore, the determination of the dominant microorganisms with the physiological function of heterotrophic denitrification has to be kept in the context.
2.2 Biofilm reactors
Multiple types of biofilm reactors with different configurations have been proposed and applied in practice to verify the denitrification efficiency of heterotrophs in the presence of organic carbon. Packed bed reactors (PBRs), fluidized bed biofilm reactors (FBRs), bio-electrochemical reactors (BERs), moving bed biofilm reactors (MBBRs) and rotating biological contactors (RBCs) are the representative reactors to remove nitrogen pollutants heterotrophically. Although other attached-growth systems such as trickling reactors (TRs) and MBfRs can also degrade NO2−/NO3−, these reactors are not designed specifically for nitrogen removal by heterotrophs anaerobically. Specifically, given that the total nitrogen removal performance in the TR is very poor, such a system is mostly adopted to treat high-strength wastewater or as a post-treatment step for nitrogen removal due to strict discharge limits.39 MBfRs are usually adopted for methane- or H2-based denitrification. Therefore, these two reactors will not be discussed in this section.
PBRs.
PBRs have been widely applied to treat wastewater heterotrophically, and are fully submerged fixed bed biofilm reactors with various materials acting as biomass carriers. Porous carrier materials such as granular activated carbon and biochar are good options as they can provide shelter and large attachment-specific surface areas for microbial growth, inducing shorter start-up time and higher nitrogen removal ability.11 In particular, biochar is a better option for biofilm support in the PBR owing to the diverse functional groups on its surface, which increases the electrostatic interaction between organic compounds and microbial activities (Fig. 1).40 Additionally, the addition of biochar could also enhance the expression of the functional gene of denitrifiers, resulting in higher nitrogen removal efficiency (Fig. 1). Although the heterotrophs thriving in the PBR could efficiently decompose nitrogen compounds into N2, the excessive organic carbon in the effluent could result in secondary pollution, requiring additional treatment processes. Therefore, the PBR was coupled with a system dedicated to autotrophic denitrification such as a BER to avoid excessive organic carbon dosage.14 More discussion will be presented in detail in this section.
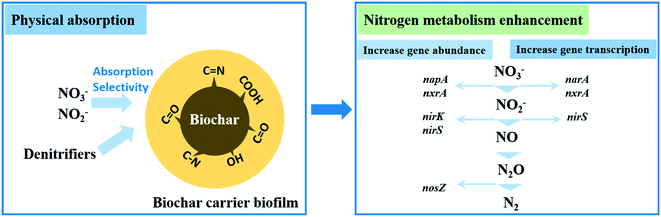 |
| Fig. 1 The nitrogen removal enhanced mechanism of denitrification for biochar carrier biofilms. The increased nitrogen removal gene abundance and expression of denitrifiers are denoted in italics. | |
FBRs.
The FBR is an attached growth system where the support is fluidized at high recirculation flow rates. Hollow fibers in the FBR usually operate in flow-through or dead-end mode. Compared to the PBR, the FBR exhibits a smaller pressure drop with higher rates, requires a smaller reactor volume, presents no bed clogging problems, and shows lower external mass transport resistance.41 To improve the performance of the FBR in heterotrophic denitrification, the support media in the reactor were usually alternated to enhance the biomass hold-up.42,43 Rubber was recommended as a good choice to enhance microbial attachment due to its lower density, suitable surface area and non-toxicity allowing for microbial growth on its surface. Therefore, rubber is easier to be fluidized than other classical media including microsand, charcoal, coconut coir and sepiolite.44 Rapid NO3− reduction rates by heterotrophs were then obtained in the FBR with rubber under low HRT conditions.42
BERs.
Prior studies have demonstrated the potential of bioelectrochemical systems in heterotrophic denitrification.4,45,46 For the BER with wastewater flowing through the anodic to cathodic side, the consumption of organic carbon in the BER is mainly by electricity generation and heterotrophic denitrification in the anode compartment.45 Notably, nitrogen pollutants can also be consumed in the cathode compartment when the BER contains a biocathode. The biocathode refers to a cathode immobilized by the biofilm, which is developed by feeding wastewater with nitrate and organic carbon in the cathodic side.46 Such a biofilm would be then selectively enriched with denitrifying microorganisms.4,46 Nitrogen pollutants can thereby be converted heterotrophically as cathodic electron acceptors, resulting in up to 100% NO3− removal efficiency.46
Given that the BER is designed based on the internal production of H2 performed by the electrolysis of water,33 bioelectrochemical systems are usually used to conduct hydrogenotrophic denitrification. However, although NO2−/NO3− could be efficiently reduced autotrophically, such reduction was achieved at the cost of high electric energy consumption. Additionally, when treating water with high nitrate but a low organic carbon source, using an autotrophic denitrification process alone might not fully remove the organic contaminants. Hence, coupling heterotrophic and autotrophic denitrification in one bioelectrochemical system was proposed to address that issue. Novel reactors such as the heterotrophic/biofilm-electrode autotrophic denitrification reactor (HAD-BER) and electro-autotrophic denitrifying packed bed reactor (HEAD-BER) have been developed for building a mixotrophic system composed of heterotrophic and autotrophic denitrifiers.14,47
The HAD-BER consisted of a cylinder, anode and cathode module (Fig. 2B).14 One of the key differences between the conventional BER and HAD-BER was that the anode in the latter reactor was a stainless steel rod, which was surrounded by the cathode module made of a perforated plexiglass column.48 Such design could avoid the generation of CO2via anodization of carbon rods, ensuring that most of the carbon sources were consumed by heterotrophic denitrifiers.48 The fiber thread was tied on the cathode and acted as a carrier for biofilm development. Both autotrophic denitrifying bacteria and heterotrophic denitrifying bacteria were found in the biofilm, where the CO2 produced by heterotrophs was used as an inorganic carbon source by autotrophs.48 The HEAD-PBR (Fig. 2A) was composed of two segments: a subjacent electrolysis segment (ES) and a superjacent denitrification segment (DS).14 H2 was firstly produced by electrolysis in the ES and then transported to the DS with the flow of influent to assist NO3− denitrification. Such a configuration allowed the electrochemical reaction to be separated from the denitrification process, eliminating the inhibition of high electric current on microbial activity, leading to high denitrification efficiency at minimum cost.14 The O2 sourced from water electrolysis (H2O → O2 + H2) can be consumed by the heterotrophs in the PBR by aerobic respiration and endogenous respiration, generating CO2 for the metabolism of H2-based autotrophic denitrifiers (eqn (1)–(4)).14 The CH2O found in eqn (1)–(4) refers to the generic organic carbon. Therefore, the overall anaerobic conditions required by heterotrophic denitrification were not seriously disturbed due to the synergistic interaction between autotrophs and heterotrophs.48 To further improve the removal of nitrogen contaminants, employing an ion exchange membrane in the BER might be a feasible strategy. This proposal has been verified by He et al. (2015)49 who reported high heterotrophic denitrification efficiency in a novel reactor integrating a membrane reactor (MBR) and BER (Fig. 2C).49 However, the membrane has not yet been applied in the HAD-BER or HEAD-BER to reveal its effect on nitrogen removal.
|  | (1) |
|  | (2) |
|  | (3) |
|  | (4) |
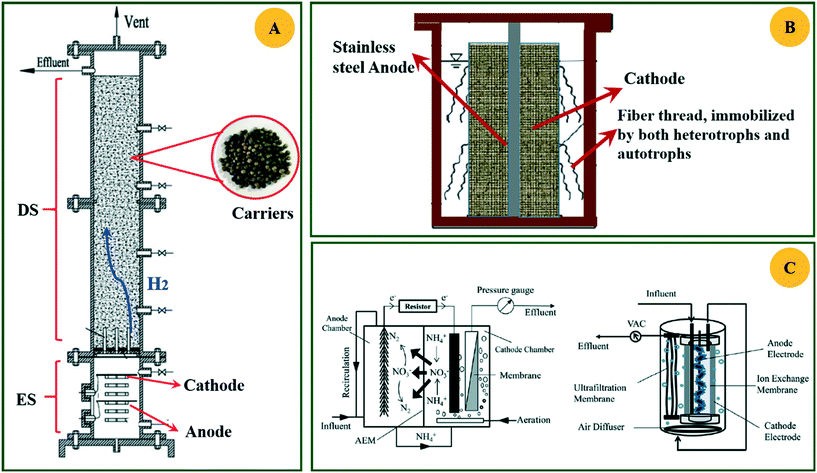 |
| Fig. 2 Schematic of the HEAD-BER (A), HAD-BER (B), and MBER (C). HAD-BER: heterotrophic/biofilm-electrode autotrophic denitrification reactor; HEAD-BER: electro-autotrophic denitrifying packed bed reactor; MBER: membrane bioelectrochemical reactor (MBER); AEM: anion exchange membrane. Reproduced from ref. 14 and 49 with permissions from [Elsevier] and [Royal Society of Chemistry], copyright 2020. | |
RBCs.
The RBC is a widely-used biofilm-based system designed for nitrogen removal and organic matter stabilization.50 This reactor is typically composed of a series of closely spaced discs that are mounted on a horizontal shaft and are partially immersed in a tank through which wastewater flows.51 With the continuous rotation of the shaft, a biofilm is immobilized on the discs and can be constantly renewed.52 Generally, the RBC is designed to achieve simultaneous nitrification and denitrification by developing a mixed microbial biofilm containing both nitrifiers and denitrifiers. This is because such a system is partially exposed to air regularly, leading to the change of O2 concentration in the biofilm exterior. Therefore, the metabolic pathways for nitrogen in the RBC are different to other fixed biofilm systems. A sufficient carbon source for NO3−/NO2− degradation is the basis to achieve complete heterotrophic denitrification in the RBC. Therefore, the total nitrogen removal in the conventional RBC was quite low when treating wastewater with a high degree of nitrogen pollutants but a low carbon source. The transformation of the RBC into a bioelectrochemical reactor (termed REBC) has been proposed to treat nitrogen-rich wastewater by creating a heterotrophic–autotrophic denitrification process.53
MBBRs.
The MBBR is a simple and efficient technology to treat wastewater heterotrophically, with biomass attached on the suspended plastic carriers.54 In comparison with the activated sludge system and fixed-bed reactor, this technology has several advantages for greater treatment capacity. Specifically, the biomass residence time in the MBBR is expected to be higher than that in a suspended growth system.55 Compared to a fixed-bed biofilm reactor like the PBR, a better oxygen transfer, higher denitrification rate, and larger surface area for mass transfer are achieved in the MBBR due to higher contact efficiency between organisms and substrates.36 For the record, the MBBR was combined with an MBR to increase the biomass attachment for the purpose of improving the denitrification efficiency.36 The oily bilge water containing high COD was better treated in this hybrid biofilm system than the MBBR alone.56 However, the membrane fouling issues in the hybrid reactor require additional solution.56 For widespread application of this integrated system, seeking fouling preventive-control strategies has become a hot topic.57 Decreasing the sludge retention time in the MBR has been proposed and deemed as an effective way to avoid this issue.57
Conclusively, the integrated system consisting of different traditional biofilm reactors exhibited promising prospects for controlling NOx− pollutants via heterotrophs. Given that the heterotrophic denitrifying bacterial activity could be enhanced by electro-stimulation, combining the BER with the PBR together with a membrane configuration is a feasible strategy to further improve the treatment capacities of conventional heterotrophic denitrifying systems in an energy-efficient way. Considerable efforts are though needed for the scale-up of various integrated reactors.
2.3 Key affecting factors
Heterotrophic denitrifying bacteria are sensitive to the change of C/N ratios. Similarly, the growth of heterotrophs in the biofilm could be accelerated by high C/N ratios, as the microbial yield of heterotrophic denitrifiers is closely tied with NOx− and COD availability (eqn (5)).4 High C/N ratios therefore promote higher total denitrification rates. Notably, the accumulation of nitrite also heavily depends on the organic source availability, with low C/N ratios being beneficial for nitrite formation.13,33 The influent C/N ratio of 1.5 was related to a satisfactory denitrification performance with almost no NO2− accumulation.33 For the influent with a poor organic carbon source, maintaining high autotrophic and heterotrophic bacterial activities is essential for high total nitrogen removal. Considering the slow growth rates of autotrophs, a longer hydraulic retention time (HRT) is required to fully decompose the nitrogen contaminants. Although extending the HRT could facilitate the nitrogen removal and lead to lower nitrite accumulation, an extremely long HRT under low C/N conditions may induce the endogenous respiration phase of heterotrophic bacteria (i.e. a phase when organisms live on the nutrition secreted by themselves).33,55 Thereby, a lower biomass would be attained. | 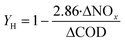 | (5) |
The nitrite build-up owing to low C/N will result in pH variation, which affects the total nitrogen removal efficiency dramatically. Generally, the optimum pH value for denitrification ranges from 7 to 8, since a neutral pH environment is suitable for most environmental microorganisms.58 Therefore, the removal rates of NO3− or NO2− tend to be lower when the pH is out of this range.59 Additionally, alkaline or acidic conditions could also negatively affect the activities of functional enzymes such as nitrite reductase, increasing N2O emissions and reducing the denitrification efficiency consequently.58
Aside from C/N and pH, the type of carbon source is another important factor affecting the denitrification efficiencies, as this could impact the structure of denitrifying microbial communities in the biofilm systems strongly.60 The microbial communities involved in the denitrification process with different carbon sources may be fundamentally distinct and exhibit different biodiversities and functionalities.60 For instance, the metabolic reaction of single-carbon compounds such as methanol is different to other organic carbon resources such as ethanol.61 Methanol is the exclusive carbon source of methylotrophs due to their unique key enzyme (methanol dehydrogenase) that catalyzes the oxidation of methanol to formaldehyde.60 Several methylotroph microorganisms including Hyphomicrobium spp., Paracoccus denitrificans, and Nitratireductor aquibiodomus have been characterized and deemed as dominant organisms in the methanol-fed denitrifying biofilm reactor.61 For the biofilm reactor fed with ethanol, a higher biomass yield was obtained than the system with acetate or methanol despite similar denitrification efficiencies.60,62 This might be due to the differences in dominant denitrifiers in the ethanol- and methanol-dosed reactors.60
Other specific factors for biofilm development, including the mixing regime, biofilm thickness and substrate concentrations in the bulk liquid, can also influence the heterotrophic denitrification performance in attached-growth systems. Two mixing regimes, namely completely mixed or plug flow, are widely applied in denitrifying biofilm systems. The choice of mixing regime may impact the homogeneity of nutrient concentration in the bulk liquid, affect mass transfer efficiencies by influencing the biofilm surface, and change the degradation rates of pollutants consequently.63 For instance, plug flow is typically operated in upflow PBRs, where the substrate concentrations at the influent end are higher than those at the effluent end. Such nutrient gradients can cause nutrient deficiency for the biofilm located at the top of the reactor, adversely affecting the heterotrophic denitrification performance in the reactor accordingly.64 Choosing complete mixing may solve the issue regarding nutrient gradients to some extent, as this mixing regime causes fewer dead ends in the reactor than plug flow.65 Therefore, for biofilm reactors such as the MBBR and sequent batch biofilm reactor, complete mixing is usually adopted to agitate the liquid. Seeking a suitable agitation rate is important for complete mixing, since a suitable agitation rate can 1) ensure good mixing to improve mass transfer, 2) improve the solubility of particulate organic matter, and 3) avoid the unnecessary abrasion of the biofilm.66 Agitation rates ranging from 200 to 500 rpm were reported to be efficient for achieving a high COD removal rate in the SBBR when treating a dairy effluent.66
In terms of biofilm thickness, the optimal thickness for denitrification performance depends on the specific operational conditions and reactor type. Biofilm thickness ranging from 600 to 1200 μm was reported to be optimal for the MBR used to conduct simultaneous nitrification and denitrification (SND) under limited O2 conditions.67 A thinner biofilm (<200 μm) could not provide enough space for anoxic denitrifier proliferation.67 A thicker biofilm (>2000 μm) ensured a thick anaerobic region but cannot achieve satisfying nitrogen removal efficiency. This is because the thick biofilm acted as a diffusive barrier and decreased the nutrient availability for nitrifiers, deteriorating the SND thereafter.67 As for the denitrifying fluidized bed bioreactor (DFBBR), higher denitrification rates were obtained in the thicker biofilm (680 μm) at high C/N compared to that in the thinner biofilm (230 μm) under low C/N.68 This was probably because the biofilm thickness and carbon availability collectively affected the substrate diffusion pattern,67 inducing the change of enzymatic activities through the depth of the biofilm.69 However, given the difficulty in characterizing the microbial composition and substrate concentration in various non-uniform biofilms, it is hard to clearly understand the interaction among various denitrifying intermediates.70 Although researchers have developed the modelling to simulate by-product profiles in denitrifying biofilms with various thicknesses, more research is still warranted.70
Bulk substrate concentrations could regulate the interactions among denitrifying intermediates in the biofilm. When the anaerobic systems were supplied with excess carbon and NO3−, high NO3− reduction rates were obtained in the exterior biofilm, and the consumption of NO2−, NO, and N2O mainly happened in the interior of the biofilm.68 When denitrifying biofilms were supplied with a limited amount of electron donors, N2O was exported to the bulk liquid along with higher NO2− formation. Such phenomena indicated that the outer portion of the biofilm, which was the main space for NO3− reduction, became smaller.68 Therefore, the behaviour of denitrifiers in the biofilm can be affected by the change of bulk substrate concentration to some extent.
Overall, the C/N ratio is the key factor for controlling the performance of the complete heterotrophic denitrification process in biofilm reactors. Seeking the appropriate C/N ratio is important, since the pH could be variated due to insufficient supply of electron donors and the unbalanced metabolic reactions of denitrification, inducing lower treatment capacity consequently. Finding a suitable mixing regime is important for keeping the biofilm intact while ensuring high mass transfer to maintain good heterotrophic performance. Altering the biofilm thickness could influence the behaviour of denitrifiers in the biofilm, affecting the nitrogen removal efficiency thereafter. More research is needed to study the complex interaction among intermediates in biofilms with various thicknesses.
2.4 N2O production
Denitrification is the consecutive reduction of NO3− and NO2− to NO, N2O and N2, with four enzymes involved: NAR, NIR, NOR, and NOS. N2O is therefore an obligate intermediate in the heterotrophic denitrifying biofilm process. The formation of N2O during the wastewater denitrification process is often due to the inactivation of NOS, which could be incurred by the choice of carbon source type and associated concentration.
Insufficient carbon resources induce high NO2− accumulation, causing pH variation and direct impairment of NOS, finally leading to the increase of N2O emissions.71 For the reactor containing fixed biofilms, higher N2O emissions in the attached system were considered to be accompanied with a thinner biofilm thickness induced by low C/N.68 Besides the inactivation of NOS, another possible explanation for this phenomenon is the decrease in carbon availability with the depth of the biofilm.70,72 The higher degree of N2O usually found in the inner section of the biofilm is then diffused to the exterior of the biofilm and consumed by denitrifiers (Fig. 3).72 A lower biofilm thickness cannot ensure enough space for denitrifier proliferation, deteriorating the denitrification process thereafter. Molecular technology further confirmed the N2O pattern in the biofilm, wherein more norB genes were transcribed than nosZ genes in the inner biofilm from the BER, indicating that N2O was mainly produced in the inner section rather than the surface of the biofilm.69 Another aspect affecting N2O production from heterogenous biofilms is the degradability of organic substrates.71 Simple carbons such as acetate, ethanol, and glucose were deemed to incur high efficiency of nitrate reduction, as they were more easily used and would supply an adequate amount of electrons for heterotrophic denitrification, inducing less N2O production thereafter.59 However, slowly degradable organics are usually found in typical wastewaters,30 which can partially explain the high N2O emissions while treating those wastewaters.
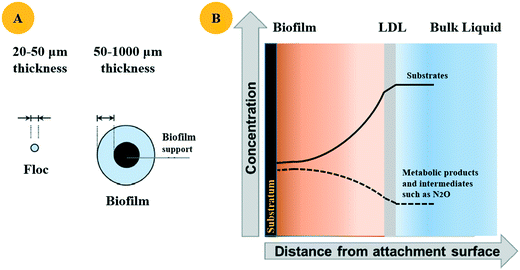 |
| Fig. 3 A shows the idealized schematics of a floc and a biofilm. B presents the liquid diffusion layer (LDL), as well as the profiles of substrate and metabolic products such as N2O in a typical biofilm. | |
In summary, the unbalance between electron supply and consumption would lead to deteriorated denitrification in the heterotrophic biofilm system by inactivating NOS and thus increased N2O production. In an attempt to mitigate N2O emissions from wastewater with inadequate organic carbon, the coupling of heterotrophic and autotrophic denitrification processes is a feasible strategy towards that goal, as the N2O emission via autotrophs is generally much less than that via heterotrophs. More discussion regarding N2O production via autotrophs will be presented in section 3.4 and section 4.4.
2.5 Challenges and strategies for the application of heterotrophic denitrifying biofilms
Heterotrophic denitrifying biofilm systems have been widely used to treat nitrogen pollutants in water due to their features of simplicity and high efficiency. However, caution is required for exogenous organic carbon addition, as the effluent may be contaminated with unused organics or be polluted by accumulated nitrite, which is more toxic than nitrate.
The disadvantages of heterotrophic denitrification can be resolved by combining it with autotrophic denitrification. An elemental-sulfur (S0) based process was recommended as a good alternative due to S0 being non-toxic, water-insoluble, and stable under normal conditions.13 However, the problem of acidity caused by sulfate generation needs to be addressed by adding an external alkaline supplement.13 Coupling heterotrophic denitrification with H2-based denitrification is another way to avoid excess addition of organic carbon. The cooperative heterotrophic and autotrophic denitrification has been successfully achieved in the newly designed BER or PBR with a diversified configuration (see more in section 2.2). However, the application of mixotrophic systems is still at its infancy due to issues such as the high capital cost of the BER; thus more pilot-scale research is urgently required.
3 Hydrogen-driven chemoautotrophic denitrifying biofilm processes
Hydrogen-driven chemoautotrophic denitrification is a process where hydrogenotrophic bacteria reduce NO2− or NO3− to N2 using H2 as an energy source and CO2 or HCO3− as a carbon source (eqn (6)).73,74 Aside from the merits of lower cell yield than heterotrophic denitrification and the elimination of post treatment, the harmless feature of H2 to water is the main advantage of hydrogenotrophic denitrification. Unlike heterotrophic denitrification, no additional steps are required to remove the excess substrate or its derivatives in this denitrifying process.73,74 However, as some drawbacks still exist in this process, the scale-up of H2-driven denitrifying systems is still challenging. | 2.16NO3− + 7.24H2 + 0.8CO2 → 0.16C5H7O2N + N2 + 5.6H2O + 2.16OH− | (6) |
3.1 Microorganisms
The microbial composition of the biofilm is crucial for the performance of the reactors. With the development of molecular analysis technology, the metabolically active communities involved in hydrogenotrophic denitrification are targeted as shown in Table 1.
Table 1 The major bacteria tied with anaerobic H2-based denitrifying biofilm systems
Strain |
Function |
Reactor type |
Ref. |
HF-MBfR: hollow fiber-membrane biofilm reactor; PER: packed bed reactor; BER: biofilm electrode reactor.
|
Thiobacillus denitrificans; Azonexus hydrophilus; Hydrogenophaga pseudoflava; Hydrogenophaga electricum; Paracoccus versutus group |
Hydrogenotrophic denitrification |
HF-MBfR |
Park et al. (2016)17 |
Paracoccus denitrificans; Flavobacteria |
Hydrogenotrophic denitrification |
PER; BER |
Park et al. (2006);75 Szekeres et al. (2002)73 |
Pseudomonas sp.; Sphingobacteria |
Hydrogenotrophic denitrification |
MBfR |
Sahu et al. (2009)76 |
Marinobacter; Hydrocarbonoclasticus |
Hydrogenotrophic denitrification |
MBfR |
Van et al. (2010)77 |
Hydrogenophaga sp. |
Hydrogenotrophic denitrification |
Glass bead biofilm reactor |
Park et al. (2005)78 |
Chloroflexi
|
Backbone forming agent; heterotrophs |
MBfR |
Park et al. (2016)17 |
High levels of Proteobacteria were usually found in biofilm bioreactors with stabilized H2-dependent denitrifying processes.73 For instance, Paracoccus denitrificans and Pseudomonas sp. are widely-reported as the dominant genera affiliated to the α subclass of Proteobacteria.73–75 Bacterial strains from these genera are known to reduce NO3− with the oxidation of H2.76Flavobacteria and Sphingobacteria are the two bacterial classes ubiquitously existing in hydrogenotrophic denitrifying biofilms.75,76 In a H2-based membrane biofilm reactor (MBfR) for nitrate and perchlorate reduction, Marinobacter hydrocarbonoclasticus represented about half of all clones in the MBfR biofilm.77Hydrogenophaga sp. was the predominant species in a glass bead biofilm reactor using H2 as an electron donor for denitrification.78Thiobacillus denitrificans constituted up to 32% of all clones in a H2-based hollow fiber-membrane biofilm reactor (HF-MBfR).17 The inevitable co-existence of autotrophs and heterotrophs in hydrogenotrophic denitrifying systems seems to be conductive to the performance of biofilm attached systems.19 For example, microorganisms such as Chloroflexi can prevent biofouling of the MBfR by decaying the biomass into carbon resources for heterotrophic denitrifiers.17,27 Therefore, with the cooperative effect of autotrophs and heterotrophs, larger treatment capacity together with higher denitrification efficiency could be attained.19
Overall, extensively diverse hydrogenotrophic species have been detected in anaerobic H2-dependent biofilm reactors. High microbial diversity with the inevitable co-existence of autotrophs and heterotrophs can prevent some technique problems such as membrane fouling in the MBfR, ensuring higher NO3−/NO2− reducing efficiency in the biofilm reactors.
3.2 Biofilm reactors
Given that 1) the maintenance fee of the PBR is low resulting from its simple configuration, 2) the BER is a cost-effective system to produce in situ H2, and 3) the MBfR provides a safer solution for H2 delivery with the possibility for higher fluxes, these three biofilm bioreactors were widely selected as the reactors to study H2-based denitrification in attached-growth systems. Although other systems such as the FBR also have potential to carry out H2-based denitrification, studies regarding this issue are exiguous.1 Therefore, this section only presents the operation principle of the PBR, BER and MBfR together with their technical or monetary consideration.
PBRs.
PBRs, also known as fixed bed reactors, have been widely adopted to perform hydrogenotrophic denitrification using various materials as biofilm carriers, including sand, silicic gravel, glass, polyurethane and bio-ceramics (Fig. 4).1 The high specific area for organism colonization provided by these inexpensive natural materials is the main reason for the superior performance of the PBR in H2-based denitrification.79 Based on the operational mode, the influent in the PBR is provided in up-flow or down-flow mode relative to the gas direction. The H2 supplied for the PBR is usually implemented by delivering H2 in an external tank for gas absorption or sparging H2 gas directly.1 Several strategies have been adopted to increase H2 availability in liquid to enhance denitrification efficiency, including increasing the hydrogen-to-water flow rate ratio, extending the HRT and adopting gas permeable membranes to increase H2 solution.80,81 H2 can also be provided inside of the reactor by conducting water electrolysis and anoxic corrosion of metallic iron (see section 2.2).82,83 The latter way for H2 provision is able to render higher H2 availability for denitrifiers, allowing the PBR to operate under higher NO3− loading rates with a lower HRT.83 Given that hydrogenotrophic denitrifiers require CO2 when converting NOx− as a carbon resource, methanol electrolysis was then coupled with the PBR to simultaneously provide H2 and CO2 to those organisms.84 Additionally, the cost of H2 generation using methanol electrolysis is even much cheaper than water electrolysis.84
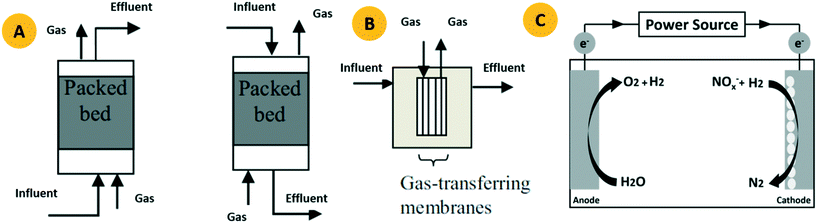 |
| Fig. 4 PBR run in either up-flow or down flow mode based on the influent direction (A). The MBfR operated in dead-end mode (B). A schematic of the BER used for H2-based denitrification (C). | |
Membrane biofilm reactors.
Unlike conventional fixed bed biofilms in which the highest microbial activity mostly happens at the outer biofilm edge, the occurrence of the highest microbial activity in the membrane biofilm reactor (MBfR) is within the biofilm hinging on the donor and acceptor concentrations.27 For instance, when the electron donor on the membrane side is limited and the electron acceptor level on the biofilm side is insufficient, the highest microbial activity usually occurs in the biofilm's middle section.85 The low solubility of H2 is a major obstacle for the application of the MBfR to carry out hydrogenotrophic denitrification. Based on the H2 transfer flux direction, two different MBfR configurations have been designed to optimize the H2 utilization efficiency by hydrogenotrophic denitrifiers as shown in Table 2. In comparison to a typical MBfR, the outside-in MBfR presented better H2 utilization efficiency as the hydrogenotrophic denitrifiers colonized on the membrane can consume H2 diffusing from the shell to the membrane surface immediately.76 The membrane is a key component of the MBfR, as it can largely determine the performance of the reactor. H2 could be fully used in the reactor containing a dead-end membrane, while the back-diffused N2 can dilute the H2 concentration and slow-down the denitrification rates thereafter. Adopting a flow-through membrane is an alternative to prevent the N2 back-diffusion. However, such a kind of reactor configuration consumes a higher amount of energy and gas.27
Table 2 Different membrane bed reactor designs for better denitrifying performance
Design |
H2 transfer flux |
Features |
Ref. |
Typical MBfR |
H2 is provided from the inside of the hollow fiber membrane fiber to the shell. Contaminated water is fed to the shell side of the reactor |
1. Membrane prompts H2 mass transfer rate, avoiding H2 loss |
Martin et al. (2010);27 Rittmann et al. (2004)86 |
2. High microbial population attached on the membrane |
3. Incur high gas utilization |
4. Achieve higher nitrogen removal |
Outside-in MBfR |
H2 is supplied to the shell while water is fed to the lumen of the reactor. |
Higher H2 utilization efficiency than that in the typical MBfR |
Sahu et al. (2009)76 |
BERs.
In order to minimize the cost of H2 provision for denitrifiers, the BER was invented.87 The H2-dependent denitrification process in the BER is based on the use of gaseous H2 by biofilm microbes.2 Specifically, water in the anodic side is firstly electrolysed to H2, which then fluxes to the denitrifying microorganisms immobilized on the surface of the cathode to assist hydrogenotrophic denitrification.75 The lower biomass obtained from hydrogenotrophic denitrification than that via heterotrophic denitrification may further improve the feasibility of the BER for removing nitrogen contaminants from nitrate-rich wastewater.2 To achieve the large-scale application of the bioreactor, the choice of the materials for electrode manufacture matters due to monetary consideration. Stainless steel and carbon rods are inexpensive but good selection for producing the cathode and anode in the BER, respectively. More specifically, stainless steel could improve the resistance against corrosion in the wastewater apart from the excellent conductivity. The anode made of carbon rods can serve as a carbon source for autotrophic denitrification bacteria through carbon oxidation.19 It should be noted that the conductivity of wastewater is further enhanced by dissolving CO2 into HCO3− and CO32− ions.2 To further improve the removal efficiency of pollutants in the BER, strategies must be proposed to curb the technique limitations such as 1) insufficient inorganic carbon supply for autotrophic denitrification, and 2) the irreversible microbial inactivation caused by high current.14 By far, BERs with multi-electrode systems88 or a new type of bio-carrier such as fiber threads48 have been suggested as an effective approach to achieve that goal. The achievement of a mixotrophic system by cultivating both autotrophs and heterotrophs is another promising method to prompt the treatment capacity of the BER (see more in section 2.2).
Altogether, to obtain better denitrifying performance of the bioreactors, the key is to seek strategies towards higher H2 utilization efficiency while maintaining satisfactory metabolic activities of associated organisms. Carrying out in situ water electrolysis for lower H2 loss together with a membrane for higher biomass attachment is the common approach to achieve that goal. Apart from the advancement in the reactor configuration, a comprehensive understanding of the factors controlling hydrogenotrophic denitrification is also essential for attaining better denitrifying performance.
3.3 Key affecting factors
Several crucial operational factors can regulate the effectiveness of hydrogenotrophic denitrification.3 The pH and H2 availability are the two most determinant factors controlling the denitrifying efficiency as presented below.
The pH variation in the H2-based denitrifying biofilm system is inevitable, as microbial hydrogenotrophic denitrification is always accompanied by the production of OH− ions (eqn (7) and (8)).10 Although the dissolution of CO2 into HCO3− or CO3− could buffer the pH variation to some extent, it is hard to completely prevent pH variation during hydrogenotrophic denitrification. The high dependency of NO3− consumption and NO2− reduction on pH have been well demonstrated previously, as pH impacts the functions of all denitrifying enzymes including nitrite and nitrate reductase.10 The associated reduction rates decrease when pH deviates from the optimal value for relative enzymes.3 Slightly acidic conditions (5.5–6.0) usually lead to segment denitrification, causing N2O emissions and NO2− accumulation.3,10 The mature biofilms seem to obtain higher resistance to the NO2− build-up induced by pH alternation. This is because the decaying cells or soluble microbial products from the attached systems may act as the organic carbon source for the coexisting heterotrophic denitrifiers in the reactor, preventing unwanted nitrite accumulation thereafter.89
| 5CH3COOH + 8NO3− → 10CO2 + 4N2 + 6H2O + 8OH− | (7) |
| 2NO3− + 5H2 → 4H2O + N2 + 2OH− | (8) |
The aqueous solubility of H
2 is another limiting factor for high hydrogenotrophic denitrifying efficiency. As described before, the optimization of reactor designs by using porous membranes, hollow fibers,
etc. has been widely adopted to enhance the biofilm hold-up and increase the H
2 utilization efficiency, inducing better denitrification kinetics than simple batch reactors. Combining the biological and electrochemical features together in the reactor such as the BER is a promising method to reduce the possibility of explosion due to H
2 loss. Such combination can also enhance NO
x reduction rates. The properties of the biofilm regarding thickness and density have been shown to affect heterotrophic denitrification by altering the diffusion coefficients of substrates for microbial metabolism.
8,90 Generally, thinner biofilm thickness improves gas transfer by alleviating the resistance to diffusion. However, thin biofilms may not possess enough mechanical strength to withstand the liquid shear stress.
27 The specific effects of biofilm properties on H
2-based denitrification is not clearly revealed yet, given that the biofilm thickness is not uniform in various reactors.
3.4 N2O production
The underlining mechanisms of N2O production and reduction during hydrogenotrophic denitrification have not been well characterized yet.3 The investigation of N2O accumulation during hydrogenotrophic denitrification in biofilm systems is lacking. Only Li et al. (2017)3 evaluated the characteristics of N2O build-up during H2-based denitrification via a series of batch tests under various environmental conditions using activated sludge. In that study, almost no N2O accumulations were observed at pH ranging from 7.0 to 9.0. However, immense N2O accumulation was achieved under slightly acidic conditions (pH = 6.0), indicating that N2O conversion suffered a more serious decline than nitrite reduction.3 Li et al. (2017)3 also found that using a small amount of nitrate rather than nitrite as the sole electron acceptor could induce higher N2O emissions, as N2O conversion could only be suppressed in the presence of NO2− with relatively high concentration.91 Low temperature (15 °C) was conductive to N2O accumulation during hydrogenotrophic denitrification,3 since temperature beyond 15 °C is the suitable range for N2O reductase. Due to the differences among biomass types, it is difficult to deduce the N2O flux pattern of denitrifying biofilm systems simply based on the knowledge of suspended sludge. More specifically, the unique microbial stratification, microbial interaction, substrate gradients, and substrate interactions within the biofilm imply the distinct N2O formation behaviour, which is completely different from that of suspended growth systems. Thus, intensive efforts are required to study the N2O sourced from H2-based denitrifying biofilm systems.
3.5 Challenges and strategies for the application of H2-based denitrifying biofilms
Due to the elimination of organic carbon requirement and the moderate cost of H2 manufacture, multiple hydrogenotrophic denitrifying biofilm reactors have been designed to treat wastewater with low C/N ratios, including the effluent from swine farms and nitrogen-dealing industries.1,17,92 The low H2 availability for autotrophic denitrifiers is the major problem for achieving high denitrification efficiency in biofilm reactors including the PBR and MBfR. Adopting an additional membrane is a common way to obtain superior effluent quality.93 Fouling in MBfRs is induced by excessive biomass accumulation and precipitation of minerals at the membrane surface, which will further impede the mass transfer rates.89 The regular physical and chemical cleaning of the membrane can avoid the fouling problem effectively; however, such an approach could lead to higher capital cost and discontinuous denitrification. Other methods including 1) increasing the transmembrane pressure, 2) choosing the membrane type like hollow fibers for cultivating a more diversified microbial community, and 3) limiting the provision of H2 flux to create starving conditions and inhibit extracellular polymeric (EPS) production are the feasible and cost-effective ways to avoid fouling.89,93,94
However, because of the low solubility of hydrogen and risk of explosion with escaped hydrogen gas, extending the application scale of hydrogenotrophic denitrification is still challenging. Most of the studies regarding H2-based denitrification in biofilm attached systems are still at the stage of laboratory development. More pilot-scale studies are needed.
4 Sulfur-driven chemoautotrophic denitrifying biofilm processes
The generation of sulfide through the biological sulfate-reducing process is a severe problem for wastewater treatment due to the corrosion and toxicity of sulphur compounds, including sulfide (S2−), thiosulfate (S2O32−) and elemental sulfur (S0). Sulfide-oxidizing chemoautotrophic denitrification (SOAD) was regarded as one of the most important mechanisms to control sulfide contamination while reducing N oxides.95Eqn (9)–(12) show the complete and partial biological oxidation of S2− to SO42− and S0, respectively, with NOx− as the electron acceptor.96 The complete oxidation of S0 to SO42− (eqn (9) and (10)) is more energetically attractive than the partial oxidation to S0 (eqn (11) and (12)) considering the variation of stranded Gibbs free energy.96 Moreover, the NOx− required by sulfide conversion to S0 is four times higher than that by complete oxidation of S2−. Therefore, the complete oxidation of S2− is usually observed under low S/N ratios.97 | 5HS− + 8NO3− + 3H+ → 5SO42− + 4N2 + 4H2O | (9) |
| 3HS− + 8NO2− + 5H+ → 3SO42− + 4N2 + 4H2O | (10) |
| 5HS− + 2NO3− + 7H+ → 5S0 + N2 + 6H2O | (11) |
| 3HS− + 2NO2− + 5H+ → 3S0 + N2 + 4H2O | (12) |
S2O32− is often observed in the industrial effluent as the product of sulfide oxidation. The drainage from the mining industry also contains thiosulfate as S2O32− is a less toxic substitute of cyanide for gold leaching.98 The anoxic S2O32− oxidation by chemoautotrophs with simultaneous nitrogen removal is shown in eqn (13)–(15).95,99 This anoxic process has been recently investigated in biofilm systems under different operational conditions (see more in section 4.2). | 3.10NO3− + S2O32− + 0.73H2O + 0.45HCO3− + 0.09NH4+ → 0.09C5H7O2N + 3.1NO2− + 1.64H+ + 2SO42− | (13) |
| 2.07NO2− + S2O32− + 0.45HCO3− + 0.08NH4+ → 0.09C5H7O2N + 1.03N2 + 0.31H2O + 2SO42− | (14) |
| 1.24NO3− + S2O32− + 0.11H2O + 0.45HCO3− + 0.09NH4+ → 0.09C5H7O2N + 0.62N2 + 0.4H+ + 2SO42− | (15) |
It is worth noticing that elemental sulphur (S0) can also serve as an electron donor for denitrifying NO3−, termed as S0-based denitrification (eqn (16)).100 Compared to other two sulfur components, S0 is much easier to handle and transport and can serve as both the energy source and biomass support.97 This denitrifying process is not only observed in the reactor with sulfur acting as the sole electron source, but also observed during the process of SOAD where S0 is an main intermediary product when reducing NO3− (Fig. 5).101 The biosynthesis of new cells via consuming S0 or S2O32− is expressed by the term C5H7O2N.102 Given that sulfur-based chemoautotrophic denitrification is usually applied when sulfur components and nitrate co-exist in wastewater such as the effluent from a refinery plant, the extra supplementation of an external electron donor is therefore not necessary. Additional benefits of this autotrophic denitrification are the low biomass yield, safe handling, and non-toxicity.100 | NO3− + 1.1S0 + 0.4CO2 + 0.76H2O + 0.08NH4+ → 0.5N2 + 1.1SO42− + 1.28H+ + 0.08C5H7O2N | (16) |
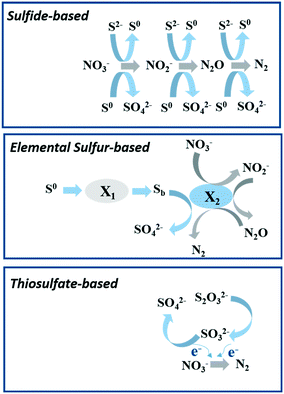 |
| Fig. 5 The proposed model for autotrophic denitrification with various sulfur compounds as the electron donor based on the description of former researchers.104,109,110 This figure was designed originally in this study. X1: hydrolytic biomass and X2: denitrifying biomass; Sb: bioavailable sulfur. | |
4.1 Microorganisms
A diversified microbial community is fundamental to the good performance of sulphur-driven autotrophic denitrification. Therefore, a comprehensive interpretation of microbial consortia involved in the sulphur-based denitrifying process is encouraged.95 Based on the functions of organisms, three major microbial populations are classified as follows (Table 3): sulphur-reducing bacteria (SRB), sulfur-driven denitrifiers (DNSOB), and heterotrophic denitrifiers.
Table 3 The functional microbial strains existing in sulfur-based denitrifying biofilm systems
Water type |
Reactor type |
Strain |
Function |
Description |
Ref. |
SRB: sulfur-reducing bacteria; DNSOB: sulfur-driven denitrifiers.
|
High-salt wastewater |
SBBR |
Desulfotignum; Desulfovibrio; Desulfatitalea |
SRB |
Utilizing various organic substances as the electron donor and carbon source for sulfate reduction |
Zhao et al. (2012)19 |
Psychrophilic temperature |
FBR |
Thiomicrospira denitrificans; Thiomicrospira thioparus |
SRB |
Di Capua et al. (2017)103 |
Refinery effluent; nitrate-contaminated water |
PBR; FBR |
Thiobacillus denitrificans; Sulfurimonas; Ignavibacteriales |
DNSOB |
Adopting S0 or S2O32− as the electron donor to conduct denitrification |
Wang et al. (2016);100 Zou et al. (2015);102 Zhang et al. (2015)20 |
Municipal wastewater |
PBR |
Hydrogenophilaceae; Comamonadaceae; Xanthomonadaceae |
DNSOB |
Kostrytsia et al. (2018)104 |
High-salt wastewater |
SBBR |
Thiohalobacter; Thioalkalivibrio; Arcobacter |
DNSOB |
Zhao et al. (2012)19 |
Refinery effluent |
PBR |
Curvibacter
|
Heterotrophic denitrifying bacteria |
Use soluble microbial products and cell lysis products as the carbon source for denitrification |
Wang et al. (2016)100 |
High-salt wastewater |
SBBR |
Defluviicoccus
|
Heterotrophic denitrifying bacteria |
Zhao et al. (2012)19 |
Anaerobic reduction of sulfate to sulfide is usually carried out by SRB for sulfate removal. The output sulfide can cause secondary contamination as addressed before.105 Fortunately, the sulfide can be further degraded by DNSOB under chemoautotrophic denitrifying conditions. Most of the DNSOB are ascribed to Proteobacteria showing capacity for autotrophic denitrification.106 The bacteria from the genera Thiobacillus, Sulfurimonas, and Ignavibacteriales have been widely found as the predominant consortium in S0- or S2O32-based autotrophic denitrification.20,104 Not all of the microbial consortium can perform complete denitrification. For example, Thiobacillus denitrificans and Thiobacillus thioparus are two ubiquitous obligate chemolithotrophs using S2O32− or S0 as an electron donor for NO3− reduction.103Thiobacillus denitrificans can reduce both NO3− and NO2− to N2 under chemolithotrophic conditions.95,102 However, unlike Thiobacillus denitrificans, Thiobacillus thioparus is a so-called “partial denitrifier”, which can only reduce NO3− to NO2−.103 Depending on the specific conditions of wastewater, the dominant genera of sulphur-driven denitrifiers can be diverted. For instance, Arcobacter, Thiohalobacter and Thioalkalivibrio were regarded as the predominant sulfur-driven denitrifiers in ultra-saline (>70 g L−1) wastewater.106
Analogous to hydrogen-driven denitrifying biofilm systems, the heterotrophs such as Chryseobacterium koreense and D. putealis were also identified in a sulfur-dependent denitrifying biofilm process.16 The growth of these two microorganisms was proposed to be maintained by endogenous metabolism and the organic substrate excreted by T. denitrificans.16 The presence of heterotrophs can solve some technique problems such as the clogging and channelling in the PBR due to biofouling.103
Conclusively, the success of the sulfur-based denitrification process relied largely on the microbial diversity. Three microbial consortia were differentiated based on their specific functions. Thiobacillus denitrificans and Thiobacillus thioparus are the two well-documented sulfur-reducing genera with the capacity to degrade nitrate and nitrite. Heterotrophic bacteria were also identified in sulfur-based biofilm denitrifying systems, which may promote sulfur-based denitrifying efficiency by preventing clogging issues in advance.
4.2 Biofilm reactors
Depending on the properties of the sulfur compound, the selection of biofilm reactors for conducting sulfur-based autotrophic denitrification is also verified. S0 is poorly soluble in water and is commonly used as the carrier material of the PBR.97 The FBR was also employed to enhance the mass transfer between the bulk liquid and biofilm when using S0 as an electron donor to reduce NO3−. S2O32− is a soluble and non-toxic sulfur compound. Analogous to S0, lab-scale FBRs with Thiobacillus-dominated biofilms were adopted to conduct thiosulfate-based denitrification. Given that S2− mainly exists in the form of gas (i.e., H2S), most of the studies on S2−-based denitrification were performed in reactors containing suspended cells to prompt the contact between the biomass and substrate. Studies regarding this process are rarely conducted in attached-growth systems.96 Vertical fixed-bed reactors and MBBRs were applied to carry out this autotrophic denitrification.96,105,107 The following section will introduce those biofilm reactors briefly combined with their flaws and merits from the perspective of technique feasibility.
PBRs.
Sulfur-based autotrophic denitrification for wastewater treatment has been widely-studied in PBRs, wherein S0 works as an electron donor and limestone (CaCO3) acts as a carbon source.1,102 The sulfur limestone autotrophic denitrification (SLAD) process is a cheap and easy solution to reduce nitrogen compounds, as S0 could act as a biofilm carrier to colonize microorganisms.1,104 The close contact between S0 particles and the bacteria from the biofilm is conductive for S0 solubilisation. Higher production of intermediate soluble sulfur compounds is then obtained, which are further oxidized to SO42− (Fig. 5).104 Therefore, the sulfur size can regulate the performance of SLAD in PBRs vastly. Pore clogging in the PBRs with S0 acting as a biofilm carrier usually results from 1) the formation of sulfide by disproportionation of S0 to SO42− and H2S; 2) the slough of biomass due to shear force; or 3) excessive biomass growth. Therefore, the clogging problem can hinder the large-scale application of PBRs in degrading nitrogen from sulfide-bearing wastewater.1 Besides cultivating the heterotrophic bacteria to consume the dead cells, ensuring the low nitrate loading by slowing down flow rates is another approach to maintain the high metabolic activity of the sulfur-oxidizing denitrifiers populating the biofilm while preventing pore clogging.103 The external supply of CaCO3 may induce low phosphorous bioavailability for bacterial growth by generating Ca3(PO4)2 and CaHPO4 precipitates.108 Therefore, Ca(HCO3)2 is adopted as an alternative to CaCO3 to buffer the acidity produced by the process while supplying an inorganic carbon source for microbial synthesis.
FBRs.
The performance of FBRs in sustaining S2O32−-driven denitrification was reported to be satisfactory under many conditions.97,102 Steady denitrifying performance was barely affected by the HRT, with high denitrifying efficiency (>90%) obtained at HRT ranging from 5.4 to 1 h.103 Anoxic thiosulfate oxidation with NO3− as an electron acceptor can be conducted in an wide range of temperatures.97 Even when the FBR was operated at psychrophilic temperatures (3 °C), complete thiosulfate-driven denitrification can still be achieved.103 All of those results indicated that the outstanding performance, robustness and resiliency of denitrification can be attained in the FBR with a denitrifying biofilm cultivated on S2O32−.
The FBR has also been applied to carry out SLAD due to the following reasons: 1) biofouling was alleviated by bed fluidization, enhancing the contact between the biomass and substrate subsequently,103 2) better mass transfer of NO3− to the biofilm attached on the sulfur surface; and 3) the absence of the clogging problem.108 However, the breakage of sulfur particles caused by high fluidization rates is a severe issue that needs to be addressed when using FBRs to run S0-based autotrophic denitrification. This issue might be resolved by adopting composite sulfur-based materials such as granular activated carbon impregnated with S0 as a biofilm carrier.108,111
Fixed-bed reactors and MBBRs for S2−-based denitrification.
Vertical fixed-bed reactors were applied to treat wastewater containing both sulfate and nitrogen pollutants.96,105 Plastic porous baffles were used as the support medium for biofilm growth. SRB were found in the biofilms when treating sulfate-laden wastewater, attaining sulfide due to sulfate reduction thereafter.105 As partial S2− oxidation requires fewer electron acceptors, low S/N could facilitate the complete sulfide oxidation subsequently.96 Heterotrophic denitrification was once integrated with S2− oxidation to achieve simultaneous nitrogen removal and sulfur recovery under high S/N ratios.105 Besides fixed-bed reactors, MBBRs can also offer a viable solution for effective SOAD biomass retention compared to suspended sludge.107 Higher populations of slow-growth organisms such as SOB can therefore be obtained in the MBBR.107 Additionally, the MBBR solved the difficulty in mass transfer between the bulk liquid and biofilm to some extent. However, the long start-up period of the MBBR for conducting the SOAD process is an obstacle to enlarge its scalability.107 Bioaugmentation by either seeding mature sludge or immobilizing SOB is an effective strategy to accelerate the start-up process.107,112
In summary, PBRs and FBRs are the two most-widely employed reactors to operate sulfur-based denitrification. FBRs can solve the biofouling problem existing in PBRs to some extent via fluidization. The sulfur size determines the denitrifying efficiency in both PBRs and FBRs by affecting the contact between the substrate and microbial consortium. Systematic evaluation of S2O32−-based denitrification in biofilms is presently lacking although fixed-bed reactors and MBBRs are applied to perform this autotrophic process. Considering the complex compositions of feeding wastewater and the intricate operational conditions, a clear understanding of the impact factors on the performance of autotrophic denitrification in biofilm reactors is needed.
4.3 Key affecting factors
For the biofilm systems used for sulfur-based denitrification, the associated denitrification efficiency can be vastly regulated by the biofilm development regarding thickness and biomass content. More specifically, the alteration of biofilm thickness could affect the interaction between the biomass and substrates. For instance, when the PBR was operated in the “counter-diffusional” mode (i.e., the electron donor and acceptor diffuse into biofilms from the opposite sides), the NO3− reduction rates increased as the biofilm thickness increased.100 Nevertheless, a decrease in NO3− reduction rates was observed when the biofilm thickness became excessive, as the electron donor and acceptor had longer diffusion distances due to the thicker biofilm, inducing lower concentrations when they meet.100 Therefore, a thick biofilm is not necessarily tied with the good performance of a sulfur-utilizing biofilm reactor for denitrification. The sulfur grain size affects the biomass content attached on S0, which then influences the SLAD performance. Small grain size usually leads to higher specific surface areas, which is favourable for biofilm growth and could promote the denitrification efficiency consequently.1 However, small sulfur particles may induce lower bed porosity, cause clogging and inhibit biofilm growth consequently as mentioned in section 4.2.
The impact of temperature on denitrifying biofilm systems is a topic of interest, as biofilm systems are supposed to be more resistant to temperature alteration than suspended-growth systems. Given that most of the well-studied sulfur-dependent genera are mesophilic species judging from their optimal growth temprtature,95 good performance of the sulfur-based biofilm denitrifying system was then obtained at 20 and 30 °C.102 It is noticeable that high-rate thiosulfate-driven denitrification can even be effectively sustained at 3 °C in an FBR with a Thiobacillus-dominated biofilm.103 Such outstanding denitrifying performance was mainly ascribed to the impressive tolerance of Thiobacillus to psychrophilic temperatures.102 This finding is exciting, as Thiobacillus is a widely-distributed denitrifier (see more in section 4.1), and the high denitrification efficiency of the FBR at low temperature allows the expansion of the scalability of sulfur-based denitrification for treating NO3− contaminated waters in cold regions.
High NO2− build-ups were observed in S0-based denitrifying biofilm systems when reactors were overloaded with NO3−.20,100 The presence of this unwanted intermediate via the nitrogen cycle is mainly due to: 1) slower NO2− reduction rates than those of NO3−;100 2) nitrate-reducing bacteria are easier to enrich than nitrite-reducing organisms; 3) nitrate reductases obtain a greater affinity for reduced electron mediators than nitrite reductases;113 and 4) slow S0 oxidation rates limit the supply of electrons for denitrifiers.113 These four problems can be solved by increasing electron supplementation through chemically-synthesized S0 powder addition while maintaining the high metabolic activities of the nitrite reducer simultaneously.113 Neutrophilic pH combined with mesophilic temperature is proposed to be the optimal conditions for achieving the full denitrification process driven by sulfur, as such conditions are deemed to be suitable for the growth of most denitrifiers.95
Altogether, to ensure the good performance of the biofilm system for denitrification utilizing sulfur as an electron donor, it is necessary to maintain the metabolic activities of denitrifiers by adjusting the temperature and pH. A thick biofilm is not linked with high organism metabolism for sure due to the sophisticated interactions between the biomass and substrates. Therefore, it is crucial to control the biofilm development regarding the thickness and biomass content for higher metabolic activities.
4.4 N2O production
The amount of N2O emitted from the sulfur-based denitrification process is much lower than that obtained from heterotrophic denitrification. Only 0.3% to 0.6% of removed nitrogen in the biofilm system was reported in the form of N2O when using sulfur or sulfur compounds as an electron donor.20 However, considering the great potential of this autotrophic denitrification in treating wastewater with insufficient organic matter and the hazardous effect of N2O on the atmosphere, it is necessary to reduce N2O formation during sulfur-driven autotrophic denitrification by controlling the operational conditions.
The ratio between S0 and nitrogen (S0/N) is an important factor affecting denitrification efficiency and N2O production. The increase in S0/N was reported to induce higher denitrification efficiency, as higher S0/N could relieve the competition among different nitrogen oxide reductases (i.e., nitrate, nitrite, NO and N2O reductases) regarding electron donor supplies.109 Lower N2O flux was then attained, since N2O reductase is the weakest competitor among the reductases.95 Different from elemental sulfur, high concentration of sulfide (∼300 mg TDS-S per L) would impose an inhibitory effect on N2O reduction.110 This is mainly because the redox active metal cofactor of N2O reductase such as copper may react with sulfide itself, producing Cu-containing sulfide precipitation and inducing inactive N2O reductase subsequently.109 Nevertheless, such toxicity and inhibitory effects on the enzyme could not be observed when the sulfide concentration is low.109 Moreover, low sulfide concentration could even stimulate the activity of N2O reductase.109 The stimulation can be explained by the continuous electron transport by the action of a series of enzymes in the sulfur-based denitrifiers.114 Specifically, the membrane-bound sulfide-quinone reductase assists the instant oxidation of sulfide into sulfur.114 The produced sulfur is then stored as sulfur globules in the periplasmic space, waiting to be mediated by N2O reductase.114 Hence, the copper co-factor in N2O will not be precipitated by the sulfide dissolving in the cell periplasm when the sulfide concentration is low.109,110
Besides the impact of sulfur or sulfide compound availability, the concentrations of nitrite and nitrate are also directly linked to N2O production in the sulfur-based denitrifying systems. Compared to nitrate, N2O production from the nitrogen cycle is more significant with nitrite as a terminal electron acceptor.95,99 This is due to the inhibitory effect induced by free nitrous acid on N2O reductase.95,99 Such inhibition can be alleviated or even omitted by reducing the electron competition among the different nitrogen oxide reductases by increasing the S/N mass ratio.100
Collectively, N2O production via sulfur-based biofilm denitrifying systems depends on nitrogen compound and sulfur/sulfide availabilities heavily. Regarding the autotrophic denitrification with S0, high S0/N combined with low FNA concentrations should be encouraged, as N2O emissions under these conditions could be lower due to sufficient electron supplement from S0 and slight inhibition on N2O reductase imposed by FNA. In terms of the autotrophic denitrification with sulfide, lowering sulfide concentrations might prevent or even completely avoid the N2O fluxes via sulfur-reducing denitrifiers. Of note is that although N2O production via sulfur-driven denitrification in suspended cultures is broadly studied, little is known about this topic in attached growth systems.
4.5 Coupling sulfur-based denitrification with anammox
Anammox is a nitrogen removal process in which NH4+ acts as an electron donor and NO2− functions as an electron acceptor via specialised anammox bacteria (eqn (17)).115,116 However, the produced NO3− in the reaction leads to a nitrogen reload into the wastewater and constrains the total nitrogen removal rate to maximal 90%.116 Coupling S0-based autotrophic denitrification with anammox is a strategy to remove the residual nitrate of the anammox process.117 More specifically, an integrated lab-scale set-up comprising an anammox and an elemental sulfur-supported packed bed has been established for achieving efficient total inorganic nitrogen removal (Fig. 6).117,118
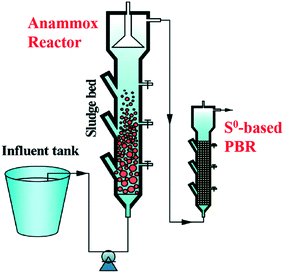 |
| Fig. 6 Schematic diagram of a coupling system consisting of anammox and S0-based PBR. Reproduced from ref. 116 with permission from [Royal Society of Chemistry], copyright 2020. | |
Anammox and S0-based denitrification are complementary in alkalinity (eqn (16) and (17)), since anammox consumes H+ and the other process produces H+.117 Such complementarity can then avoid the big variations of pH in the systems. Additionally, as NO2− is normally removed more slowly than NO3− when using the sulfur compound as an electron donor for denitrifiers, such combination can in turn avoid nitrite accumulation from happening in S0-based denitrifying systems.117 Both S2O32− in wastewater and S0 were jointly used as an electron donor and sulfur source for biomass proliferation.118 A biofilm was then attached on the surface of sulfur granules and gravel.118 However, due to the loose bond with the sulfur and biomass, the biofilm was easily extracted from the gravel, increasing the turbidity in the effluent.118 To further stabilize the performance of this integrated system, adding organic carbon into the reactor to establish a mixotrophic denitrifying process might be a solution. This is because the mixotrophic denitrification has higher transfer efficiencies of information and materials among the microbial consortium compared to solo autotrophic denitrification.117 Higher biomass content of the biofilm will be obtained, and the quality of the effluent from the system could be then improved.
| NH4+ + 1.32NO2− → 1.02N2 + 0.26NO3− + 2.03H2O | (17) |
4.6 Challenges and strategies for the application of sulfur-based denitrifying biofilms
Sulfur based denitrification is usually applied to remove the nitrogen from sulfide-bearing wastewater streams or groundwater polluted by NO3− with almost no organic matter.20,119 S0 or sulfide is usually considered as a good alternative to organic matter for an energy resource in denitrification since no organic residues would be left in the treated water. Of note is that water-insoluble S0 is stable under normal conditions and could be physically removed from the effluent for reuse.20 However, PBRs with S0 serving as the biomass carrier are often subject to clogging problems and nitrite accumulation control issues as described in section 4.2. Therefore, we restated the importance of seeking suitable sulfur grain size for enlargement of the scalability of PBRs performing autotrophic denitrification.
Although the FBR shows greater potential than the PBR towards higher denitrification efficiency, the clogging problem caused by the breakage of sulfur particles in the FBR can decrease the nitrate reduction rates. To further enhance the removal capacity of the FBR, the optimization of the reactor configuration by installing a membrane inside of the reactors was supposed to be a feasible method.20 However, membrane fouling is the new technical hurdle in applying this method.21 Low flow rates were proposed to address the occurrence of fouling.20 However, the lower active biomass concentration induced by fouling or low flow rates may prolong membrane operation.20
Overall, although sulfur-based autotrophic denitrification is a promising process for nitrogen removal from organic-deficient wastewater, challenges still exist to utilize this process in attached growth systems. Efforts are still required to handle the fouling issues present in various biofilm systems when utilizing sulfur or sulfur compounds as energy resources for denitrifiers.
5 Methane-driven denitrifying biofilm processes
The discovery of DAMO forms an important link between global carbon and nitrogen cycles, in which methane is oxidized anaerobically to provide electrons for NO2− or NO3− respiration (eqn (18) and (19)).25 CH4 can also be converted to complex organic carbon by methanotrophs in the presence of limited O2. The organic carbon then acts as a carbon source for denitrifiers to decompose NOx−. CH4 is a widely available and fairly inexpensive carbon source, since it is the main content of biogas generated from on-site anaerobic treatment of wastewater itself or from side-stream sludge digesters, providing a no-cost exogenous donor.24,26 Unlike conventional heterotrophic denitrification where post-denitrification might be required due to residual organics, CH4-driven denitrification could eliminate that step due to the poor solubility of CH4 in water. The features of CH4 may therefore potentially enlarge the scalability of CH4-based denitrification. However, the start-up of the reactor designed for methane-driven denitrification is difficult due to long doubling time of denitrifying methanotrophs.120 Given that biofilm reactors are suitable for the proliferation of slow-growing cultures such as DAMO organisms, the adoption of attached growth systems can therefore shorten the start-up of the reactor by reducing the enrichment period of functional cultures. | CH4 + NO3− → CO2 + 4NO2− + 2H2O | (18) |
| 3CH4 + 8NO2− + 8H+ → 3CO2 + 4N2 + 10H2O | (19) |
5.1 Microorganisms
The methane-supported nitrogen removal process is generally mediated by multiple microorganisms: denitrifying anaerobic methane oxidation (DAMO) archaea, DAMO bacteria, methanotrophs, and heterotrophic denitrifiers.
The populations of DAMO bacteria and DAMO archaea often occur concurrently in methane-driven denitrifying biofilm systems, which are deemed to have the ability to enhance nitrogen removal by using CH4 as an alternative carbon source (Table 4).22,121Candidatus Methylomirabilis and Methanoperedens nitroreducens are the two most representative DAMO organisms. Specifically, Candidatus Methylomirabilis affiliated to the NC10 phylum is a widely known bacterial DAMO organism with the capability to couple nitrite reduction with anaerobic oxidation of methane (eqn (19)).22,122 The archaeal DAMO organism, Methanoperedens nitroreducens from ANME-2D, can oxidize CH4 through reverse methanogenesis using NO3− as the terminal electron acceptor (eqn (18)).120 Besides the microbial consortium introduced herewith, other kinds of DAMO organisms were also considered to obtain the similar function as listed in Table 6. Some DAMO cultures including Rhodocyclaceae need to be further verified, as their role in the methane-driven denitrification process is still not clear.25
Table 4 Functional organisms involved in the methane-driven denitrification process
Organisms |
Families |
Representative genus |
Function |
Ref. |
DAMO bacteria |
Methylomirailiaceae |
Candidatus ‘Methylomirabilis oxyfera’ |
Widely distributed in both natural habitats and biofilm reactors. Coupling methane oxidation to nitrite reduction through an intra-aerobic methane oxidation pathway |
Haroon et al. (2013)122 |
DAMO bacteria |
Betaproteobacteria |
|
Being able to compete with anammox for nitrite; integrating nitrate reduction with the oxidation of metabolites obtained from anammox bacteria |
Xie et al. (2018)121 |
DAMO bacteria |
Anaerolineae |
|
Using CH4 as an electron donor to reduce nitrogenous pollutants |
Xie et al. (2017)25 |
DAMO bacteria |
Gammaproteobacteria |
|
Reduce nitrite to dinitrogen with the oxidation of methane |
Xie et al. (2018)121 |
DAMO archaea |
ANME-2D |
Candidatus ‘Methanoperedens nitroreducens’ |
Anaerobically oxidizing methane via reverse methanogenesis using NO3− as a terminal helectron donor |
He et al. (2014)120 |
Other DAMO organisms |
Rhodocyclaceae
|
|
The role of Rhodocyclaceae has not been well elucidated |
Xie et al. (2017)25 |
Heterotrophs |
See section 2.1 |
See section 2.1 |
Living on the microbial products excreted by denitrifying methanotrophs |
He et al. (2015)26 |
Methanotrophs |
|
Methylomicrobium alcaliphilum
|
Capable of using O2 within the biofilm and create an anoxic micro-environment for denitrifiers |
Kalyuzhnaya et al. (2013)123 |
Although no organic matter is supplied to the feed, the presence of heterotrophic denitrifiers in the methane-driven denitrifying systems seems to be unavoidable, as the heterotrophs can live on the microbial products such as EPS excreted by DAMO organisms.26
Therefore, a portion of NOx− is decomposed by heterotrophic denitrification in the CH4-dependent denitrifying reactors. The mutualism established between methanotrophs, heterotrophic denitrifiers and denitrifying methanogens can be delineated as shown in Fig. 7. Of note is that methanotrophs such as Methylomicrobium alcaliphilum could convert CH4 to soluble metabolites such as methanol, acetate, etc. which then serve as the carbon source for heterotrophic denitrifiers (Fig. 7).123 Moreover, the presence of methanotrophs could create an anoxic micro-environment for denitrifiers in the biofilm by consuming O2 on the surface.124 Therefore the cooperation among DAMO organisms, methanotrophs, and heterotrophs ensures the high NOx conversion efficiency in the CH4-supported biofilm denitrifying systems.
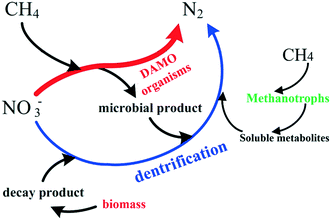 |
| Fig. 7 The schematic interactions among heterotrophs, methanotrophs and DAMO organisms regarding NO3− removal in CH4-driven denitrifying systems. The red arrow indicates the NO3− reduction by DAMO organisms. The blue arrow refers to the NO3− reduction via heterotrophic denitrification. Designed originally in this study. | |
5.2 Methane based membrane biofilm reactors
MBfRs have been widely employed to conduct the CH4-supported denitrification process.25,121 The membranes inside of the MBfR provide a technological solution for overcoming the low solubility of CH4. In detail, since the CH4 gas is fluxed directly to the biofilm through gas-permeable membranes rather than via the bulk liquid and diffusion layer, a better methane transfer efficiency could be attained. The DAMO organisms and methanotrophs immobilized on the biofilm consume the CH4 concurrently and quickly, avoiding the risk of CH4 loss thereafter. The CH4 diffusion from the gaseous to aqueous phase could be further enhanced by elevating gaseous pressures. High concentration of functional organisms can be achieved in the MBfR, as the co-existence of DAMO organisms, heterotrophs and methanotrophs is better protected in biofilm systems than that in suspended systems. Collectively, the MBfR shows advantages in CH4 transfer and utilizing efficiency. The special configuration of the MBfR could speed up biomass growth compared to suspended sludge systems. To further verify the feasibility of biofilm systems in conducting methane-driven denitrification, it is encouraged to adopt other kinds of attached growth systems aside from the MBfR.
5.3 Key affecting factors
Multiple factors can impact the CH4-based denitrification efficiency significantly by regulating the interaction among functional organisms, including the temperature, pH conditions, oxygen level in the feed, and HRT.
As most of the DAMO organisms are mesophilic cultures, the optimal temperature for DAMO organism activity was reported to be around 35 °C according to the short-term batch tests conducted by He et al. (2015).120 However, a lower contribution of DAMO organisms to the removal of total nitrogenous pollutants was obtained at 35 °C, since high temperature also promoted the activity of heterotrophic denitrifiers.120 Aside from the higher activity of heterotrophic denitrifiers stimulated by temperature, the core reason for this phenomenon was the increasing decay rate of biomass, which acted as a carbon source for the growth of heterotrophs.120 Notably, the DAMO bacteria were inactivated when the temperature was above 45 °C, probably due to one or more essential cell components such as the key enzyme were damaged under high-temperature conditions.125 Therefore, CH4 driven denitrification is temperature-dependent. Maintaining the temperature conditions around 35 °C might be a key to ensure high denitrification efficiency in the biofilm systems.
A pH range of 7.0–8.0 is suitable to the activity of DAMO organisms without affecting their contribution to nitrogen removal.120 The activity of these organisms would be suppressed significantly when the pH is above 9.0. So maintaining the pH around neutral values is necessary to achieve a stable performance of methane-supported denitrification in biofilm reactors.120
The effect of O2 on denitrification efficiency is inevitable when adopting methane-based denitrification to treat water containing a certain amount of O2. More specifically, CH4 is oxidized into volatile fatty acids (VFAs) by methanotrophs under oxygen-limited conditions, and NOx—is then decomposed by heterotrophic denitrifiers with VFA serving as the carbon source.22 Although the cooperation between methanotrophs and heterotrophs could maintain the micro-anaerobic conditions in the biofilm, the excessive O2 level may deteriorate the stability of the CH4-based denitrification process. To further ensure the high efficiency of nitrogen removal under aerobic conditions, prolonging the HRT to a certain extent is helpful. A too long HRT such as 5 days may induce VFA accumulation, requiring an additional step to remove organic residues.22
In summary, the synergistic interactions among the functional organisms in the biofilm systems are vastly affected by the change of operational conditions, impacting the final effluent quality consequently. Natural pH, mesophilic temperature and long HRT are summarized as the suitable conditions for achieving stable CH4-dependent denitrification, as such conditions are optimal for the growth of the microbial consortium involved in this denitrifying process.
5.4 Coexistence with anammox
The application of anammox to treat wastewater is hindered due to the high NO3− concentration obtained via anammox organisms, reducing the overall nitrogen removal efficiency and impeding its engineering application.29 However, the DAMO process provides an opportunity for enhancing the nitrogen removal via the anammox process utilizing biogas methane as a cheap, supplementary electron donor (Fig. 8). Specifically, the NO2− obtained from DAMO archaea is rapidly utilized by anammox bacteria (eqn (17)), and the NO3− produced via the anammox reaction is then consumed by DAMO bacteria in the absence of oxygen (eqn (20)).126 | NH4+ + 1.06NO2− + 0.065CH4 → 1.02N2 + 2.16H2O + 0.065CO2 | (20) |
The key to conduct the anammox reaction successfully is the strict maintenance of the NO2− to NH4+ ratio around 1.32,127 which is not always satisfied in practice with one or two substrates remaining in the effluent. However, because of the synergistic relationship among DAMO organisms and anammox bacteria, the requirement of that ratio could be more flexible.25,121 The robustness of the biofilm system regrading nitrogen removal can be then enhanced by the combination of DAMO and anammox.
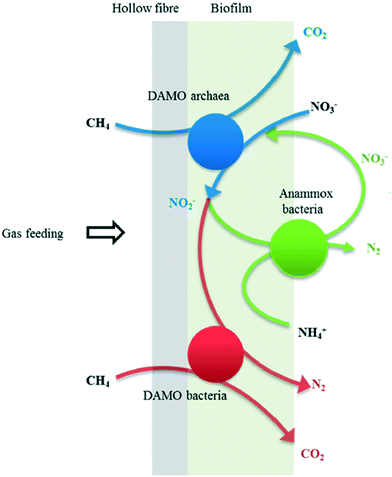 |
| Fig. 8 Proposed biochemical reactions and the interaction between DAMO organisms and anammox bacteria in the MBfR. Reproduced from ref. 129 with permission from [ACS Publications], copyright [2020]. | |
Given that the biofilm is the biomass aggregate type which enables high retention for microorganisms with a slow growth rate, the MBfR was hence adopted to co-culture anammox bacteria and DAMO organisms simultaneously while removing nitrogenous pollutants.26 In most cases, the anammox process took the major responsibility for nitrogen removal, while nitrite reduction by DAMO bacteria played a relatively minor role.25,121 The nitrate reduction rate by DAMO archaea and the nitrite reduction rate by DAMO bacteria were found to be increased after increasing the CH4 partial pressure in the MBfR.128 However, a noticeable rise of dissolved CH4 concentration in the effluent could be observed regardless of increasing methane utilization efficiency by DAMO organisms.128 Additional steps were then required to treat excessively dissolved CH4.
Taken together, the partnership between anammox bacteria and DAMO organisms could solve the problem regarding varying NO2−/NH4+ ratio in the feed for the anammox process to some extent. Co-culturing those microorganisms in the MBfR is a feasible way to improve the nitrogen removal efficiency in the anammox process while using CH4 as an externally provided electron donor. Anammox bacteria were the larger contributor to nitrogen removal in comparison with DAMO organisms in most cases.
5.5 Challenges and strategies for the application of CH4-driven denitrifying biofilms
A number of bottlenecks should be overcome when using CH4-based denitrification to treat wastewater, such as improving the CH4 purity in biogas, shortening the enrichment period, enhancing the CH4 solubility, improving the biological activity and strengthening the process robustness.120
The actual raw biogas produced via anaerobic digestion constitutes high levels of CO2 (30–50%) and CH4 (50–70%) depending on the specific operational conditions.23 Such low CH4 percentage implies the need to upgrade the biogas to avoid acidification induced by excessive CO2 ingress during the CH4-based denitrification process.130 Fortunately, multiple approaches such as chemical, physical and biological techniques have been proposed and practiced to remove undesired CO2 and increase the CH4 content. The information regarding the CO2 upgrading process has been comprehensively review by others.131 Additionally, the H2S present in the crude biogas may suppress anammox; however, this unwanted component can be utilized by DNSOB to convert nitrogen pollutants into N2.
The difficulties in enriching DAMO organisms and improving the substrate utilization kinetics could be solved in the MBfR, where the CH4 transfer efficiency is regulated and the biomass is enriched by colonizing microorganisms on the membrane.24 To further increase the low anaerobic oxidation rates due to low methane kinetics, increasing the partial methane pressure in the MBfR is a proposed strategy which needs further optimization. This is mainly because of the concern regarding the potential CH4 leaking.132 The biological activity of functional organisms can be improved by optimizing the operational conditions when conducting CH4-supported denitrification.
In terms of the challenge regarding strengthening the process robustness, the development of synergistic processes such as autotrophic with heterotrophic denitrification, or DAMO with anammox is a feasible way to overcome that barrier.25,26 These cooperative processes have shown great potential for treating wastewater containing a high-level of NH4+.25,26
Overall, although methane-driven denitrification exhibits potential in treating wastewater with a high amount of nitrogenous pollutants, the application of this process is still challenging after considering its technical barriers. Several strategies have been suggested to overcome those challenges. Lowering the CO2 content in the biogas, adopting the MBfR to carry out the synergistic processes and optimizing the conditions for high biological activity are probably the solutions to achieve high nitrogen removal efficiency via CH4-driven denitrification.
6 Other denitrifying processes in biofilm systems
6.1 Fe(II)-Based
Fe(II)-Driven autotrophic denitrification is an alternative to heterotrophic denitrification, in which denitrifying bacteria could grow anaerobically with ferrous iron as the electron donor while recovering iron through the formation of Fe(III) precipitates (eqn (21)).133 Fe(II)-Driven autotrophic denitrification in biofilm reactors has gained some interest in recent years. PBRs and MBBRs have been proven to be very effective for iron-based denitrification.133,134 The well-known functional denitrifiers including Thiobacillus and Pseudomonas were seeded to those reactors to enhance nitrogen removal, obtaining better nitrogen removal efficiencies compared to the reactors without immobilization.133,135 Independent of the good denitrifying performance, there is a need to address the concern regarding the encrusted cells caused by generated Fe(OH)3 adhering onto the cell surface. This is because Fe(III)-hydroxide encrustation can decrease the nutrients and substrate uptake rates, leading to the loss of cells with normal metabolic activity thereafter.134 EDTA was supplied to enhance biofilm formation by chelating Fe(II) to avoid Fe(III)-hydroxide encrustation, allowing a prolonged activity of microbes.133,134 Analogous to other denitrifying processes, the Fe(II)-based denitrification performance can be affected by pH, HRT and substrate availability. Acidic pH and longer HRT are beneficial to the removal of NO3− and Fe(II).135 However, a longer HRT is not conductive to performing this denitrification process at a larger scale. Therefore, NO2− accumulation caused by high nitrogen rates should be avoided as this may induce serious cell encrustation due to the consumption of Fe2+ by NO2−.134 | 10Fe2+ + 2NO3− + 24H2O → N2 + Fe(OH)3 + 18H+ | (21) |
6.2 Pyrite-based
Pyrite (FeS2) is an abundant disulphide mineral in the Earth's crust, which has been regarded as an alternative electron donor for autotrophic denitrification (eqn (22)).136,137 Pyrite has been used as a packing medium in PBRs for nitrate removal.138 Compared to sulfur-based denitrification, pyrite-oxidizing autotrophic denitrification generates less SO42− when reducing the same amount of nitrate.138 However, the production of H+via FeS2-driven denitrification would decrease the pH (<6.5) and inhibit the microbial denitrification activities; it is therefore necessary to minimize the detrimental effects of acidity. Researchers have developed two strategies to tackle that issue by 1) finding suitable alkaline materials to maintain the pH conditions; or 2) integrating FeS2-based denitrification with other denitrifying processes. | 15NO3− + 5FeS2 + 10H2O → 7.5N2 + 10SO42− + 5Fe(OH)3 + 5H+ | (22) |
Low-cost alkaline materials such as oyster shells and limestone are good buffering reagents when treating wastewater containing low levels of nitrate (10–100 mg N per L).137 Moreover, as materials with a rough surface could facilitate biofilm attachment, and the large surface area of oyster is conductive to biofilm growth, higher biomass contents are then obtained in the biofilm reactor with oyster shells. Nevertheless, if the oyster-containing reactor was employed for wastewater with higher NO3− content (>100 mg L−1), a decrease in the denitrification efficiency was observed, probably due to the insufficient pH buffer capacity of oyster and limestone.136 As alkaline products were obtained via H2-based (eqn (7)) and conventional heterotrophic denitrification (eqn (8)), these two denitrifying processes were coupled with pyrite-based denitrification to avoid the reduction of pH in the reactor.137,138 Moreover, this combined system can also solve the limitation of buffering reagent addition and could be applied to treat wastewater containing high NO3− content (>100 mg L−1).137,138 Although Pseudomonas and Thiobacillus denitrificans might have the specific ability to conduct pyrite-oxidizing denitrification, the exclusive microbial consortium responsible for FeS2-based denitrification has not been determined for sure.137 Intensive efforts are warranted regarding this issue.
6.3 Arsenite-based
Arsenite-driven denitrification is of high interest for public health and economy, as it can simultaneously remove arsenic and nitrate, increasing the drinking water availability by solving the severe arsenic contamination of groundwater.97 BERs have been adopted to convert toxic As(III) to nontoxic As(V) in the anodic chamber while denitrifying NO3− to N2.139 Autotrophic As(III)-oxidizing bacteria, including Achromobacter spp., Ensifer spp., and Sinorhizobium spp., were found to colonize on the surface of the anode to degrade As(III).139 Studies regarding arsenite-based denitrification in biofilm systems are scarce. Future studies should focus on conducting this autotrophic denitrification process in various biofilm systems.
7 Differences between biofilms and suspended growth systems for denitrification
The representative microorganisms and metabolic pathways for nitrogen pollutant degradation in biofilms are similar to those in suspended sludge (Table 5), but the observed behaviour may be different. More specifically, substrate gradients can be observed due to diffusion and reactions within biofilms. Such gradients are usually not present in suspended sludge (SS). The microorganisms were then stratified into different layers, leading to various types of metabolisms dominating at different depths within the biofilms. Therefore, the microbial community compositions and associated interactions in the biofilm are distinct from those in the SS systems. Such distinction makes the biofilm thickness, mixing regime, and bulk substrate concentrations become the unique factors for controlling the denitrification performance.68 For more details regarding these unique factors on denitrification, please refer to sections 2.3, 3.3, and 4.3.
Table 5 Key denitrifiers in the biofilm and suspended sludge systems
Species |
Function |
Biofilm reactor |
Existence in SS |
Thauera-like bacteria |
Heterotrophic and hydrogenotrophic denitrifiers |
PBR, BER |
Yes |
Ottowia
|
|
MBBR–MBR |
Yes |
Thermomonas
|
|
PBR, MBBR-MBR |
Yes |
Paracoccus pantotrophus
|
A heterotrophic nitrifier and aerobic denitrifier |
RBC |
Yes |
Comamonadaceae
|
Hydrogenotrophic denitrifier |
HEAD-BER |
Yes |
Thiobacillus
|
Oxidizing S2−, S2O32−, and S0 to SO42− |
PBR, FBR |
Yes |
Sulfurimonas
|
S0-Based autotrophic denitrifier |
PBR, FBR |
Ill-identified |
Ignavibacteriales
|
S0-Based autotrophic denitrifier |
PBR, FBR |
Ill-identified |
Candidatus Methylomirabilis
|
DAMO bacteria |
MBfR |
Yes |
Methanoperedens nitroreducens
|
DAMO archaea |
MBfR |
Yes |
The change in the kinetic coefficient (Ks) of the biofilm can also be ascribed to the variations of the abovementioned unique factors. For instance, different mixing regimes yield different hydrodynamic shear forces, controlling the biofilm structure and altering the nitrogen removal rates accordingly.140 Applying high shear force proved to be effective in improving denitrification rates by reducing the thickness of the biofilm via biomass detachment.90 Other operational conditions including the substrate type, pH, and temperature are also closely related to the denitrifying kinetics in the biofilm (Table 6). For example, the Ks was reported to be 140–190 mg NO3-N g per VSS d−1 in the S0-supported denitrifying systems at 25 °C.141 When adopting H2 as an electron donor, the denitrification rates were then changed to 210–740 mg NO3-N g per VSS d−1 under similar conditions.91 Although intensive efforts have been done to evaluate the Ks of different denitrifying biofilms with the assistance of mathematical modelling,74 it is still difficult to systematically summarize the Ks in biofilms given the uneven structure of biofilms and non-uniformed operational conditions.
Table 6 A comparison between nitrate reduction rates by denitrifiers using different substrates under various conditions
Electron donor |
Biomass con. (g L−1) |
Temp. (°C) |
pH |
Nitrate reduction rate (NO3-N g per VSS d−1) |
Ref. |
S2O32− |
NG |
33–25 |
6.5–7.5 |
7.2–9.6 |
Oh et al. (2000)142 |
S0 |
1.19–6.61 |
25 |
7–8 |
0.14–0.19 |
Koenig and Liu (2004)143 |
H2 |
0.5 |
25 |
7.5–9.5 |
0.38–0.74 |
Rezania et al. (2005)91 |
H2 |
0.5 |
12 |
7.5–9.5 |
0.21–0.28 |
Rezania et al. (2005)91 |
A wide range of biofilm reactors have been designed to conduct denitrification. They can be classified based on the diffusion pattern of the electron donor or acceptor within the biofilm, the form of carrier for biomass attachment (fixed or moving), the mixing regime (completely mixed or plug flow), etc.64 Considering that the types of electron donors for various denitrifying processes are different, the designs of the biofilm reactors and associated impact factors are also diversified. A summary of the distinctions and similarities among various denitrifying biofilm reactors is given based on the discussion of this review (Table 7). Thus, for the purpose of high overall nitrogen removal efficiency, the selection of biofilm reactor should be based on the system characteristics and specific wastewater compositions.
Table 7 Similarities and distinctions of various biofilm reactors for conducting heterotrophic or autotrophic denitrification from the aspect of configuration and operation mode
Reactor type |
Heterotrophic |
H2-Based |
Sulfur-based |
CH4-Based |
ND: not discussed.
|
PBR |
Configuration: a typical submerged fixed biofilm reactor with porous materials as biofilm carriers |
Configuration: similar to a typical PBR with an electrode to produce internal H2via water electrolysis, or a membrane to increase H2 solution externally |
Configuration: similar to a typical reactor using S0 to colonize biomass |
ND |
Operational mode: up-flow or down-flow |
Operational mode: up-flow or down-flow |
Operational mode: up-flow or down-flow |
Unique impact factor: S0 gravel size |
FBR |
Configuration: biofilm holder made from various support-media, with wastewater flowing through in the centre |
ND |
Configuration: similar to a typical reactor when using S2O32− as an electron donor |
ND |
S0 works as a support-medium when conducting SLAD. The breakage of S0 may cause clogging |
Operational mode: flow-through or dead-end |
Operational mode: flow-through or dead-end |
MBfR |
ND |
Configuration: a cylinder container with a gas permeable membrane working as both a gas diffuser and biofilm carrier |
ND |
Configuration: similar to a typical MBfR with DAMO with methanotrophs and heterotrophs colonized on the membranes |
Operational mode: flow-through or dead-end |
Operational mode: flow-through or dead-end |
BER |
Configuration: composed of electrodes, poised electrical power and bipolar membrane |
Similar to the reactor used in heterotrophic denitrification |
ND |
ND |
Usually integrated into the PBR or MBR to enhance the treatment capacity |
RBC |
Configuration: discs connected to the shaft in series, with the biofilm immobilized on the discs |
ND |
ND |
ND |
May be coupled with electrodes to remove organic pollutants completely when treating wastewater with high nitrogen but low carbon |
Conclusively, the differences between the biofilm and SS regarding biomass structure determine their substrate transfer patterns and substrate competition among functional organisms. The design of the reactor could affect the biofilm characteristics. To fully reveal the mechanisms of denitrifying biofilm systems, future studies should aim at understanding the joint impact of factors such as biofilm thickness, mixing regime, etc. on denitrification performance in the attached-growth reactors.
8 Conclusions and outlook
Heterotrophic denitrification is a conventional approach to remove nitrogen contaminants using attached growth systems. The dominant nitrite/nitrate-reducing organisms in the denitrifying systems are determined by the specific operational conditions and configuration of the reactors. The mixotrophic denitrification process in integrated attached growth systems is a promising process to treat nitrogen-contaminated wastewater without worrying about the overdosage of external carbon. To further expand the scalability of heterotrophic denitrification and sequestrate N2O emissions by heterotrophs, pilot-scale applications of the integrated systems are expected in the near future.
Considerable ongoing efforts have been focused on hydrogenotrophic denitrification of wastewater characterized by low C/N ratios. Besides hydrogenotrophic denitrifiers, heterotrophic denitrifiers were also observed in the biofilm attachment systems. To increase the H2 availability and increase the denitrifying efficiency, biofilm reactors were redesigned to include an electrode for water electrolysis and an additional membrane for biomass attachment. Low H2 solubility and pH variation are the two main factors controlling the performance of H2-based denitrifying biofilm systems. Extensive research is needed to address the N2O emission pattern in the biofilm systems, as a better understanding of the impacts of process configurations on N2O emissions can help to minimize N2O production from biofilm processes for wastewater treatment.
Sulfur-based autotrophic denitrification is a promising and cost-effective process for nitrogen removal from waters poor in organics. Three different groups of microorganisms have been classified based on their functions. The roles of Thiobacillus denitrificans and Thiobacillus thioparus have been well-documented as sulfur-driven denitrifiers. S0 can act as a biomass carrier in both the PBR and FBR, enhancing the denitrification efficiency by promoting the solubilization of elemental sulfur. Fouling might occur due to the small pore caused by small sulfur grain size or sulfur breakage, signifying that future research dedicated to prompting organism metabolisms by avoiding fouling is warranted. Much of the past research regarding N2O emissions from sulfur-based denitrification was mainly based on suspended growth systems rather than biofilm reactors. More investigations are required to investigate the N2O production from attached growth systems using sulfur as an energy resource.
Given that CH4 from biogas can be utilized directly as an electron donor to drive the denitrification process, CH4-supported denitrification was hence proposed as a widely-applicable alternative to remove nitrogenous pollutants from wastewater. The MBfR is a good biofilm reactor to conduct this denitrifying process due to its advantages in high biomass retention by biofilm development and efficient methane delivery. The cooperation among the diversified microorganisms in the MBfR ensures the high denitrification efficiency. The robustness of the system can also be enhanced by the synergistic interaction among organisms. Enlarging the scalability of CH4-based denitrification in the MBfR is hindered by some technique problems such as low methane content in the raw biogas. More studies are warranted to verify the feasibility of fostering DAMO organisms using raw biogas directly in the MBfR.
Several biofilm systems have been adopted to conduct ferrous iron-, pyrite, and arsenite-driven denitrification in recent years. Cell encrustation and acidic conditions are the main limitations for upscaling the feasibility of Fe(II)-based and pyrite-driven denitrification, respectively. To pave the way towards the upscaling of these two nitrate removal biotechnologies, future developments should aim at solving those two issues. The large-scale feasibility of arsenite-driven denitrification still needs further verification, as only limited studies focused on this biotechnology in the attached-growth systems.
Conflicts of interest
There are no conflicts to declare.
Acknowledgements
This work is supported by an Australian Research Council (ARC) Future Fellowship (FT160100195). Ms. Lan Wu was supported by the China Scholarship Council (File No. 201806330112) for a PhD scholarship at the University of Technology Sydney.
References
- F. Di Capua, S. Papirio, P. N. L. Lens and G. Esposito, Chemolithotrophic denitrification in biofilm reactors, Chem. Eng. J., 2015, 280, 643–657 CrossRef CAS.
- I. Klodowska, J. Rodziewicz, W. Janczukowicz, A. Gotkowska-Plachta and A. Cydzik-Kwiatkowska, Hydrogenotrophic denitrification process efficiency and the number of denitrifying bacteria (MPN) in the sequencing batch biofilm reactor (SBBR) with platinum and carbon anodes, J. Environ. Sci. Health, Part A: Toxic/Hazard. Subst. Environ. Eng., 2016, 51, 389–392 CrossRef CAS.
- P. Li, Y. Wang, J. Zuo, R. Wang, J. Zhao and Y. Du, Nitrogen Removal and N2O Accumulation during Hydrogenotrophic Denitrification: Influence of Environmental Factors and Microbial Community Characteristics, Environ. Sci. Technol., 2017, 51, 870–879 CrossRef CAS.
- Y. Zhao, C. Feng, Q. Wang, Y. Yang, Z. Zhang and N. Sugiura, Nitrate removal from groundwater by cooperating heterotrophic with autotrophic denitrification in a biofilm-electrode reactor, J. Hazard. Mater., 2011, 192, 1033–1039 CrossRef CAS.
-
WHO, Guidelines for drinking-water quality, incorporating first and second addenda, Recommendations, World Health Organization, Geneva, 3rd edn, 2008, vol. 1 Search PubMed.
- A. Gupta, Simultaneous carbon and nitrogen removal from high strength domestic wastewater in an aerobic RBC biofilm, Water Res., 2001, 35, 1714–1722 CrossRef CAS.
- Y. F. Cheng, G. F. Li, Y. Y. Liu, B. Q. Zhu, Q. Zhang, Y. Xue, Z. Z. Zhang and R. C. Jin, Evaluating the effects of Zn(II) on high-rate biogranule-based denitrification: Performance, microbial community and sludge characteristics, Bioresour. Technol., 2019, 279, 393–397 CrossRef CAS.
- Y. Pan, Y. Liu, L. Peng, H. H. Ngo, W. Guo, W. Wei, D. Wang and B. J. Ni, Substrate Diffusion within Biofilms Significantly Influencing the Electron Competition during Denitrification, Environ. Sci. Technol., 2019, 53, 261–269 CrossRef CAS.
- Y. F. Cheng, Q. Zhang, G. F. Li, Y. Xue, X. P. Zheng, S. Cai, Z. Z. Zhang and R. C. Jin, Long-term effects of copper nanoparticles on granule-based denitrification systems: Performance, microbial communities, functional genes and sludge properties, Bioresour. Technol., 2019, 289, 121707 CrossRef CAS.
- P. Albina, N. Durban, A. Bertron, A. Albrecht, J. C. Robinet and B. Erable, Influence of Hydrogen Electron Donor, Alkaline pH, and High Nitrate Concentrations on Microbial Denitrification: A Review, Int. J. Mol. Sci., 2019, 20, 5163 CrossRef CAS.
- S. He, L. Ding, X. Wang, Y. Pan, H. Hu, K. Li and H. Ren, Biochar carrier application for nitrogen removal of domestic WWTPs in winter: challenges and opportunities, Appl. Microbiol. Biotechnol., 2018, 102, 9411–9418 CrossRef CAS.
- S. F. Korom, Natural denitrification in the saturated zone: A review, Water Resour. Res., 1992, 28, 1657–1668 CrossRef CAS.
- D. Ucar, E. Ubay Cokgor and E. Sahinkaya, Simultaneous nitrate and perchlorate reduction using sulfur-based autotrophic and heterotrophic denitrifying processes, J. Chem. Technol. Biotechnol., 2016, 91, 1471–1477 CrossRef CAS.
- T. Peng, C. Feng, W. Hu, N. Chen, Q. He, S. Dong, Y. Xu, Y. Gao and M. Li, Treatment of nitrate-contaminated groundwater by heterotrophic denitrification coupled with electro-autotrophic denitrifying packed bed reactor, Biochem. Eng. J., 2018, 134, 12–21 CrossRef CAS.
- V. Matějů, S. Čižinská, J. Krejčí and T. Janoch, Biological water denitrification—A review, Enzyme Microb. Technol., 1992, 14, 170–183 CrossRef.
- F. Di Capua, I. Milone, A. M. Lakaniemi, E. D. V. Hullebusch, P. N. L. Lens and G. Esposito, Effects of different nickel species on autotrophic denitrification
driven by thiosulfate in batch tests and a fluidized-bed reactor, Bioresour. Technol., 2017, 238, 534–541 CrossRef CAS.
- J. H. Park, O. Choi, T. H. Lee, H. Kim and B. I. Sang, Pyrosequencing analysis of microbial communities in hollow fiber-membrane biofilm reactors system for treating high-strength nitrogen wastewater, Chemosphere, 2016, 163, 192–201 CrossRef CAS.
- J. Wang, H. Lu, G. H. Chen, G. N. Lau, W. L. Tsang and M. C. van Loosdrecht, A novel sulfate reduction, autotrophic denitrification, nitrification integrated (SANI) process for saline wastewater treatment, Water Res., 2009, 43, 2363–2372 CrossRef CAS.
- Y. Zhao, B. Zhang, C. Feng, F. Huang, P. Zhang, Z. Zhang, Y. Yang and N. Sugiura, Behavior of autotrophic denitrification and heterotrophic denitrification in an intensified biofilm-electrode reactor for nitrate-contaminated drinking water treatment, Bioresour. Technol., 2012, 107, 159–165 CrossRef CAS.
- L. Zhang, C. Zhang, C. Hu, H. Liu and J. Qu, Denitrification of groundwater using a sulfur-oxidizing autotrophic denitrifying anaerobic fluidized-bed MBR: performance and bacterial community structure, Appl. Microbiol. Biotechnol., 2015, 99, 2815–2827 CrossRef CAS.
- J. Kim, K. Kim, H. Ye, E. Lee, C. Shin, P. L. McCarty and J. Bae, Anaerobic fluidized bed membrane bioreactor for wastewater treatment, Environ. Sci. Technol., 2011, 45, 576–581 CrossRef CAS.
- J. H. Luo, H. Chen, Z. Yuan and J. Guo, Methane-supported nitrate removal from groundwater in a membrane biofilm reactor, Water Res., 2018, 132, 71–78 CrossRef CAS.
- O. W. Awe, Y. Zhao, A. Nzihou, D. P. Minh and N. Lyczko, A Review of Biogas Utilisation, Purification and Upgrading Technologies, Waste Biomass Valorization, 2017, 8, 267–283 CrossRef CAS.
- H.-S. Lee, Y. Tang, B. E. Rittmann and H.-P. Zhao, Anaerobic oxidation of methane coupled to denitrification: fundamentals, challenges, and potential, Crit. Rev. Environ. Sci. Technol., 2018, 48, 1067–1093 CrossRef CAS.
- G. J. Xie, C. Cai, S. Hu and Z. Yuan, Complete Nitrogen Removal from Synthetic Anaerobic Sludge Digestion Liquor through Integrating Anammox and Denitrifying Anaerobic Methane Oxidation in a Membrane Biofilm Reactor, Environ. Sci. Technol., 2017, 51, 819–827 CrossRef CAS.
- Z. He, J. Wang, X. Zhang, C. Cai, S. Geng, P. Zheng, X. Xu and B. Hu, Nitrogen removal from wastewater by anaerobic methane-driven denitrification in a lab-scale reactor: heterotrophic denitrifiers associated with denitrifying methanotrophs, Appl. Microbiol. Biotechnol., 2015, 99, 10853–10860 CrossRef CAS.
- K. J. Martin and R. Nerenberg, The membrane biofilm reactor (MBfR) for water and wastewater treatment: principles, applications, and recent developments, Bioresour. Technol., 2012, 122, 83–94 CrossRef CAS.
- A. Abdelfattah, M. I. Hossain and L. Cheng, High-strength wastewater treatment using microbial biofilm reactor: a critical review, World J. Microbiol. Biotechnol., 2020, 36, 75 CrossRef CAS.
- T. Liu, S. Hu and J. Guo, Enhancing mainstream nitrogen removal by employing nitrate/nitrite-dependent anaerobic methane oxidation processes, Crit. Rev. Biotechnol., 2019, 39, 732–745 CrossRef CAS.
- E. Dulekgurgen, S. Dogruel, O. Karahan and D. Orhon, Size distribution of wastewater COD fractions as an index for biodegradability, Water Res., 2006, 40, 273–282 CrossRef CAS.
- P. Zhang, J. S. Guo, Y. Shen, P. Yan, Y. P. Chen, H. Wang, J. X. Yang, F. Fang and C. Li, Microbial communities, extracellular proteomics and polysaccharides: A comparative investigation on biofilm and suspended sludge, Bioresour. Technol., 2015, 190, 21–28 CrossRef CAS.
- W. Wan, D. He and Z. Xue, Removal of nitrogen and phosphorus by heterotrophic nitrification-aerobic denitrification
of a denitrifying phosphorus-accumulating bacterium Enterobacter cloacae HW-15, Ecol. Eng., 2017, 99, 199–208 CrossRef.
- R. Hao, S. Li, J. Li and C. Meng, Denitrification of simulated municipal wastewater treatment plant effluent using a three-dimensional biofilm-electrode reactor: operating performance and bacterial community, Bioresour. Technol., 2013, 143, 178–186 CrossRef CAS.
- S. Xia, J. Li, R. Wang, J. Li and Z. Zhang, Tracking composition and dynamics of nitrification and denitrification microbial community in a biofilm reactor by PCR-DGGE and combining FISH with flow cytometry, Biochem. Eng. J., 2010, 49, 370–378 CrossRef CAS.
- T. Osaka, K. Shirotani, S. Yoshie and S. Tsuneda, Effects of carbon source on denitrification efficiency and microbial community structure in a saline wastewater treatment process, Water Res., 2008, 42, 3709–3718 CrossRef CAS.
- J. C. Leyva-Díaz, A. González-Martínez, M. M. Muñío and J. M. Poyatos, Two-step nitrification in a pure moving bed biofilm reactor-membrane bioreactor for wastewater treatment: nitrifying and denitrifying microbial populations and kinetic modeling, Appl. Microbiol. Biotechnol., 2015, 99, 10333–10343 CrossRef.
- S. Geng, X. C. Pan, R. Mei, Y. N. Wang, J. Q. Sun, X. Y. Liu, Y. Q. Tang and X. L. Wu, Ottowia shaoguanensis sp. nov., isolated from coking wastewater, Curr. Microbiol., 2014, 68, 324–329 CrossRef CAS.
- J. Dolinsek, I. Lagkouvardos, W. Wanek, M. Wagner and H. Daims, Interactions of nitrifying bacteria and heterotrophs: identification of a Micavibrio-like putative predator of Nitrospira spp, Appl. Environ. Microbiol., 2013, 79, 2027–2037 CrossRef CAS.
- I. Naz, D. P. Saroj, S. Mumtaz, N. Ali and S. Ahmed, Assessment of biological trickling filter systems with various packing materials for improved wastewater treatment, Environ. Technol., 2015, 36, 424–434 CrossRef CAS.
- Y. Gao, W. Zhang, B. Gao, W. Jia, A. Miao, L. Xiao and L. Yang, Highly efficient removal of nitrogen and phosphorus in an electrolysis-integrated horizontal subsurface-flow constructed wetland amended with biochar, Water Res., 2018, 139, 301–310 CrossRef CAS.
- M. Seifi and M. H. Fazaelipoor, Modeling simultaneous nitrification and denitrification (SND) in a fluidized bed biofilm reactor, Appl. Math. Model., 2012, 36, 5603–5613 CrossRef.
- P. Rungkitwatananukul, S. Nomai, Y. Hirakata, W. Pungrasmi, C. Puprasert, M. Hatamoto and T. Yamaguchi, Microbial community analysis using MiSeq sequencing in a novel configuration fluidized bed reactor for effective denitrification, Bioresour. Technol., 2016, 221, 677–681 CrossRef CAS.
- D. Garca-Caldern, P. Buffire, R. Moletta and S. Elmaleh, Comparison of three granular support materials for anaerobic fluidized bed systems, Biotechnol. Lett., 1996, 18, 731–736 CrossRef.
- T. Sirinukulwattana, W. Pungrasmi and C. Puprasert, Treatment of low strength wastewater by rubber granules media AFB reactors without internal recirculation, J. Water Sustainability, 2012, 2, 97–106 Search PubMed.
- Y. Tong and Z. He, Nitrate removal from groundwater driven by electricity generation and heterotrophic denitrification in a bioelectrochemical system, J. Hazard. Mater., 2013, 262, 614–619 CrossRef CAS.
- J. Desloover, S. Puig, B. Virdis, P. Clauwaert, P. Boeckx, W. Verstraete and N. Boon, Biocathodic nitrous oxide removal in bioelectrochemical systems, Environ. Sci. Technol., 2011, 45, 10557–10566 CrossRef CAS.
- S. Tong, N. Chen, H. Wang, H. Liu, C. Tao, C. Feng, B. Zhang, C. Hao, J. Pu and J. Zhao, Optimization of C/N and current density in a heterotrophic/biofilm-electrode autotrophic denitrification reactor (HAD-BER), Bioresour. Technol., 2014, 171, 389–395 CrossRef CAS.
- S. Tong, B. Zhang, C. Feng, Y. Zhao, N. Chen, C. Hao, J. Pu and L. Zhao, Characteristics of heterotrophic/biofilm-electrode autotrophic denitrification for nitrate removal from groundwater, Bioresour. Technol., 2013, 148, 121–127 CrossRef CAS.
- J. Li and Z. He, Optimizing the performance of a membrane bio-electrochemical reactor using an anion exchange membrane for wastewater treatment, Environ. Sci.: Water Res. Technol., 2015, 1, 355–362 RSC.
- N. Li, W. Zeng, Y. Yang, B. Wang, Z. Li and Y. Peng, Oxygen mass transfer and post-denitrification in a modified rotating drum biological contactor, Biochem. Eng. J., 2019, 144, 48–56 CrossRef CAS.
- V. Singh and A. K. Mittal, Characterization of biofilm of a rotating biological contactor treating synthetic wastewater, Water Sci. Technol., 2012, 66, 429–437 CrossRef CAS.
- N. Matias, A. H. Nielsen, J. Vollertsen, F. Ferreira and J. S. Matos, Liquid-gas mass transfer at drop structures, Water Sci. Technol., 2017, 75, 2257–2267 CrossRef CAS.
- J. Rodziewicz, A. Mielcarek, W. Janczukowicz, T. Jozwiak, J. Struk-Sokolowska and K. Bryszewski, The share of electrochemical reduction, hydrogenotrophic and heterotrophic denitrification in nitrogen removal in rotating electrobiological contactor (REBC) treating wastewater from soilless cultivation systems, Sci. Total Environ., 2019, 683, 21–28 CrossRef CAS.
- L. Larrea, J. Albizuri, A. Abad, A. Larrea and G. Zalakain, Optimizing and modelling nitrogen removal in a new configuration of the moving-bed biofilm reactor process, Water Sci. Technol., 2007, 55, 317–327 CrossRef CAS.
- F. Polesel, E. Torresi, L. Loreggian, M. E. Casas, M. Christensson, K. Bester and B. G. Plosz, Removal of pharmaceuticals in pre-denitrifying MBBR - Influence of organic substrate availability in single- and three-stage configurations, Water Res., 2017, 123, 408–419 CrossRef CAS.
- C. Sun, T. Leiknes, J. Weitzenböck and B. Thorstensen, Development of a biofilm-MBR for shipboard wastewater treatment: The effect of process configuration, Desalination, 2010, 250, 745–750 CrossRef CAS.
- P. Gkotsis, D. Banti, E. Peleka, A. Zouboulis and P. Samaras, Fouling Issues in Membrane Bioreactors (MBRs) for Wastewater Treatment: Major Mechanisms, Prevention and Control Strategies, Processes, 2014, 2, 795–866 CrossRef.
- C. Glass and J. Silverstein, Denitrification kinetics of high nitrate concentration water: pH effect on inhibition and nitrite accumulation, Water Res., 1998, 32, 831–839 CrossRef CAS.
- Y. Y. Lee, H. Choi and K. S. Cho, Effects of carbon source, C/N ratio, nitrate, temperature, and pH on N2O emission and functional denitrifying genes during heterotrophic denitrification, J. Environ. Sci. Health, Part A: Toxic/Hazard. Subst. Environ. Eng., 2019, 54, 16–29 CrossRef CAS.
- E. Torresi, M. Escola Casas, F. Polesel, B. G. Plosz, M. Christensson and K. Bester, Impact of external carbon dose on the removal of micropollutants using methanol and ethanol in post-denitrifying Moving Bed Biofilm Reactors, Water Res., 2017, 108, 95–105 CrossRef CAS.
- N. Labbe, V. Laurin, P. Juteau, S. Parent and R. Villemur, Microbiological community structure of the biofilm of a methanol-fed, marine denitrification system, and identification of the methanol-utilizing microorganisms, Microb. Ecol., 2007, 53, 621–630 CrossRef CAS.
- H. Lemmer, A. Zaglauer and G. Metzner, Denitrification in a methanol-fed fixed-bed reactor. part 1 physico-chemical and biological characterization, Water Res., 1997, 31, 1897–1902 CrossRef.
- A. Mohanty, A. K. Yadav and G. Roy Chaudhury, Removal of nitrate nitrogen and chemical oxygen demand in upflow fixed bed reactor using heterotrophic microorganisms, J. Cleaner Prod., 2016, 127, 573–578 CrossRef CAS.
- F. Sabba, A. Terada, G. Wells, B. F. Smets and R. Nerenberg, Nitrous oxide emissions from biofilm processes for wastewater treatment, Appl. Microbiol. Biotechnol., 2018, 102, 9815–9829 CrossRef CAS.
- C. Deygout, A. Lesne, F. Campillo and A. Rapaport, Homogenised model linking microscopic and macroscopic dynamics of a biofilm: Application to growth in a plug flow reactor, Ecol. Modell., 2013, 250, 15–24 CrossRef.
- G. Tommaso, R. Ribeiro, S. C. de Pinho, A. L. B. Dibiazi, R. S. S. Santana and T. Z. Penteado, Effect of agitation on the performance of an anaerobic sequencing batch biofilm reactor in the treatment of dairy effluents, Water Sci. Technol., 2011, 63, 995–1003 CrossRef.
- S. Matsumoto, A. Terada and S. Tsuneda, Modeling of membrane-aerated biofilm: Effects of C/N ratio, biofilm thickness and surface loading of oxygen on feasibility of simultaneous nitrification and denitrification, Biochem. Eng. J., 2007, 37, 98–107 CrossRef CAS.
- A. Eldyasti, G. Nakhla and J. Zhu, Influence of biofilm thickness on nitrous oxide (N2O) emissions from denitrifying fluidized bed bioreactors (DFBBRs), J. Biotechnol., 2014, 192(Pt A), 281–290 CrossRef CAS.
- Y. Xiao, S. Wu, Z. H. Yang, Z. J. Wang, C. Z. Yan and F. Zhao, In situ probing the effect of potentials on the microenvironment of heterotrophic denitrification biofilm with microelectrodes, Chemosphere, 2013, 93, 1295–1300 CrossRef CAS.
- F. Sabba, C. Picioreanu and R. Nerenberg, Mechanisms of nitrous oxide (N2O) formation and reduction in denitrifying biofilms, Biotechnol. Bioeng., 2017, 114, 2753–2761 CrossRef CAS.
- D. Todt and P. Dörsch, Mechanism leading to N2O production in wastewater treating biofilm systems, Rev. Environ. Sci. Bio/Technol., 2016, 15, 355–378 CrossRef CAS.
- Y. Pan, B. J. Ni and Z. Yuan, Modeling electron competition among nitrogen oxides reduction and N2O accumulation in denitrification, Environ. Sci. Technol., 2013, 47, 11083–11091 CrossRef CAS.
- S. Szekeres, I. Kiss, M. Kalman and M. I. M. Soares, Microbial population in a hydrogen-dependent denitrification reactor, Water Res., 2002, 36, 4088–4094 CrossRef CAS.
- K. A. Karanasios, I. A. Vasiliadou, S. Pavlou and D. V. Vayenas, Hydrogenotrophic denitrification of potable water: a review, J. Hazard. Mater., 2010, 180, 20–37 CrossRef CAS.
- H. I. Park, J. S. Kim, D. K. Kim, Y.-J. Choi and D. Pak, Nitrate-reducing bacterial community in a biofilm-electrode reactor, Enzyme Microb. Technol., 2006, 39, 453–458 CrossRef CAS.
- A. K. Sahu, T. Conneely, K. Nusslein and S. J. Ergas, Hydrogenotrophic denitrification and perchlorate reduction in ion exchange brines using membrane biofilm reactors, Biotechnol. Bioeng., 2009, 104, 483–491 CrossRef CAS.
- S. W. Van Ginkel, R. Lamendella, W. P. Kovacik Jr, J. W. Santo Domingo and B. E. Rittmann, Microbial community structure during nitrate and perchlorate reduction in ion-exchange brine using the hydrogen-based membrane biofilm reactor (MBfR), Bioresour. Technol., 2010, 101, 3747–3750 CrossRef CAS.
- H. I. Park, Y. J. Choi and D. Pak, Autohydrogenotrophic denitrifying microbial community in a glass beads biofilm reactor, Biotechnol. Lett., 2005, 27, 949–953 CrossRef CAS.
- I. A. Vasiliadou, K. A. Karanasios, S. Pavlou and D. V. Vayenas, Experimental and modelling study of drinking water hydrogenotrophic denitrification in packed-bed reactors, J. Hazard. Mater., 2009, 165, 812–824 CrossRef CAS.
- C. Lu, P. Gu, P. He, G. Zhang and C. Song, Characteristics of hydrogenotrophic denitrification in a combined system of gas-permeable membrane and a biofilm reactor, J. Hazard. Mater., 2009, 168, 1581–1589 CrossRef CAS.
- J. W. Lee, K. H. Lee, K. Y. Park and S. K. Maeng, Hydrogenotrophic denitrification in a packed bed reactor: effects of hydrogen-to-water flow rate ratio, Bioresour. Technol., 2010, 101, 3940–3946 CrossRef CAS.
- S. Szekeres, I. Kiss, T. T. Bejerano, M. Inês and M. Soares, Hydrogen-dependent denitrification in a two-reactor bio-electrochemical system, Water Res., 2001, 35, 715–719 CrossRef CAS.
- N. Sunger and P. Bose, Autotrophic denitrification using hydrogen generated from metallic iron corrosion, Bioresour. Technol., 2009, 100, 4077–4082 CrossRef CAS.
- R. Vagheei, H. Ganjidoust, A.-A. Azimi and B. Ayati, Nitrate removal from drinking water in a packed-bed bioreactor coupled by a methanol-based electrochemical gas generator, Environ. Prog. Sustainable Energy, 2010, 29, 278–285 CrossRef CAS.
- S. Rishell, E. Casey, B. Glennon and G. Hamer, Characteristics of a methanotrophic culture in a membrane-aerated biofilm reactor, Biotechnol. Prog., 2004, 20, 1082–1090 CrossRef CAS.
- B. E. Rittmann and R. Nerenberg, Hydrogen-based, hollow-fiber membrane biofilm reactor for reduction of perchlorate and other oxidized contaminants, Water Sci. Technol., 2004, 49, 223–230 Search PubMed.
- J. Curry and B. W. Glickman, Moloney murine leukemia reverse transcriptase suspect in the production of multiple misincorporations during hprt cDNA synthesis, Mutat. Res., Fundam. Mol. Mech. Mutagen., 1997, 374, 145–148 CrossRef CAS.
- Y. Sakakibara and T. Nakayama, A novel multi-electrode system for electrolytic and biological water treatments, Water Res., 2001, 35, 768–778 CrossRef CAS.
- J. Liessens, J. Vanbrabant, P. De Vos, K. Kersters and W. Verstraete, Mixed culture hydrogenotrophic nitrate reduction in drinking water, Microb. Ecol., 1992, 24, 271–290 CrossRef CAS.
- D. Celmer, J. A. Oleszkiewicz and N. Cicek, Impact of shear force on the biofilm structure and performance of a membrane biofilm reactor for tertiary hydrogen-driven denitrification of municipal wastewater, Water Res., 2008, 42, 3057–3065 CrossRef CAS.
- B. Rezania, N. Cicek and J. A. Oleszkiewicz, Kinetics of hydrogen-dependent denitrification under varying pH and temperature conditions, Biotechnol. Bioeng., 2005, 92, 900–906 CrossRef CAS.
- S. Xia, Z. Zhang, F. Zhong and J. Zhang, High efficiency removal of 2-chlorophenol from drinking water by a hydrogen-based polyvinyl chloride membrane biofilm reactor, J. Hazard. Mater., 2011, 186, 1367–1373 CrossRef CAS.
- B. O. Mansell and E. D. Schroeder, Hydrogenotrophic denitrification in a microporous membrane bioreactor, Water Res., 2002, 36, 4683–4690 CrossRef CAS.
- D. Celmer, J. Oleszkiewicz, N. Cicek and H. Husain, Hydrogen limitation--a method for controlling the performance of membrane biofilm reactor for autotrophic denitrification of wastewater, Water Sci. Technol., 2006, 54, 165–172 CrossRef CAS.
- M. F. Shao, T. Zhang and H. H. Fang, Sulfur-driven autotrophic denitrification: diversity, biochemistry, and engineering applications, Appl. Microbiol. Biotechnol., 2010, 88, 1027–1042 CrossRef CAS.
- B. S. Moraes, T. S. O. Souza and E. Foresti, Effect of sulfide concentration on autotrophic denitrification from nitrate and nitrite in vertical fixed-bed reactors, Process Biochem., 2012, 47, 1395–1401 CrossRef CAS.
- F. Di Capua, F. Pirozzi, P. N. L. Lens and G. Esposito, Electron donors for autotrophic denitrification, Chem. Eng. J., 2019, 362, 922–937 CrossRef CAS.
- M. G. Aylmore and D. M. Muir, Thiosulfate leaching of gold—A review, Miner. Eng., 2001, 14, 135–174 CrossRef CAS.
- J. H. Park, H. S. Shin, I. S. Lee and J. H. Bae, Denitrification of high NO3--N containing wastewater using elemental sulfur; nitrogen loading rate and N2O production, Environ. Technol., 2002, 23, 53–65 CrossRef CAS.
- Y. Wang, C. Bott and R. Nerenberg, Sulfur-based denitrification: Effect of biofilm development on denitrification fluxes, Water Res., 2016, 100, 184–193 CrossRef CAS.
- S. Popiel, T. Nalepa, D. Dzierzak, R. Stankiewicz and Z. Witkiewicz, Effect of temperature and initial dibutyl sulfide concentration in chloroform on its oxidation rate by ozone, J. Hazard. Mater., 2008, 157, 319–327 CrossRef CAS.
- G. Zou, S. Papirio, A. M. Lakaniemi, S. H. Ahoranta and J. A. Puhakka, High rate autotrophic denitrification in fluidized-bed biofilm reactors, Chem. Eng. J., 2016, 284, 1287–1294 CrossRef CAS.
- F. Di Capua, I. Milone, A.-M. Lakaniemi, P. N. L. Lens and G. Esposito, High-rate autotrophic denitrification in a fluidized-bed reactor at psychrophilic temperatures, Chem. Eng. J., 2017, 313, 591–598 CrossRef CAS.
- A. Kostrytsia, S. Papirio, L. Frunzo, M. R. Mattei, E. Porca, G. Collins, P. N. L. Lens and G. Esposito, Elemental sulfur-based autotrophic denitrification and denitritation: microbially catalyzed sulfur hydrolysis and nitrogen conversions, J. Environ. Manage., 2018, 211, 313–322 CrossRef CAS.
- Z. Wang, X. Liu, P. Guo, B. Cao, J. Lin, X. Liang and W. Li, Sulfate reduction and mixotrophic sulfide-utilization denitrification integrated biofilm process for sulfate-laden wastewater treatment and sulfur recovery, Water Sci. Technol., 2015, 71, 1852–1858 CrossRef.
- J. Wang, J. Zhou, Y. Wang, Y. Wen, L. He and Q. He, Efficient nitrogen removal in a modified sequencing batch biofilm reactor treating hypersaline mustard tuber wastewater: The potential multiple pathways and key microorganisms, Water Res., 2020, 177, 115734 CrossRef CAS.
- Y.-X. Cui, G. Guo, B. K. Biswal, G.-H. Chen and D. Wu, Investigation on sulfide-oxidizing autotrophic denitrification in moving-bed biofilm reactors: An innovative approach and mechanism for the process start-up, Int. Biodeterior. Biodegrad., 2019, 140, 90–98 CrossRef CAS.
- H.-R. Kim, I.-S. Lee and J.-H. Bae, Performance of a sulphur-utilizing fluidized bed reactor for post-denitrification, Process Biochem., 2004, 39, 1591–1597 CrossRef CAS.
- W. Yang, Q. Zhao, H. Lu, Z. Ding, L. Meng and G. H. Chen, Sulfide-driven autotrophic denitrification significantly reduces N2O emissions, Water Res., 2016, 90, 176–184 CrossRef CAS.
- Y. Liu, L. Peng, H. H. Ngo, W. Guo, D. Wang, Y. Pan, J. Sun and B. J. Ni, Evaluation of Nitrous Oxide Emission from Sulfide- and Sulfur-Based Autotrophic Denitrification Processes, Environ. Sci. Technol., 2016, 50, 9407–9415 CrossRef CAS.
- P. C. Endler, W. Matzer, C. Reich, T. Reischl, A. M. Hartmann, K. Thieves, A. Pfleger, J. Hofocker, H. Lothaller and W. Scherer-Pongratz, Seasonal variation of the effect of extremely diluted agitated gibberellic acid (10e-30) on wheat stalk growth: a multiresearcher study, Sci. World J., 2011, 11, 1667–1678 CrossRef CAS.
- Y. Liu, H. L. Xu, K. Y. Show and J. H. Tay, Anaerobic granulation technology for wastewater treatment, World J. Microbiol. Biotechnol., 2002, 18, 99–113 CrossRef CAS.
- Y. Pan, B.-J. Ni, P. L. Bond, L. Ye and Z. Yuan, Electron competition among nitrogen oxides reduction during methanol-utilizing denitrification in wastewater treatment, Water Res., 2013, 47, 3273–3281 CrossRef CAS.
-
C. Griesbeck, G. Hauska and M. Schütz, Biological Sulfide Oxidation: Sulfide-Quinone Reductase (SQR), the Primary Reaction, 2000 Search PubMed.
- Z. Gong, S. Liu, F. Yang, H. Bao and K. Furukawa, Characterization of functional microbial community in a membrane-aerated biofilm reactor operated for completely autotrophic nitrogen removal, Bioresour. Technol., 2008, 99, 2749–2756 CrossRef CAS.
- T. Wang, J. Guo, C. Lu, H. Li, Y. Han, Y. Song, Y. Hou and J. Zhang, Faster removal of nitrite than nitrate in sulfur-based autotrophic denitrification coupled with anammox, affected by the anammox effluent, J. Environ. Sci. Water Resour., 2020, 6, 916–924 RSC.
- S. Du, T. Ya, M. Zhang, M. Zhu, N. Li, S. Liu and X. Wang, Distinct microbial communities and their networks in an anammox coupled with sulfur autotrophic/mixotrophic denitrification system, Environ. Pollut., 2020, 262, 114190 CrossRef CAS.
- S. Dasgupta, S. Wu and R. Goel, Coupling autotrophic denitrification with partial nitritation-anammox (PNA) for efficient total inorganic nitrogen removal, Bioresour. Technol., 2017, 243, 700–707 CrossRef CAS.
- E. Sahinkaya, H. Hasar, A. H. Kaksonen and B. E. Rittmann, Performance of a Sulfide-Oxidizing, Sulfur-Producing Membrane Biofilm Reactor Treating Sulfide-Containing Bioreactor Effluent, Environ. Sci. Technol., 2011, 45, 4080–4087 CrossRef CAS.
- Z. He, S. Geng, L. Shen, L. Lou, P. Zheng, X. Xu and B. Hu, The short- and long-term effects of environmental conditions on anaerobic methane oxidation coupled to nitrite reduction, Water Res., 2015, 68, 554–562 CrossRef CAS.
- G. J. Xie, T. Liu, C. Cai, S. Hu and Z. Yuan, Achieving high-level nitrogen removal in mainstream by coupling anammox with denitrifying anaerobic methane oxidation in a membrane biofilm reactor, Water Res., 2018, 131, 196–204 CrossRef CAS.
- M. F. Haroon, S. Hu, Y. Shi, M. Imelfort, J. Keller, P. Hugenholtz, Z. Yuan and G. W. Tyson, Anaerobic oxidation of methane coupled to nitrate reduction in a novel archaeal lineage, Nature, 2013, 500, 567–570 CrossRef CAS.
- M. G. Kalyuzhnaya, S. Yang, O. N. Rozova, N. E. Smalley, J. Clubb, A. Lamb, G. A. Gowda, D. Raftery, Y. Fu, F. Bringel, S. Vuilleumier, D. A. Beck, Y. A. Trotsenko, V. N. Khmelenina and M. E. Lidstrom, Highly efficient methane biocatalysis revealed in a methanotrophic bacterium, Nat. Commun., 2013, 4, 2785 CrossRef CAS.
- F. Y. Sun, W. Y. Dong, M. F. Shao, X. M. Lv, J. Li, L. Y. Peng and H. J. Wang, Aerobic methane oxidation coupled to denitrification in a membrane biofilm reactor: treatment performance and the effect of oxygen ventilation, Bioresour. Technol., 2013, 145, 2–9 CrossRef CAS.
- S. Hu, R. J. Zeng, L. C. Burow, P. Lant, J. Keller and Z. Yuan, Enrichment of denitrifying anaerobic methane oxidizing microorganisms, Environ. Microbiol. Rep., 2009, 1, 377–384 CrossRef CAS.
- Y. Wang, G. Zhu, H. R. Harhangi, B. Zhu, M. S. Jetten, C. Yin and H. J. Op den Camp, Co-occurrence and distribution of nitrite-dependent anaerobic ammonium and methane-oxidizing bacteria in a paddy soil, FEMS Microbiol. Lett., 2012, 336, 79–88 CrossRef CAS.
- M. Strous, J. A. Fuerst, E. H. Kramer, S. Logemann, G. Muyzer, K. T. van de Pas-Schoonen, R. Webb, J. G. Kuenen and M. S. Jetten, Missing lithotroph identified as new planctomycete, Nature, 1999, 400, 446–449 CrossRef CAS.
- C. Cai, S. Hu, X. Chen, B.-J. Ni, J. Pu and Z. Yuan, Effect of methane partial pressure on the performance of a membrane biofilm reactor coupling methane-dependent denitrification and anammox, Sci. Total Environ., 2018, 639, 278–285 CrossRef CAS.
- Y. Shi, S. Hu, J. Lou, P. Lu, J. Keller and Z. Yuan, Nitrogen removal from wastewater by coupling anammox and methane-dependent denitrification in a membrane biofilm reactor, Environ. Sci. Technol., 2013, 47, 11577–11583 CrossRef CAS.
- J. C. Lopez, E. Porca, G. Collins, R. Perez, A. Rodriguez-Alija, R. Munoz and G. Quijano, Biogas-based denitrification in a biotrickling filter: Influence of nitrate concentration and hydrogen sulfide, Biotechnol. Bioeng., 2017, 114, 665–673 CrossRef CAS.
- I. Angelidaki, L. Treu, P. Tsapekos, G. Luo, S. Campanaro, H. Wenzel and P. G. Kougias, Biogas upgrading and utilization: Current status and perspectives, Biotechnol. Adv., 2018, 36, 452–466 CrossRef CAS.
- D. L. Valentine, Adaptations to energy stress dictate the ecology and evolution of the Archaea, Nat. Rev. Microbiol., 2007, 5, 316–323 CrossRef CAS.
- K. Kiskira, S. Papirio, Y. Pechaud, S. Matassa, E. D. van Hullebusch and G. Esposito, Evaluation of Fe(II)-driven autotrophic denitrification in packed-bed reactors at different nitrate loading rates, Process Saf. Environ. Prot., 2020, 142, 317–324 CrossRef CAS.
- J. Zhou, H. Wang, K. Yang, B. Ji, D. Chen, H. Zhang, Y. Sun and J. Tian, Autotrophic denitrification by nitrate-dependent Fe(II) oxidation in a continuous up-flow biofilter, Bioprocess Biosyst. Eng., 2016, 39, 277–284 CrossRef CAS.
- S. Jun Feng, C. Cheng and L. Wei, Assessment of Nitrate Removal Coupled with Fe (II) Oxidation and Analysis of Microbial Communities in a Moving-Bed Biofilm Reactor, Geomicrobiol. J., 2018, 35, 277–283 CrossRef.
- S. Tong, J. L. Stocks, L. C. Rodriguez-Gonzalez, C. Feng and S. J. Ergas, Effect of oyster shell medium and organic substrate on the performance of a particulate pyrite autotrophic denitrification (PPAD) process, Bioresour. Technol., 2017, 244, 296–303 CrossRef CAS.
- Z. Xiao, W. Wang, D. Chen, Y. Yu and H. Huang, pH control of an upflow pyrite-oxidizing denitrifying bioreactor via electrohydrogenesis, Bioresour. Technol., 2019, 281, 41–47 CrossRef CAS.
- Z. Kong, L. Li, C. Feng, S. Dong and N. Chen, Comparative investigation on integrated vertical-flow biofilters applying sulfur-based and pyrite-based autotrophic denitrification for domestic wastewater treatment, Bioresour. Technol., 2016, 211, 125–135 CrossRef CAS.
- V. K. Nguyen, Y. Park, J. Yu and T. Lee, Simultaneous arsenite oxidation and nitrate reduction at the electrodes of bioelectrochemical systems, Environ. Sci. Pollut. Res., 2016, 23, 19978–19988 CrossRef CAS.
- B. Cui, X. Liu, Q. Yang, J. Li, X. Zhou and Y. Peng, Achieving partial denitrification through control of biofilm structure during biofilm growth in denitrifying biofilter, Bioresour. Technol., 2017, 238, 223–231 CrossRef CAS.
- L. Foglar and F. Briški, Wastewater denitrification process—the influence of methanol and kinetic analysis, Process Biochem., 2003, 39, 95–103 CrossRef CAS.
- S.-E. Oh, K.-S. Kim, H.-C. Choi, J. Cho and I. S. Kim, Kinetics and physiological characteristics of autotrophic denitrification by denitrifying sulfur bacteria, Water Sci. Technol., 2000, 42, 959–968 Search PubMed.
- A. Koenig and L. Liu, Autotrophic denitrification of high-salinity wastewater using elemental sulfur: batch tests, Water Environ. Res., 2004, 76, 37–46 CrossRef CAS.
|
This journal is © The Royal Society of Chemistry 2021 |