DOI:
10.1039/D1EN00700A
(Critical Review)
Environ. Sci.: Nano, 2021,
8, 3493-3510
Disinfection applications of ozone micro- and nanobubbles
Received
30th July 2021
, Accepted 2nd November 2021
First published on 8th November 2021
Abstract
Micro- and nanobubbles (MNBs) are microscopic gas bodies sized at micro (<100 μm) and nanoscale (<1 μm), that have a long lifetime in aqueous solutions and large specific surface area due to their small size. Recently, scientific interest has been focused on ozone micro- and nanobubbles (OMNBs) used in disinfection processes since research findings support the idea that ozone micro and nanosized bubbles can significantly improve the disinfection capacity and the residual activity of ozone. The aim of this critical review is to present recent studies which investigate the feasibility of ozone-based disinfection processes by exploiting the strong oxidizing ability of ozone and the noteworthy longevity of MNBs in aqueous solutions. Properties of MNBs and generation techniques are briefly discussed besides the monitoring methods for their characterization in terms of size and number. In this critical review, we provide recent research related to the application of OMNBs in disinfection of drinking water, as well as in aquaculture, agriculture, and wastewater treatment. Finally, research gaps and limitations of this technology are highlighted and directions for future studies are suggested.
Environmental significance
Bacterial contamination and subsequent infections are recognized as a major threat to human health and there is dire need to prevent the waterborne diseases to ensure water safety. Moreover, attention must be paid on the occurrence and fate of trace organic compounds that have become an emerging concern, since conventional wastewater treatment plants (WWTPs) have not been designed for their elimination leading to their discharge to natural water bodies. Within the context of upgrading the water and wastewater treatment processes, the development of new disinfection technologies is addressed, with a view to provide high quality water at the least possible cost to the consumers. By utilizing the higher gaseous ozone half-life time (3 days versus 20 min at 20 °C) and the remarkable properties of ultra fine bubbles, the ozone delivery by MNBs has been found to improve the disinfection capacity and the residual concentration. In this regard, the application of OMNBs technology is paving the way to novel integrated and highly efficient disinfection systems.
|
1. Introduction
Nowadays, the mass production of wastewater derived from increasing population and industrialization is of major of concern since it poses a remarkable threat to existing water resources. Consequently, reclamation and reuse of wastewater are extremely important to meet the human needs arising from inadequate water supplies. However, the core problem of reclaimed water is that it may contain different types of resistant pathogens and persistent organic compounds.1 The microbiological quality plays a crucial role for any potential reuse options, and hence, the presence and persistence of antibiotic-resistant bacteria (ARB) and antibiotic resistance genes (ARG) after tertiary treatment is considered an issue of great importance regarding public health.2–6 In order to prevent the dispersal of ARB, several treatment strategies have been tested and their inactivation efficiency was evaluated,7–9 however, most of these studies have not been conducted in real drinking water and/or wastewater revealing a considerable risk arising from the reduced disinfection ability compared to non-resistant bacteria.10 Emerging organic contaminants (EOCs) consist of a large and relatively new group of compounds covering complex synthetic or naturally occurring molecules or even any microorganism, not commonly monitored in the environment.11 These chemicals compounds are classified as endocrine disrupting chemicals (EDCs), pharmaceutically active compounds (PhACs) and personal care products (PCPs), which even in low concentrations (from ng L−1 to μg L−1) may have detrimental ecological and human health effects.12–14 Moreover, in the last couple of decades, it is well documented that the effluent of WWTPs is the major pathway to aquatic environment,15–19 since they are poorly removed by the conventional activated sludge treatment.20 The emergence of new contaminants in effluent wastewater streams has led to the development of advanced technologies in order to achieve an efficient degradation of these emerging contaminants.21–23
MNBs technology is novel and vitally important owing to the ability to generate highly reactive free radicals.24 In general, microbubbles (MBs) and nanobubbles (NBs) are microscopic gaseous bodies sized with diameters from tens of nanometres to several tens of micrometres. Since the majority of commercially available generators produce gas-carrying bubbles with a diameter within micro- and nano-range, a significant amount of research has been conducted on the use of MNBs technology.25–28
Ozonation is recognized as a favourable treatment method since ozone is an extremely powerful oxidant and is used to inactivate pathogenic microorganisms for the prevention of waterborne diseases spread to users and the environment.29 Furthermore, ozone in aqueous solution auto-decomposes quickly and is converted to oxygen resulting in no harmful residues. However, this is also the main limitation of this method as ozone dissolved in water is unstable and short-lived and hence, the residual action in a drinking water network is very limited. Air MNBs are used to improve gas–liquid contacting and achieve increased effectiveness and enhanced mass transfer compared to conventional aeration including the use of ozone/air mixtures for more efficient ozonation.30,31
The attribute of micro and nanobubbles to ozonation has stimulated widespread interest, and hence, a growing body of literature has investigated the effect of combined micro- and nanobubbles technology and ozonation in many fields of engineering and wastewater treatment.32–35 Despite the considerable progress in academic studies related to MNBs, there are limited comprehensive reviews that focus on the ozonation technology applied to disinfection as shown in Table 1. In this review, we summarize recent research findings regarding the application of ozone micro- and nanobubbles technology on disinfection and thus assist researchers who wish to become involved in this fast-expanding field.
Table 1 Published reviews on the application of ozone micro- and nanobubbles (OMNBs) technology
Title |
Content |
Ref./year |
Applications of Ozone Micro- and Nanobubble Technologies in Water and Wastewater Treatment: Review |
The use of ozone MNBs |
Tekile, Kim and Lee,36 2017 |
• For disinfection in water and wastewater |
• For oxidation of organic and inorganic pollutants |
• For colour removal of water and wastewater treatment |
• To control water pollution in other areas |
The future of ozone MNBs |
Micro and nanobubble technologies as a new horizon for water-treatment techniques: A review |
Applications of MBs and NBs in water-treatment technology |
Temesgen et al.,37 2017 |
• Disinfection with ozone microbubbles (OMBs) |
A Review of Microbubble and its Applications in Ozonation |
Properties and generation of microbubbles; applications in ozone treatment |
Shangguan et al.,38 2018 |
Microbubbles and their application to ozonation in water treatment: A critical review exploring their benefit and future application |
Application of microbubble ozonation for degradation of contaminants |
John et al.,39 2020 |
• Dyestuff |
• Pharmaceuticals |
• Other organic compounds (phenols, heterocyclic organic compounds, nitro aromatic compounds, etc.) |
Challenges and future prospects of microbubble ozonation |
• Microbubble generation methods |
• Reactor configuration |
2. Nanobubbles–microbubbles
2.1 Fundamental properties
According to Temesgen et al.,37 there is no clear definition in terms of diameter size of MNBs. A proposed categorization is that MBs and NBs are in size scale at 10–100 μm and less than a micron, respectively even though in many studies MBs are classified less than 50 μm and NBs less than 200 nm. In this critical review, based on the majority of existing studies, we define MBs less than 100 μm and NBs less than 1 μm, according to Fig. 1.37
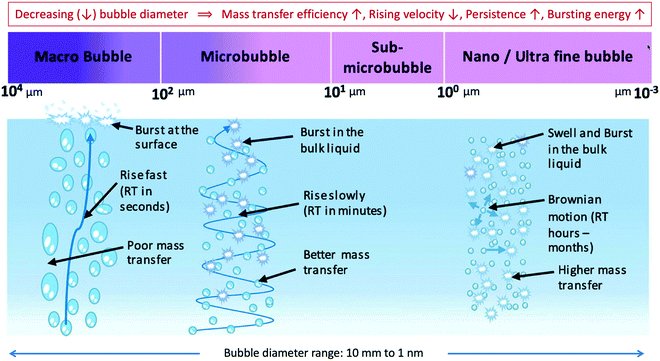 |
| Fig. 1 Range of bubbles sizes and corresponding major properties. Adapted from ref. 37 with permission from Elsevier, copyright 2017. | |
As seen in Fig. 1, bubbles have different properties based on their size. In particular, large bubbles, known as millibubbles or macrobubbles (MaBs) rise rapidly and directly to the liquid surface,40 where they burst out. Compared to ordinary large bubbles, microbubbles have several interesting features such as longevity in aqueous solutions due to low rising velocity, large gas–liquid interfacial area41 and the most important the generation of hydroxyl radicals by their collapse providing an oxidation ability, which makes the dissolution easier.36 So far, a number of researchers have recognized the significance of these properties and they have employed MBs technology in various applications.42–47 In particular, the striking property of MBs, high surface area per unit volume has been used for degradation of organic pollutants and water disinfection.24 Nevertheless, they have been found to be unstable for a long period of time (∼min), rising slowly to the liquid surface.37
Smaller bubbles than MBs, classified as nanobubbles display noteworthy stability resulting in high stagnation times.48,49 NBs can remain stable in aqueous solution for a long period of time (weeks), due to their negligible buoyancy and excellent stability against coalescence.50,51 Considering their unique characteristics, they improve the mass transfer and oxidation ability, simply because the gas/liquid contact area is increased.52 Moreover, the gas solubility and chemical reactions at the gas–liquid interface are remarkably enhanced.48,51
The degree of nanobubbles stability is associated with the absolute value of zeta potential, which is presented in detail in the “Monitoring methods” section. More recent evidence53 highlights that the generation of smaller and more stable nanobubbles is achieved in solutions of high pH, low temperature and low salt concentrations. Another study by Hewage et al. demonstrated the stability of nanobubbles for one week in solutions of different electrolytes at a low concentration (0.001 M), confirming that the neutral and high pH values under low valency cation adsorption leads to negative charged bubbles.54 The highest negative charge of bulk nanobubbles and therefore their stability was also reported in alkaline solutions by Michailidi et al. In the case of oxygen and air nanobubbles, the magnitude of negative zeta potential increases as pH increases.55
Thus far, a number of studies have reported that they have widely applied NBs in water treatment, aquaculture, agricultural cultivation, health preservation, mineral flotation56 and in removing organic pollutants in wastewater treatment.57,58 It is crucial to note that in relevant scientific literature, there is remarkable growth in microbubbles and nanobubbles-related citations and publications over the last 20 years as presented in Fig. 2.59
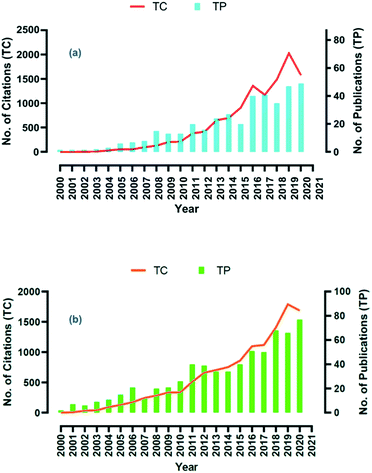 |
| Fig. 2 (a) Annual number of publications for nanobubbles, (b) annual number of publications for microbubbles. Reproduced from ref. 59 with permission from Elsevier, copyright 2021. | |
However, there is still considerable controversy surrounding the existence and the stability of bulk NBs. In order to ascertain that the stable detected nanoentities are gas-filled domains and not impurities or nanodroplets, many analytical experimental techniques have been employed.60–65
Even though, there is considerable discussion in the literature on whether NBs can exist or are thermodynamically stable, it has been demonstrated that the Young–Laplace equation is valid even at nanoscale.66 More precisely, the pressure inside the gas cavities is defined in relation to the diameter of bubbles in accordance to the thermodynamic calculation based on Young–Laplace:
where,
Pin is the internal pressure inside a gaseous bubble (N m
−2),
Pout is the pressure of bulk liquid (N m
−2),
γ is the surface tension (mN m
−1) and
r is the radius of bubbles (nm). It is estimated that a radius of NBs equal to 100 nm can result in an internal pressure 1.5 × 10
6 N m
−2 when the surface tension is 72 mN m
−1 and the atmospheric pressure in the surrounding water is 10
5 N m
−2.
67
Hence, the inner pressure of the bubble increases when the size decreases which is expected to lead to a rapid dissolution and disappearance within seconds. Prior study describes the lifetime (tb) of a bubble according to the following equation:
where,
K is the Henry's law constant (J mol
−1),
do is the bubble diameter at
t = 0 (nm),
R is the gas constant (J K
−1 mol
−1),
T is the temperature (K) and
D is the diffusion constant (m
2 s
−1). For instance, a nanobubble with a diameter 100 nm should exist for only 10 μs.
60,68 Surprisingly, this is not the case with nanosized gas cavities which can stay in aqueous solutions for prolonged periods of time (up to 12 months) compared to larger bubbles.
48
In order to explain the longevity of nanobubbles, Ohgaki et al. proposed that the surfaces of nanobubbles contain strong hydrogen bonds at the gas–liquid interface similar to those found in ice and dehydrated gas. This ameliorates the stability of NBs as it decreases the gas diffusion in liquid, which contributes to kinetic balance against high internal pressure.49 Another possible explanation for the stability of NBs is that it may be dependent on the selective adsorption of anions at the interface that could result in electrostatic repulsive forces, leading to balance the compressive force from surface tension. Hence, a non-contact between gas molecules inside the NBs and the bulk liquid is created due to balance of these forces from the surface tension.69
2.2 Generation methods
In the case of bulk nanobubbles, as mentioned in a recent review by Zhou et al.,56 two main pathways can lead to their formation and generation. The first one is the emergence of the new gas phase from the liquid phase through nucleation and the second through the collapse of microbubbles. The formation, the growth and the collapse of microbubbles in solution can be defined as cavitation and there are four types based on the mode of generation:24,53,69,70
• Hydrodynamic cavitation describes the pressure variation in a moving fluid due to the change in the geometry of the system leading to the occurrence of vaporization and generation of bubbles. In order to enhance the generation of nanobubbles, hydrodynamic cavitation by mechanical agitation, by axial flow shearing and through depressurized flow constriction have been proposed.71
• Acoustic cavitation can be created by applying ultrasonic waves to liquids leading to local pressure variations and subsequently to the formation of bubbles.
• Optical cavitation includes short-pulsed lasers focused into low absorption coefficient solutions.
• Particle cavitation produces nanobubbles by electric discharge or elementary particles in water through passing high intensity light photons in liquids.
Moreover, electrolysis,72 applying nanopore membranes,73 sonochemistry using ultrasound74 and water-solvent mixing64 have been used to form ultra-fine bubbles.
The generation of nanobubbles is influenced by several factors such as pressure, temperature, type and concentration of dissolved gas and electrolyte solution.69 As of today, there are many commercially available nanobubble generators, mostly for laboratory or small pilot applications.67
2.3 Monitoring methods
Several methods have been reported in the literature for the measurement of the size distribution of MNBs.35,56,75,76 The size detection of bubbles has become a crucial issue in classification of ultrafine bubbles due to the fact that it is complex to distinguish the gas bubbles from other colloidal dispersions such as oil nanodroplets or nanoparticles. Undoubtedly, there is a need for the development of techniques with higher level of sensitivity and spatial resolution. Until now, most researchers have utilized mostly dynamic light scattering (DLS) and nanoparticle tracking analysis (NTA), both based on scattering and diffraction of laser on the micro- and nanobubbles.77
Light scattering technique.
The light scattering method is a simple and easy monitoring method based on Tyndall effect.55,78 More precisely, as a light beam passes through a colloid, the light scatters and reflects light, making the beam visible.79 Hence, as the nanobubbles do not rise quickly they can be illuminated by a laser beam and can be viewed with bare eyes while in a clean solution no laser beam can be detected.80 It is an ideal method for simple detection in clear water.
Nanoparticle tracking analysis (NTA).
Nanoparticle tracking analysis offers direct and real-time visualisation of nanoparticles in liquids and size determination within the size range ∼10 to 1000 nm.81 This technique captures the movement of each scattering object with dark field microscopy and their sizes are derived from the analysis of the particles trajectories. It should be highlighted that this technique can also provide adequate information about the particle concentration,82 which is fundamental for the estimation of the micro/nanobubbles generator performance. The main advantage of this technique is that it can record individual particles providing higher resolution and visual information, and thus some kinetic processes can be observed, such as aggregation phenomena.83 However, the main drawback is the analysis of particles with low refractive indices (RI) compared to the background, as it becomes somewhat challenging due to low light scattering intensity.82
Dynamic light scattering (DLS).
Dynamic light scattering is among the most widely used methods to measure the size distribution of micro- and nanobubbles, typically ranging from 0.5 nm to 6 μm. A laser beam illuminates the sample and the fluctuations of the scattered light are detected by a photon detector at a scattering angle θ. The particles follow the Brownian motion, with the larger giving greater scattering but slower fluctuations. Analysis of the intensity fluctuations can provide the particle size distribution.67 The results obtained by light scattering alone may be misleading as a result of the high sensitivity to nano-sized contaminants. Therefore, it is recommended the combination of this technique with acoustic-based flow cytometry in order to ascertain the existence of nanobubbles instead of particles.84 A study conducted by Gnyawali et al. demonstrated that the acoustic flow cytometer can be used in order to detect individual NBs using high-frequency ultrasound and photoacoustic waves since the amplitude of the detected ultrasound backscatter signal is dependent on the NBs size.85
Zeta potential.
Another method of gas bubbles detection that is often used is the measurement of the zeta potential value. Nanobubbles have strong electron affinity and that is identified by a high magnitude of zeta potential ranging from 10 to 50 mV in absolute values. The measurement of zeta potential shows high negative values in most studies verifying that NBs in solution are normally negatively charged.53,86,87 This can be illustrated by the preferential adsorption of hydroxide ions (OH−) at the gas–liquid interface,88 which results in electrostatic repulsive forces leading to balance the compressive force from surface tension. Thus, aggregation and coalescence of NBs are prevented.89
Other methods employed for monitoring of nanobubbles are resonant mass measurement (RMM),90 electron microscopy91 and electrical sensing zone method.92
3. Ozone
Ozone has been applied for primary disinfection in drinking water treatment since the beginning of the 20th century and its use is becoming gradually more common. It is an unstable trioxygen molecule and therefore it must be generated on-site. As it is a very strong oxidant among other commonly used disinfectants (free chlorine, chlorine dioxide and UV light), it provides an excellent inactivation capacity against waterborne pathogens including bacteria, viruses, protozoa and endospores.93 Disinfection parameters such as ozone concentration and contact time are very important for the design of disinfection systems and depend strongly on the operating temperature. Moreover, the rate of inactivation of microorganisms by ozone depends on the type of organism and can vary by about four orders of magnitude. Moreover, other factors that influence the disinfection efficiency are the dissolved organic carbon (DOC), pH and bromide concentration.
3.1 Health risks of ozone
In waters containing significant concentrations of bromide, the required ozone exposures for a certain degree of inactivation may lead to high levels of bromate, which is a carcinogen for humans.94 Thus, in many applications bromate formation may be the limiting factor, and measures have to be taken to comply with the drinking water standard.95 According to a study conducted by Rice et al., in order to meet the requirements for an efficient microbial disinfection in drinking water treatment, the usual ozone dosage is 1.5 to 2 mg L−1, while for viral inactivation, a residual ozone concentration of 0.4 mg L−1 should be detected at least 4 min after the initial ozone dosage.96 Ingestion of drinking water treated by ozone poses no danger since ozone is short-lived and all the concentration present in water will decline to zero when reaching the consumer through the distribution system. However, there is a significant risk though the direct exposure to ozone; inhalation since it is very corrosive. Exposure to ozone at levels below 1 ppm for 10 min is asymptomatic. More severe exposures (1.5 to 2 ppm of ozone for 2 h) produce acute symptoms, such as dryness of mouth and throat, chest pains, coughing etc.96
3.2 Ozone disinfection mechanism
Ozone can react with microbes and contaminants in two different ways, directly and indirectly. Direct reactions involve ozone molecules and are very specific. On the other hand, the indirect reaction involves free hydroxyl radicals (OH˙) produced by the ozone decomposition in water and are more reactive (E° = 2.80 V) and less selective than ozone (E° = 2.07 V). The pH of water is a vital factor in ozone decomposition, because of the fact that hydroxyl ions can initiate the reactions that take place. The direct ozonation dominates when pH < 4, while the indirect pathway prevails above pH 10. In waters with pH = 7, both direct and indirect ozone reactions can be important and they should be taken into account in the process of treatment design.97 The mechanism and kinetics of the basic reactions regarding the ozone decomposition was under investigation by many researchers.98 The interpretation of the processes is based on the following reactions in alkaline medium proposed by Tomiyasu et al.99 In acidic medium, the sequence of reactions taking place are also listed in Table 2.100
No |
Reactions |
Rate constant |
In alkaline medium |
1 |
O3 + OH− → O2 + HO2− |
k
1 = 40 M−1 s−1 |
2 |
O3 + HO2− → O3˙− + HO2 |
k
2 = 2.2 × 106 M−1 s−1 |
3 |
HO2 + OH− ↔ O2− + H2O |
pK = 4.8 |
4 |
O2˙− + O3 → O3˙− + O2 |
k
4 = 1.6 × 109 M−1 s−1 |
5 |
O3˙− + H2O ↔ HO˙ + O2 + OH− |
k
5 = 20–30 s−1 |
6 |
O3˙− + HO˙ → O2− + HO2 |
k
6 = 6 × 109 M−1 s−1 |
7 |
O3˙− + HO˙ → O3 + OH− |
k
7 = 2.5 × 109 M−1 s−1 |
8 |
O3 + HO˙ → HO2 + O2 |
k
8 = 3 × 109 M−1 s−1 |
In acidic medium |
9 |
O3 ↔ O + O2 |
|
10 |
O + H2O → 2HO˙ |
|
11 |
HO˙ + O3 → HO2˙ + O2 |
k
11 = 1.1 × 108 M−1 s−1 |
12 |
HO2˙+ O3 → HO˙ + 2O2 |
k
12 < 104 M−1 s−1 |
An important reaction is the first one in Table 2, where ozone reacts with OH− and hence, it is greatly dependent on pH. At alkaline pH, eqn (5) describes the generation of HO˙. Higher concentration of hydroxyl ions leads to the increased generation of HO2−, O2−, O3− and HO˙. At 7 < pH < 9, the generation of hydroxyl radicals is slow corresponding to the rate constant of the reaction (5) (20–30 s−1). The propagation and termination reactions [i.e., those given by eqn (6)–(8)] are very fast, leading to a lower concentration of HO˙.101
However, in acidic medium, a different mechanism is involved, as the reaction with OH− cannot be the initiation step. According to Sehested et al.,100 it is proposed the thermal dissociation of ozone to form an oxygen atom, which is followed by the reaction of this atom with water to form the hydroxyl radical [i.e., those given by eqn (9) and (10)]. Then, the hydroxyl radical reacts with ozone to form the perhydroxyl radical (HO2˙).
3.3 Ozone interaction with microorganisms
Ozone even in low concentrations (0.01 ppm) is effective against bacteria due to its high oxidation potential. There is limited information in the literature concerning the inactivation mechanisms of microorganisms by ozone. The bactericidal efficiency lies on the fact that there are many ozone reactions with chemicals of high biological importance. First of all, it is suggested that ozone attacks the glycoproteins and glycolipids in the cell membrane resulting in rupture of the cell. In addition, another bactericidal activity is the oxidation of the sulfhydryl groups of certain enzymes which results in disruption of cellular enzymatic activity and loss of function. Moreover, ozone attacks the purine and pyrimidine bases of nucleic acids leading to DNA damage.102
The proposed mechanism for the inactivation of E. coli proceeds in the following order of viability indicators:29
I. Direct oxidation/destruction of the cell wall with leakage of cellular constituents outside of the cell.
II. Reactions with radical by-products of ozone decomposition entering the cell.
III. Damage to constituents of the nucleic acids (purines and pyrimidines).
IV. Breakage of carbon–nitrogen bonds leading to depolymerization and to cell wall disintegration causing cell lysis.
The antimicrobial capacity of ozone includes not only bacteria, but also molds, viruses, and protozoa. Ozone can react with numerous organic compounds and generate radical species such as hydroxyl radical that have more oxidative potential. Both HO2˙ and the HO˙ radicals are highly reactive and play a fundamental role in the disinfection process. After the direct protoplasmic oxidation of bacteria, the free radicals produced react with the nucleic acids and provoke a sufficient damage, and incontrovertibly achieve inactivation.103
3.4 Properties of ozone micro- and nanobubbles
One factor credited for the stability of MNBs in aqueous solutions is their zeta potential. High zeta potential values prevent the bubbles from coalescence by increasing the repulsive electrostatic forces.50 In the case of OMNBs, the long term stability has a strong effect on dissolved ozone concentration and consequently on enhanced disinfection efficiency. It should be noted that small diameter with high specific area and low rising velocity increases the mass transfer rate and the ozone reactivity to target contaminants.104 The main factors that have a great impact on OMNBs are the following:
Temperature.
The temperature is considered a crucial factor that can influence the stability of OMNBs. A recent study by Hewage et al. investigated the effect of temperature on the size of ozone nanobubbles and the zeta potential. They reported elevated temperatures resulted in an increase of diameter and a decrease of the zeta potential. The size was in the range of 100–300 nm, and the negative zeta potential values were within the range of −25 to −14 mV. To elucidate the fact that temperature is inversely proportion to zeta potential, the adsorbed ions at the gas–liquid interface should be taken into account since in high temperature they decrease owing to higher mobility.105
pH.
The aforementioned studies have emphasized the strong impact of solution pH on zeta potential and specifically suggested that NBs produced in water at a high pH value exhibit small diameter and high zeta potential.53 In the case of OMNBs, the same trend was confirmed by another study where they investigated the values of zeta potential over a range of pH conditions.106 It was reported that the zeta potential value increased in absolute values as the pH values increased. Specifically, at pH = 2, 4.5, 7.5 and 8, zeta potential values were found to be 9.92, 2.35, −32.34, −37.55, respectively.106 Another research study produced similar results. The zeta potential of OMBs in deionized water was approximately −33 mV at pH = 8 and above −20 at pH = 7.107 Hence, it is clear from these results that at high pH the stability of OMNBs is greater, mainly due to increased adsorbed OH− ions at the interface. However, since ozone decomposes more quickly at high pH,108 in order to achieve the same levels of ORP, a greater amount of bubbles is required at higher pH.109
Salt concentration.
The generation of ozone nanobubbles under various salt concentrations (0.01, 0.1 and 1 M) showed that increasing sodium chloride (NaCl) concentration resulted in a decrease in the magnitude of zeta potential with a slight increase in diameter.53 It is noted that the values of the zeta potential were negative in all cases. Another experiment focused on the effect of salinity on the stability of OMNBs in terms of zeta potential and size distribution showed that OMNBs are stable under various salinity levels, since they remained negatively charged. Specifically, the salinity caused a reduction in negative zeta potential when no obvious effect on the diameter of OMNBs was observed.30
Hydroxyl radicals.
Hydroxyl radicals exhibit microbicidal activity, and as such, their generation should be taken into consideration in order to provide some insight into the observed disinfection efficiency. Takahashi et al. reported that the generation of free radicals occurs by the micro- and nanobubbles collapse thanks to the high density of ions in the gas–liquid interface and they concluded that ozone microbubbles generate hydroxyl radicals under strong acidic conditions.110 Several studies, for instance,106,107,111 have proven that hydroxyl radicals existed in water containing ozone microbubbles using fluorescence intensity. It is noted that the capacity for generating free radicals is of high importance as hydroxyl radicals are strong oxidants and not selective, and thus the oxidation processes can be accelerated.106
3.5 Ozone dissolution with micro- and nanobubbles
Even though conventional ozonation is widely used for ozone dissolution in aqueous phase, the main drawback is the high amount of escaping ozone gas resulting in a high level of gas consumption. When microbubbles is used for ozonation, the degradation of trace organic compounds were found to be efficiently enhanced since the solubility of ozone in water is increased.112 Several research studies suggest an association between bubble size diameter and the enhancement of ozone solubilization rate in the aqueous phase. Table 3 lists a number of existing studies, which have examined the comparison of ozone dissolution between macro and MNBs. All the available information about the experimental conditions is provided. A notable increase in peak value of dissolved ozone concentration was reported more recently by Hu and Xia,30 as the ozone level for OMNBs was 10.09 mg L−1 compared to macrobubbles which provided a very low ozone value (0.64 mg L−1) within a generation time 30 min. Kobayashi et al.113 noted that aqueous dissolved ozone concentration is higher when the water is treated with microbubbles compared to macrobubbles. In 5 min ozonation with microbubbles, the concentration of ozone reached 1.58, 1.24 and 0.82 ppm at 15 °C, 25 °C and 30 °C, respectively. On the other hand, when macrobubble ozonation was applied, the concentration was found 3-fold and 4-fold lower at 15 °C and 25 °C, respectively and no ozone was detected at the highest temperature. Another comparison of ozone microbubbles and normal bubbles demonstrated that the dissolved ozone concentration was approximately 2.5 times higher than that obtained by ordinary bubbling.114 More recent evidence115 showed that ozone dissolution using micro- and nanobubbles was approximately 50% higher after 5 min-aeration compared to a classical mixing pump with larger bubbles. The findings of another study confirm the observation that bubbles with smaller diameter can enhance the dissolution of gaseous ozone into the aqueous phase.116 In fact, the concentration of dissolved ozone by the regular method of ozone delivery was found to be 0.5 mg L−1 at 20 °C, when microbubble ozonation could reach the value of 1.67 mg L−1 in the presence of para-chlorobenzoic acid (pCBA).116 An increase in ozone concentration with nanobubbles was also reported in the study conducted by Batagoda et al.,104 where the initial dissolved ozone concentration was 52.79 mg L−1, higher than 48.28 mg L−1 found with ozone macrobubbles. Fan et al.117 illustrated that the concentration of dissolved ozone after MNBs aeration was 3.54 mg L−1 in 25 min while after the millibubbles ozonation the ozone reached only 1.74 mg L−1 in 30 min. The most striking result to emerge from this study is that the ozone solubility was calculated about 4 times higher in 5% acetic acid solutions after OMNBs aeration reaching the ozone value of 15.26 mg L−1. It is well documented that acetic acid is considered an ozone stabilizer due to non-reactivity with it and thus, it can be beneficial to the ozonation process.117 Further confirmation is given by another research study,118 where the saturated ozone concentration with microbubble ozonation reached the value of 9.6 mg L−1 within 7 min and was found to be enhanced since the macrobubble ozonation achieved a lower dissolved ozone concentration at longer time period. This can be elucidated by the fact that ozone mass-transfer coefficient was 2.2 times higher than that of the conventional ozonation process.118 Research findings from two other studies corroborate with the previous result as the augmentation of total mass transfer in microbubbles ozonation was also proved for simulated dyestuff wastewater treatment (1.8 times higher) and for landfill leachate pre-treatment (1.5 times higher).111,119 Similar results were reported by Wang, Lin and Liao,120 a team which recently explored experimentally the raise in ozone dissolved concentration, when ultrafine bubbles are used. Within 10 min, the maximum dissolved ozone concentration reached the value of 8.3 and 3.5 mg L−1, during ozonation with MNBs and MaBs, respectively. The most recent evidence confirms once more the higher dissolved ozone concentration of 4 mg L−1 in microbubbles ozonation instead of 2.49 mg L−1.121 In three test fluids, pure water, tap water and phosphate buffered saline, the ozone dissolution velocity (mg L−1 min−1) was found higher by 1.5, 1.6 and 2.7 times when ozone injected by microbubble generator instead of porous diffuser.122 In the course of the ozonation of synthetic semi-conductor wastewater containing tetramethyl ammonium hydroxide within 7 min, the gas transfer to water by nanobubbles (1.67 mg L−1 min−1) was 9.8 times faster than that of macro-ozone (0.17 mg L−1 min−1).123 Consistently, the ozone dissolution was found once again 1.5 times higher by ozone microbubbles injection in tap water.124 Finally, a group of researchers in 2021 has investigated the ozone mass transfer coefficient with nanobubble aeration and compared it with macrobubble aeration. Their findings are in line with all the previous results. In fact the volumetric mass transfer coefficient (KLa) was estimated 0.179 min−1, reaching the peak value of ozone concentration of 13.4 mg L−1, while in macrobubble aeration the volumetric mass transfer coefficient was 4.7 times lower (0.038 min−1) with a dissolved ozone concentration up to 7.9 mg L−1.125 These findings demonstrate the strong effect of MNBs to ozone solubilization. In general, it can be concluded that the use of MNBs in ozonation leads to a more efficient process as the ozone utilization efficiency is higher.
Table 3 Comparison of ozone dissolution between ozone macrobubbles (OMaBs) and ozone micro- and nanobubbles (OMNBs)
Ref. |
Size |
Flow rate (L min−1) |
Ozone conc. (mg L−1) or rate (g h−1) |
Time (min) |
Volume (L) |
Temp. (°C)/pH |
Type of water |
Peak concentration (mg L−1) OMaBs vs. OMNBs |
30
|
Micro/nano (32–460 nm, 4.55 × 107 bubbles per mL) |
4 L min−1 |
50 mg L−1 |
30 |
20 |
20 |
Deionized |
0.64 vs. 10.09 |
113
|
Micro (<50 μm) |
2.5 L min−1 |
|
5 |
10 |
15 |
De-chlorinated |
3-fold lower-1.58 ppm |
25 |
4-fold lower-1.24 ppm |
30 |
No ozone detected in OMaBs vs. 0.82 ppm in OMBs |
114
|
Micro (peak at 15 μm) |
1 L min−1 |
50 mg L−1 |
30 |
5 |
Ambient |
Distilled |
2.5 fold higher than OMaBs |
115
|
Micro/nano |
|
25 g h−1 |
5 |
20 |
20 ± 1/6 |
Distilled |
5.5 vs. 8.3 |
116
|
Micro (5–25 μM = 50%) |
|
0.61–0.72 g h−1 |
5 |
20 |
10/7 |
Ultrapure |
0.65 vs. 2.16 |
20/7 |
0.50 vs. 1.67 |
30/7 |
0.40 vs. 1.32 |
104
|
Nano |
|
|
3 |
20 |
20/7 |
|
48.28 vs. 52.79 |
117
|
Micro/nano (3.38 μm, 2.41 × 105 bubbles per mL) |
0.5 L min−1 |
11 mg L−1 |
30 |
20 |
17.4 ± 1.2 |
Distilled |
1.74 vs. 3.91 |
118
|
Micro (<45 μm, 3.9 × 105 counts per mL) |
0.5 L min−1 |
5 g h−1 |
14 |
3 |
20/8 |
Wastewater from acrylic fiber manufacturing industry |
8.4 vs. 9.6 |
111
|
Micro (<58 μm, 2.9 × 104 counts per ml) |
0.5 L min−1 |
|
10 |
20 |
18 ± 2 |
Deionized |
∼8 vs. 13 |
119
|
Micro |
0.2 L min−1 |
36 mg L−1 |
40 |
8 |
20 |
Tap |
∼4 vs. 11 |
120
|
Ultra-fine (0.5–3 μm) |
30 mL min−1 |
|
10 |
1 |
25 |
Distilled |
3.5 vs. 8.3 |
121
|
Micro |
|
3–4 mg L−1 |
12 |
80 |
|
Secondary treated sewage water |
2.49 vs. 4.00 |
123
|
Nano (133.7 nm, 5.25 × 109 particles per mL) |
|
|
7 |
|
25/7 |
Synthetic semi-conductor wastewater containing TMAH |
∼1 vs. 12 |
124
|
Micro |
|
|
5 |
20 |
|
Tap |
3.5 vs. 5.3 (reached in 2 min) |
125
|
Nano (580 nm, 2.16 × 105 particles per mL) |
0.5 L min−1 |
38 mg L−1 |
30 |
|
|
|
7.9 vs. 13.4 |
3.6 Ozone decomposition rate
The half-life time of ozone in gas phase is much higher than in aqueous phase. In more detail, at 20 °C the gaseous ozone will be degraded in 3 days, in contrast the degradation of dissolved ozone in water will take place within only 20 minutes.126,127 Due to its low utilization efficiency, nanobubbles technology is gradually used for ozone application in a more efficient way. However, there are very limited research studies that investigated the comparison of the half-life times between OMNBs and macrobubbles owing to the fact that the academic interest is focused on the study of ozone solubility and mass-transfer. Hu and Xia30 have also investigated the half-life time of dissolved ozone with and without the use of MNBs and their results demonstrated that the average lifespan for the MNB system was 10.51 min, whereas that for macrobubbles system was only 0.70 min for 30 minutes generation time. A 2007 research study observed that a longer half-life was found when a microbubble generator injected ozone in tap water instead of a porous diffuser (1.6 times longer at 19.2 °C).122 The lifespan when ozone delivered through nanobubbles in water was greater than conventional ozone bubbles. In fact, ozone is retained in water approximately four time longer than using a sandstone diffuser.104 In another research study,117 ozone decomposition was investigated when OMNBs were present in various concentrations of acetic acid and in water alone. In this case, the results showed that the average half-lives of ozone were longer by 1.39, 2.04 and 3.52 times in 0.5, 3 and 5% acetic acid solutions, respectively. The evidence from this study points towards the idea that acetic acid can further enhance the longevity of ozone in water apart from MNBs.117 Remarkably in a very recent study, it was shown that half-life of ozone generated by nanobubbles was found to be 23 times higher than that of macro-ozone.123 The ozone lifespan was investigated in nanobubble and macrobubble aeration groups and was found to be 3.50 h and 1.75 h in the latter. In the presence of hydroxypropyl-β-cyclodextrin (HPβCD), which is used as an ozone stabilizer, the ozone half-life time were 2.8, 4.3, 9.3 and 2.2 times higher than those estimated from the macrobubbles aeration under different HPβCD
:
O3 molar ratios (1
:
1, 3
:
1, 5
:
1 and 10
:
1, respectively).125 The results so far confirmed that the utilization of MNBs can extend the ozone half-life. Moreover, it can be concluded that the addition of an ozone stabilizer can further intensify the ozone lifespan and can be utilized to strengthen the ozonation process.
4. Application of ozone-based macro- and nanobubble technology in disinfection
4.1 Antimicrobial and disinfection process
Bacterial contamination and subsequent infections are recognized as being a major threat to human health and there is an urgent need to inactivate pathogenic organisms and prevent the waterborne diseases spread to users and the environment. In this regard, the development of novel technologies based on the application of OMNBs is of paramount importance.
Furuichi et al.128 reported that ONBs water deactivates both Gram-positive and Gram-negative bacteria while this approach does not show any cytotoxicity against human gingival fibroblasts, unlike conventional mouth wash. Dissolved ozone concentration of 1.5 mg L−1 provided a sufficient bactericidal activity for periodontal pathogens. Specifically, the inactivation of the bacterial cells (S. aureus-2.4 × 108 CFU mL−1, S. sanguinis-1.5 × 108, K. pneumoniae-7.6 × 108 CFU mL−1 and E. coli-1.6 × 109 CFU mL−1) was >99.99% since the viable bacteria were below detection limit (<10 CFU mL−1). For P. gingivalis cells with initial bacterial concentration 7.0 × 107 CFU mL−1, the percentage of killed bacteria was higher than 99.99%, while the disinfection activity was deteriorated in case of S. mutans with initial bacterial concentration 1.7 × 106 CFU mL−1, since it reached a maximum disinfection of 94.69% within three minutes.128 Another study for the evaluation of the bactericidal activity against periodontal pathogenic bacteria (P. gingivalis and A. actinomycetemcomitans) reported that ONBs water with concentration 1.5 mg L−1 was capable to reduce the numbers of colony forming units (CFU mL−1) below the limit of detection (<10 CFU mL−1) after only 0.5 min of exposure, providing evidence that it is not cytotoxic to cells of human oral tissues.129
As it is mentioned before, ozone is highly unstable and this is a problem posed in terms of stocking ozone aqueous solutions. This issue was explored by Seki et al.130 implementing ONBs technology for the storage of ozone. It was found that such an approach produces good efficiency in storage as the microbicidal activity was adequate for different set time periods. ONBs stored at 4 °C retained more than 90% of ozone after a week and more than 65% after a month. Moreover, the residual concentration of ozone stored at 4 °C for 1 year was adequate to kill one of the most resistant bacteria, M. smegmatis, within 15 min; even though E. coli was not entirely killed even after a 60 min exposure.130
4.2 Drinking water disinfection
A common strategy used to ensure safety in drinking water is ozonation. The rapid decomposition of ozone in water and the low residual concentration are the main drawbacks of this process. Utilizing NBs serves as a more efficient alternative to drinking water disinfection as the decomposition of ozone in water is decelerated and the ozone dosage required against contaminants or pathogens is reduced thanks to a greater dissolution. Sumikura et al. found that the ozone dose was lower when OMBs were used instead of the conventional ozonation with macrobubbles providing the same inactivation rate of target pathogen E. coli.122
One of the most crucial parameters of conventional ozonation is the cost effectiveness of installation. A recently conducted cost–benefit analysis indicated that the installation of a ONBs generator is beneficial for existing water treatment plants as the total cost would be four times less and could save 375k$ per year.104
Another important parameter is the effect of inlet ozone gas concentration on the removal rate. This issue has been investigated on the log reduction of B. subtilis by microbubble ozonation and the results showed that higher gaseous ozone concentration led to higher disinfection efficiency after 2 min of operation (reduction by 5
log for 140 mg L−1 O3 in gas phase, compared to 1.6
log and 0.3
log for 110 mg L−1 and 40 mg L−1, respectively). This can be justified by the fact that the size of bubbles in higher ozone inlet was found to be smaller inducing higher volumetric mass transfer coefficient (KLa) and consequently an increased utilization efficiency. It was also found that the KLa had almost been doubled from inlet gas concentration 40 mg L−1 to 140 mg L−1, while the Sauter mean diameter was decreased from 75.7 μm to 49.7 μm, respectively.131 Combination of ozonation and hydrodynamic cavitation showed the best performance in disinfection of E. coli with an initial bacterial concentration of approximately 105 CFU mL−1 was decreased to zero within 45 min whereas for the same ozone concentration using only ozonation without cavitation, the bacterial concentration reached zero after 60 minutes.132
Summarizing, the higher mass transfer leading to lower ozone dosage renders the use of OMNBs a promising and an efficient technology in terms of cost and disinfecting capacity.
4.3 Disinfection of wastewater treatment plant effluents
Apart from the importance of disinfection, attention must also be paid on the occurrence and fate of trace organic compounds that are considered of emerging concern. It is of major importance to eliminate these pollutants as they can be discharged to water bodies and induce adverse and undesirable effects onto humans, living organisms and environment even at low concentrations.133 As demonstrated in literature, the ozone amounts required for PPCPs oxidation may lead to a partial disinfection, hence it is crucial to highlight the influence of emerging contaminants existence on the ozone disinfection capacity.134,135
In aspect of wastewater treatment, an analysis on deactivation of faecal and total coliforms in domestic waste water in Peru indicated that through applying air–ozone micro–nanobubbles, it was obtained 99.58% for faecal coliforms and 99.01% for total coliforms.136 Lee et al. investigated the degradation of pharmaceuticals compounds by a microbubble ozonation process and showed that it was markedly enhanced by the decrease in diameter of the ozone bubbles. It was found that the residual concentrations (C/C0) of the selected pharmaceuticals compounds, including 17α-ethinylestradiol (EE2), ibuprofen (IBU) and atenolol (ATE) was estimated (at 20 °C) 0.61, 0.75 and 0.77, respectively, when treated with microbubbles and differ significantly from ozone millibubbles treatment, where the residual concentrations were found to be 0.79, 0.88 and 0.87.116 Another investigation on the degradation of 39 pharmaceuticals in water showed that the introduction of microbubble ozonation improved significantly the removal rate by 8–34%.137 Concerning the degradation of tetracycline, the removal was found 50% and 95% with millibubble and ultrafine bubbles ozonation, respectively, within 20 min, indicating the enhanced degradation of the antibiotic when lowering the bubble size. The same study concluded that the most-favourable degradation and mineralization of the target persistent pollutant was achieved when ultrafine bubbles ozonation was performed at lower pH levels and higher reaction temperature.120 In another research study, the degradation of 26 PPCPs was examined and the average elimination was found to be 53% and 63.9% in macrobubbles and microbubble ozonation at low concentration, respectively.121
In this section, the degradation behaviour of target organic compounds by OMNBs was explored in tertiary treatment of wastewater and it was found that their application provides a better performance compared to conventional ozonation and as a result OMNBs can minimize the discharge of emerging contaminants into water bodies.
4.4 Aquaculture
Fisheries and aquaculture are a growing industry and seafood consumption has reached 20.3 kg per capita in 2017.138 Additionally, seafood remains at the top level of the global market as in 2018, 88% of total fishery and aquaculture production was used for direct human production.138 Seafood contamination is associated with a number of pathogenic microorganisms and has become a key challenge regarding the food safety. In this regard, effective pathogen intervention strategies have been applied.139
Several studies have been recently conducted in order to explore the effect of ozone nanobubbles (ONBs) in aquaculture against aquatic pathogens (Table 4). Specifically, Jhunkeaw et al.140 have investigated the disinfection efficiency against Streptococcus agalactiae and Aeromonas veronii in fresh water which are considered pathogenic fish bacteria. Three consecutives ozone treatments (10 min exposure at ONBs at 15 min intervals) were tested. The first 10 min treatment reduced the bacterial load of S. agalactiae and A. veronii 26 and 48 fold or 96.11% and 97.92%, respectively. The next two 10 min ONBs treatment reduced further the bacteria load in water reaching higher than 99.9% reduction for both pathogenic bacteria. In water taken from a Nile tilapia-cultured tank (initial bacterial concentration: 8.18 × 105 CFU mL−1) with the presence of organic matter the disinfection efficacy of ozone nanobubbles was reduced and reached the 59.63% after the first treatment and the other two treatments were required to reach the 99.29%. The loss in the disinfection capacity can be illustrated by the fact that the presence of the organic matter led to the rapid ozone oxidation and degradation.140
Table 4 Applications of ozone micro- and nanobubbles (OMNBs) in aquaculture
Target microorganism |
Bacterial conc. (CFU mL−1) |
Type of water |
ORP |
Time (min) |
Disinfection efficiency with NBs |
Ref. |
S. agalactiae
|
3.45 × 106 |
Dechlorinated tap water |
834 ± 22 mV |
10 |
96.11% |
140
|
A. veronii
|
1.65 × 106 |
97.92% |
V. parahaemolyticus
|
106 |
15‰ saline |
830 ± 70 mV |
6 |
100% |
141
|
V. parahaemolyticus
|
1.8 × 105 |
Artificial sea water |
960 mV (∼3.5 mg O3 per L) |
5 |
100% |
143
|
In another research study, the disinfection of Vibrio parahaemolyticus at a concentration 106 CFU mL−1 in 15‰ saline water was studied. At the end of the experiment, the bacterial concentration (CFU mL−1) was estimated 2.3 × 101, 2.2 × 100 and 0 CFU mL−1 for 2-, 4- and 6-minute ONBs exposure, respectively. The results of the oxidation–reduction potential (ORP) showed that the initial ORP value, which was 240 mV rose to 830 ± 70 mV after six minutes operation and remained stable at over 900 mV as the nanobubbles generator continued working for ten more minutes.141
Thanh Dien et al. reported that even though, the bacterial concentration was high (∼2 × 107 CFU mL−1), multiple ONBs treatments in the first two days reduced the bacteria between 15.9% and 35.6% of total bacterial load in water, while bacterial concentration increased from 13.1% to 27.9% in the untreated control.142 ONBs sea water at 960 mV ORP was used to carry out disinfection experiments against V. parahaemolyticus EMS/AHPND strain. From these results it is clear that ONBs treatment provide a high disinfection efficiency, since after 1 min incubation over 99.99% of tested bacteria were killed and after 5 min or longer incubation the sterilization efficiency was 100%.143
Apart from the ozone disinfection efficiency, Kurita in 2017 demonstrated the killing effect of cavitation treatment on small planktonic crustaceans that can cause detrimental problems in invertebrate aquaculture tanks through predatory damage or competition for food resources with the aquaculture species.144 The results showed that micro and nanobubbles reduced the planktonic crustaceans in the aquaculture tanks by 63.3% compared with the control by killing crustaceans of all sizes equally.
Effect of ozone nanobubbles on fish health.
Ozone has found its greatest use as disinfectant in closed recirculating aquaculture systems in order to reduce the pathogenic bacteria and prevent any fish disease.145 The residual ozone concentration is of high importance since it has been found that concentrations within the range 0.01–0.1 mg L−1 can be highly toxic to fish in fresh- and seawater. There is significant difference between the ozone reaction with saline and freshwater in terms of disinfection. The presence of bromide ion (Br−) in seawater results in the formation of brominated compounds like bromate (BrO3−) by ozone oxidation, which is toxic to aquatic organisms.146 On the other hand, in fresh water ozone decomposes to oxygen elevating the levels of dissolved oxygen in the system, which may also have detrimental effects on fish if it is very high.146 In terms of ORP, several studies suggest that the levels in the range from 300 to 425 mV can ensure the safety of fish, crustaceans and molluscs.142 Summarizing, in order to apply a safe ozone disinfection system, the lethal limits, which depend on the cultured species and the type of water, have to be determined and not exceeded during operation.
A study from Jhunkeaw et al. suggested that a single 10 min exposure to ONBs with an ozone level 860 ± 42 mV is safe for Nile tilapia in fresh water. Even though no mortality was observed after receiving the second and the third consecutive ONBs treatments, the increased exposure caused damage in the gill filaments.140 However in another study, they set up a modified recirculation system to reduce direct exposure to the fish, in order to avoid any alterations in exposed fish. In this case, juvenile Nile tilapia did not exhibit any abnormalities in behaviour or mortality by the application of multiple ONBs treatments.142 ONBs in seawater containing ozone dose at 3.5 mg L−1 and 960 mV ORP was proven to be toxic to shrimp, therefore a twofold dilution of ozonated seawater was suggested as shrimp survival and excellent inactivation activity was observed.143 An additional study in the literature regarding the exposure of Nile tilapia (Oreochromis niloticus) to ozone nanobubbles noted that innate immunity genes involved in the systematic frontline defence system were stimulated. In all examined organs, these genes expressed an upregulation very fast within 15 min – post ozone nanobubbles treatment and lasted from 12 to 24 h in the gills, the head kidney and the spleen. It was thus concluded that based on the efficient stimulation of the genes by ONBs treatment, a protection to cultivated animals from potential pathogenic infections can be provided.147 In addition, any possible negative effect of the ultrafine bubbles in cavitation treatment on two juvenile sea cucumbers (Apostichopus japonicus) and sea urchins (Strongylocentrotus intermedius) was evaluated and it was found that all individuals were intact and uninjured four days after exposure to ozone nanobubbles.144
Experimental results provide a basis for the application of ozone nanobubbles in aquaculture since it is efficient for reducing pathogenic bacteria (Table 4). Future studies should aim to replicate results in a larger scale and further explore the efficiency to prevent disease outbreaks. The safety of using ONBs is a core issue and should be investigated in more detail in order to gain a better understanding of the toxicity to fish, which depends upon species and the life stage.
4.5 Agriculture
The effect of ozone ultra-fine bubbles on washing fresh vegetables was tested and when acidic electrolyzed water containing ozone ultra-fine bubbles and strong mechanical action combined, the lowest viable bacterial count was recorded among other treatments including sodium hypochlorite.148 The disinfection efficiency of F. oxysporum f. sp. melonis spores was tested and the results confirmed that ozone microbubbles exhibited higher disinfection efficiency than macrobubbles (Table 5). In addition, spores treated with OMBs showed surface injury after 30 s and wavy deformation of cell membrane was observed after 180 s, which may be caused by the generation of hydroxyl radicals penetrating into the spores.149 Two phytopathogens, Fusarium oxysporum f. sp. melonis and Pectobacterium. carotovorum subsp. carotovorum have been investigated and the results suggest that ozone-rich microbubbles showed higher disinfection activity than the millibubbles over the same period of application. It is reported that the number of these two phytopathogens decreased rapidly thanks to elevated initial ozone concentration (3
logs at 0.33 min). At the same ozone level, they concluded that OMBs provided higher disinfecting activity against both pathogens.113 Micro and nanobubbles technology was also implemented to tackle tomato airborne disease. The results highlighted that the inactivation activity against Alternaria solani Sorauer conidia was reduced by 2
logs when ozone concentration of 1.6 mg L−1 was applied. In the case of Cladosporium fulvum conidia it was found that one log reduction was achieved when 1.8 mg L−1 of ozone was used. This level of ozone application did not affect tomato growth.115 The study by Kwack et al.124 have verified that using ozone microbubbles for seed sterilization is the most feasible treatment since the germination and growth of alfalfa sprouts have not been negatively affected. Another study provides additional support into the superiority of OMBs over other sanitizers such as sodium hypochlorite.150 After washing with OMBs at 1 mg L−1 for 7 minutes, the bacterial reduction of S. typhimurium was the highest reaching the value of 2.6
log CFU g−1 or 99.8%, converting into percentage. Increasing attention has been given to the removal of persistent, highly toxic and accumulative pesticides which are extensively used in agriculture. The degradation of fluopyram is more efficient with OMBs, among different treatment methods. More specifically, the half-life of fluopyram in ozonated water was found to be 6.1 times higher compared to OMBs treatment and whereas in MBs treatment (without ozone) was only 1.3 times higher.151 The removal of fenitrothion in three kinds of vegetables (lettuce, cherry tomatoes and strawberries) was investigated and was found to be higher when OMBs generated by decompression compared to OMBs generated by gas–water circulation were used. This can be explained by the creation of a larger number of smaller OMBs by the former, yielding a higher efficiency of fenitrothion degradation as the infiltration of smaller OMBs into vegetables is easier.152
Table 5 Applications of ozone micro- and nanobubbles (OMNBs) in agriculture
Target microorganism |
Bacterial conc. (CFU mL−1) |
Ozone conc. (mg L−1) |
Disinfection efficiency with OMBs |
Ref. |
F. oxysporum f. sp. melonis |
1 × 103–1 × 104 |
1.5 ppm (15 °C) |
The number of surviving spores reached the detection limit in 45 s with OMBs instead of 60 s with OMaBs |
149
|
F. oxysporum f. sp. melonis |
∼1 × 103 |
0.1 ppm (20 °C) |
2.6 logs of surviving cells with OMBs instead of 2.9 logs with OMaBs after 180 s |
113
|
P. carotovorum subsp. carotovorum |
2.5 logs of surviving cells with OMBs instead of 2.9 logs with OMaBs after 180 s |
Alternaria solani Sorauer conidia
|
1 × 105 |
1.6 ppm |
2 logs reduction |
115
|
Cladosporium fulvum conidia
|
1.8 ppm |
1 log reduction |
S. typhimurium
|
1 × 106–1 × 107 (CFU g−1) |
1 ppm (30 °C) |
2.6 logs reduction |
150
|
5. Research limitations and needs
Although MNBs technology is widely used in various applications, there are still gaps in our understanding of the behaviour of NBs that need further investigation which is however outside the scope of this review. This critical review has tried to cover most of the important research conducted in the field of disinfection using OMNBs in order to get a better insight on the correlation of ozone dose-exposure time and microorganism viability, and highlight the major advantages of utilizing these MNBs-based processes. Besides the increasing number of research studies in this field there all still research limitations that are encountered in the implementation of this technology. In particular, the challenges that need to be addressed regarding the OMNBs are:
○ Even though there are many comprehensive studies carried out in water treatment regarding the inactivation of various microorganisms, this is not the situation for ballast water treatment. There are some investigations about the generation of nanobubbles under different salt concentrations,52 however, there is no literature about the disinfection capacity of OMNBs in real seawater. The sodium chloride present in seawater reacts quickly with ozone generating a mixture of oxidants which kill microbial pathogens. In addition, it is important to examine the inactivation efficiency when OMNBs are used in the presence of bromide in order to estimate the concentration of by-products derived from the reaction between the ozone and the bromide and compare with that created in a typical ozonation.
○ One of the objectives of this review was also to gather knowledge regarding the degradation of organic pollutants present in wastewater treatment plants by OMNBs since their existence may influence the disinfecting potential in tertiary treatment. However, the formation of oxidation by-products is an issue of greater importance since they can be more resistant towards ozone and a higher level of disinfectant may be required. As reported in the literature,153 a carbamazepine by-product was highly persistent and the ozone level had to be elevated up to 15 mg L−1, when only 5 mg L−1 of ozone was sufficient to remove the target compound. Hence, we must bear in mind the detection and identification of emerging by-products in order to evaluate in an accurate manner the efficiency of OMNBs in eliminating organic compounds.
○ A general limitation is that all studies have been performed in laboratory or small pilot scale (up to 50 L). It would be helpful to examine the upscaling of this process in the field and at industrial scale. Moreover, in this case it is important to mention that a cost/benefit analysis should also be conducted, since an ozonation system is often portrayed by high energy requirements.
○ The characterization of MNBs with high resolution has been to some extent achieved; however, there is a chance the size measurement to be misleading as the gas cavities cannot be distinguished among nanodroplets and impurities derived from the equipment or present in the water. It is worth mentioning that most studies have investigated the use of NBs on ultrapure water and hence, typical drinking water or wastewater matrices may influence the NBs size and entangle the measurement of number concentration thanks to the existence of other colloids.58 There are several MNBs generators available commercially but without providing a detailed description concerning the size distribution and concentration of the generated bubbles.35 For that reason a standard measurement protocol should be established in order to ensure the correct characterization of MNBs.
○ Finally, although theoretical models for micro–nano-bubbles mass transfer and stability154,155 have been developed they should be extended in order to simulate the reactions with microorganisms or micropollutants for a given number of OMNBs and their size distribution taking into consideration potential ozonation by-products. Such a model would be valuable for the optimal design of ozone-based disinfection systems, specifically in the case that existing treatment plants will be retrofitted by the installation of MNBs technology.
Conclusions
Even though several researchers have expressed doubts about the existence and the stability of MNBs, many studies have proven that their application by different types of gas can enhance process efficiency compared to conventional aeration since the results so far have been very encouraging. The OMNBs technology can reduce the operation and maintenance cost of an ozonation system since it can overcome at least partially the serious weakness which is the limited residual disinfection capacity and the low solubility of ozone leading to the requirement of a high ozone dose. Apart from the reduced construction and operational cost, there is another positive aspect concerning the environment. The chemical dosage is lower due to the excellent mass transfer and hence, it can be considered more eco-friendly than conventional ozonation. Further work needs to be carried out to standardize the selection of a OMNBs system in accordance with the disinfection needs in wastewater treatment and reuse, taking into account the economic and environmental impact.
Author contributions
P. S.: investigation, validation, writing first draft and revising. N. K.: supervision, conceptualization, reviewing and editing.
Conflicts of interest
There are no conflicts to declare.
Acknowledgements
This research is co-financed by Greece and the European Union (European Social Fund-ESF) through the Operational Programme “Human Resources Development, Education and Lifelong Learning” in the context of the project “Strengthening Human Resources Research Potential via Doctorate Research” (MIS-5000432), implemented by the State Scholarships Foundation (IKY).
References
- M. Helmecke, E. Fries and C. Schulte, Regulating water reuse for agricultural irrigation: risks related to organic micro-contaminants, Environ. Sci. Eur., 2020, 32, 4 CrossRef CAS.
- F. F. Reinthaler, J. Posch, G. Feierl, G. Wüst, D. Haas, G. Ruckenbauer, F. Mascher and E. Marth, Antibiotic resistance of E. coli in sewage and sludge, Water Res., 2003, 37, 1685–1690 CrossRef CAS PubMed.
- J. J. Huang, H. Y. Hu, F. Tang, Y. Li, S. Q. Lu and Y. Lu, Inactivation and reactivation of antibiotic-resistant bacteria by chlorination in secondary effluents of a municipal wastewater treatment plant, Water Res., 2011, 45, 2775–2781 CrossRef CAS PubMed.
- W. Zieliński, E. Korzeniewska, M. Harnisz, J. Hubeny, M. Buta and D. Rolbiecki, The prevalence of drug-resistant and virulent Staphylococcus spp. in a municipal wastewater treatment plant and their spread in the environment, Environ. Int., 2020, 143, 105914 CrossRef PubMed.
- S. Monteiro and R. Santos, Incidence of enterococci resistant to clinically relevant antibiotics in environmental waters and in reclaimed waters used for irrigation, J. Water Health, 2020, 18, 911–924 CrossRef PubMed.
- S. A. Rosenberg, R. E. Goldstein, S. A. Micallef, S. G. Gibbs, J. A. Davis, X. He, A. George, L. M. Kleinfelter, N. A. Schreiber, S. Mukherjee, A. Sapkota, S. W. Joseph, A. Sapkota and S. W. Joseph, Methicillin-Resistant Staphylococcus aureus (MRSA) Detected at Four U.S. Wastewater Treatment Plants, Environ. Health Perspect., 2012, 120, 1551–1558 CrossRef PubMed.
- C. W. McKinney and A. Pruden, Ultraviolet disinfection of antibiotic resistant bacteria and their antibiotic resistance genes in water and wastewater, Environ. Sci. Technol., 2012, 46, 13393–13400 CrossRef CAS PubMed.
- D. Venieri, F. Tournas, I. Gounaki, V. Binas, A. Zachopoulos, G. Kiriakidis and D. Mantzavinos, Inactivation of Staphylococcus aureus in water by means of solar photocatalysis using metal doped TiO2 semiconductors, J. Chem. Technol. Biotechnol., 2017, 92, 43–51 CrossRef CAS.
- W. Ding, W. Jin, S. Cao, X. Zhou, C. Wang, Q. Jiang, H. Huang, R. Tu, S. F. Han and Q. Wang, Ozone disinfection of chlorine-resistant bacteria in drinking water, Water Res., 2019, 160, 339–349 CrossRef CAS PubMed.
- V. K. Sharma, N. Johnson, L. Cizmas, T. J. McDonald and H. Kim, A review of the influence of treatment strategies on antibiotic resistant bacteria and antibiotic resistance genes, Chemosphere, 2016, 150, 702–714 CrossRef CAS PubMed.
- B. F. da Silva, A. Jelic, R. López-Serna, A. A. Mozeto, M. Petrovic and D. Barceló, Occurrence and distribution of pharmaceuticals in surface water, suspended solids and sediments of the Ebro river basin, Spain, Chemosphere, 2011, 85, 1331–1339 CrossRef PubMed.
- C. G. Campbell, S. E. Borglin, F. B. Green, A. Grayson, E. Wozei and W. T. Stringfellow, Biologically directed environmental monitoring, fate, and transport of estrogenic endocrine disrupting compounds in water: A review, Chemosphere, 2006, 65, 1265–1280 CrossRef CAS PubMed.
- Z. R. Hopkins and L. Blaney, An aggregate analysis of personal care products in the environment: Identifying the distribution of environmentally-relevant concentrations, Environ. Int., 2016, 92–93, 301–316 CrossRef CAS PubMed.
- M. J. Benotti and B. J. Brownawell, Microbial degradation of pharmaceuticals in estuarine and coastal seawater, Environ. Pollut., 2009, 157, 994–1002 CrossRef CAS PubMed.
- H. Dong, X. Yuan, W. Wang and Z. Qiang, Occurrence and removal of antibiotics in ecological and conventional wastewater treatment processes: A field study, J. Environ. Manage., 2016, 178, 11–19 CrossRef CAS PubMed.
- B. M. Sharma, J. Bečanová, M. Scheringer, A. Sharma, G. K. Bharat, P. G. Whitehead, J. Klánová and L. Nizzetto, Health and ecological risk assessment of emerging contaminants (pharmaceuticals, personal care products, and artificial sweeteners) in surface and groundwater (drinking water) in the Ganges River Basin, India, Sci. Total Environ., 2019, 646, 1459–1467 CrossRef CAS PubMed.
- J. Fernández-Rubio, J. L. Rodríguez-Gil, C. Postigo, N. Mastroianni, M. López de Alda, D. Barceló and Y. Valcárcel, Psychoactive pharmaceuticals and illicit drugs in coastal waters of North-Western Spain: Environmental exposure and risk assessment, Chemosphere, 2019, 224, 379–389 CrossRef PubMed.
- M. Kuster, M. J. López de Alda, M. D. Hernando, M. Petrovic, J. Martín-Alonso and D. Barceló, Analysis and occurrence of pharmaceuticals, estrogens, progestogens and polar pesticides in sewage treatment plant effluents, river water and drinking water in the Llobregat river basin (Barcelona, Spain), J. Hydrol., 2008, 358, 112–123 CrossRef CAS.
- S. L. Bartelt-Hunt, D. D. Snow, T. Damon, J. Shockley and K. Hoagland, The occurrence of illicit and therapeutic pharmaceuticals in wastewater effluent and surface waters in Nebraska, Environ. Pollut., 2009, 157, 786–791 CrossRef CAS PubMed.
- M. B. Ahmed, J. L. Zhou, H. H. Ngo, W. Guo, N. S. Thomaidis and J. Xu, Progress in the biological and chemical treatment technologies for emerging contaminant removal from wastewater: A critical review, J. Hazard. Mater., 2017, 323, 274–298 CrossRef CAS PubMed.
- J. Rivera-Utrilla, M. Sánchez-Polo, M. Á. Ferro-García, G. Prados-Joya and R. Ocampo-Pérez, Pharmaceuticals as emerging contaminants and their removal from water. A review, Chemosphere, 2013, 93, 1268–1287 CrossRef CAS PubMed.
- L. W. Matzek and K. E. Carter, Activated persulfate for organic chemical degradation: A review, Chemosphere, 2016, 151, 178–188 CrossRef CAS PubMed.
- O. M. Rodriguez-Narvaez, J. M. Peralta-Hernandez, A. Goonetilleke and E. R. Bandala, Treatment technologies for emerging contaminants in water: A review, Chem. Eng. J., 2017, 323, 361–380 CrossRef CAS.
- A. Agarwal, W. J. Ng and Y. Liu, Principle and applications of microbubble and nanobubble technology for water treatment, Chemosphere, 2011, 84, 1175–1180 CrossRef CAS PubMed.
- B. Park, S. Yoon, Y. Choi, J. Jang, S. Park and J. Choi, Stability of engineered micro or nanobubbles for biomedical applications, Pharmaceutics, 2020, 12, 1–12 Search PubMed.
- A. K. Patel, R. R. Singhania, C.-W. Chen, Y.-S. Tseng, C.-H. Kuo, C.-H. Wu and C. Di Dong, Advances in micro- and nano bubbles technology for application in biochemical processes, Environ. Technol. Innovation, 2021, 23, 101729 CrossRef CAS.
- H. Li, L. Hu, D. Song and F. Lin, Characteristics of Micro-Nano Bubbles and Potential Application in Groundwater Bioremediation, Water Environ. Res., 2015, 86, 844–851 CrossRef PubMed.
- A. Azevedo, R. Etchepare and J. Rubio, Raw water clarification by flotation with microbubbles and nanobubbles generated with a multiphase pump, Water Sci. Technol., 2017, 75, 2342–2349 CrossRef CAS PubMed.
-
U.S. EPA, Wastewater Technology Fact Sheet Ozone Disinfection, Off. Water Washington, D.C., 1999, 7.
- L. Hu and Z. Xia, Application of ozone micro-nano-bubbles to groundwater remediation, J. Hazard. Mater., 2018, 342, 446–453 CrossRef CAS PubMed.
- Z. Xiao, T. Bin Aftab and D. Li, Applications of micro–nano bubble technology in environmental pollution control, Micro Nano Lett., 2019, 14, 782–787 CrossRef CAS.
- P. Khan, W. Zhu, F. Huang, W. Gao and N. A. Khan, Micro-nanobubble technology and water-related application, Water Sci. Technol.: Water Supply, 2020, 20, 2021–2035 CAS.
- Z. Xia and L. Hu, Treatment of organics contaminated wastewater by ozone micro-nano-bubbles, Water, 2018, 11, 55 CrossRef.
- K. Hashimoto, N. Kubota, T. Okuda, S. Nakai, W. Nishijima and H. Motoshige, Reduction of ozone dosage by using ozone in ultrafine bubbles to reduce sludge volume, Chemosphere, 2021, 274, 129922 CrossRef CAS PubMed.
- A. Azevedo, H. Oliveira and J. Rubio, Bulk nanobubbles in the mineral and environmental areas: Updating research and applications, Adv. Colloid Interface Sci., 2019, 271, 101992 CrossRef CAS PubMed.
- A. Tekile, I. Kim and J.-Y. Lee, Applications of Ozone Micro- and Nanobubble Technologies in Water and Wastewater Treatment: Review, J. Korean Soc. Water Wastewater, 2017, 31, 481–490 CrossRef.
- T. Temesgen, T. T. Bui, M. Han, T. il Kim and H. Park, Micro and nanobubble technologies as a new horizon for water-treatment techniques: A review, Adv. Colloid Interface Sci., 2017, 246, 40–51 CrossRef CAS PubMed.
- Y. Shangguan, S. Yu, C. Gong, Y. Wang, W. Yang and L. A. Hou, A Review of Microbubble and its Applications in Ozonation, IOP Conf. Ser. Earth Environ. Sci., 2018, 128, 12149 CrossRef.
- A. John, A. Brookes, I. Carra, B. Jefferson and P. Jarvis, Microbubbles and their application to ozonation in water treatment: A critical review exploring their benefit and future application, Crit. Rev. Environ. Sci. Technol., 2020, 1–43 CrossRef.
- M. Takahashi, T. Kawamura, Y. Yamamoto, H. Ohnari, S. Himuro and H. Shakutsui, Effect of shrinking microbubble on gas hydrate formation, J. Phys. Chem. B, 2003, 107, 2171–2173 CrossRef CAS.
- P. Li and H. Tsuge, Ozone transfer in a new gas-induced contactor with microbubbles, J. Chem. Eng. Jpn., 2006, 39, 1213–1220 CrossRef CAS.
- S. Khuntia, S. K. Majumder and P. Ghosh, Removal of ammonia from water by ozone microbubbles, Ind. Eng. Chem. Res., 2013, 52, 318–326 CrossRef CAS.
- W. Cheng, L. Jiang, X. Quan, C. Cheng, X. Huang, Z. Cheng and L. Yang, Ozonation process intensification of p-nitrophenol by in situ separation of hydroxyl radical scavengers and microbubbles, Water Sci. Technol., 2019, 80, 25–36 CrossRef CAS PubMed.
- A. Agarwal, H. Xu, W. J. Ng and Y. Liu, Biofilm detachment by self-collapsing air microbubbles: A potential chemical-free cleaning technology for membrane biofouling, J. Mater. Chem., 2012, 22, 2203–2207 RSC.
- J. Zhang, G. Q. Huang, C. Liu, R. N. Zhang, X. X. Chen and L. Zhang, Synergistic effect of microbubbles and activated carbon on the ozonation treatment of synthetic dyeing wastewater, Sep. Purif. Technol., 2018, 201, 10–18 CrossRef CAS.
- Y. Gao, Y. Duan, W. Fan, T. Guo, M. Huo, W. Yang, S. Zhu and W. An, Intensifying ozonation treatment of municipal secondary effluent using a combination of microbubbles and ultraviolet irradiation, Environ. Sci. Pollut. Res., 2019, 26, 21915–21924 CrossRef CAS PubMed.
- L. B. Chu, S. T. Yan, X. H. Xing, A. F. Yu, X. L. Sun and B. Jurcik, Enhanced sludge solubilization by microbubble ozonation, Chemosphere, 2008, 72, 205–212 CrossRef CAS PubMed.
- K. Ebina, K. Shi, M. Hirao, J. Hashimoto, Y. Kawato, S. Kaneshiro, T. Morimoto, K. Koizumi and H. Yoshikawa, Oxygen and Air Nanobubble Water Solution Promote the Growth of Plants, Fishes, and Mice, PLoS One, 2013, 8, 2–8 Search PubMed.
- K. Ohgaki, N. Q. Khanh, Y. Joden, A. Tsuji and T. Nakagawa, Physicochemical approach to nanobubble solutions, Chem. Eng. Sci., 2010, 65, 1296–1300 CrossRef CAS.
- F. Y. Ushikubo, T. Furukawa, R. Nakagawa, M. Enari, Y. Makino, Y. Kawagoe, T. Shiina and S. Oshita, Evidence of the existence and the stability of nano-bubbles in water, Colloids Surf., A, 2010, 361, 31–37 CrossRef CAS.
- T. Uchida, S. Oshita, M. Ohmori, T. Tsuno, K. Soejima, S. Shinozaki, Y. Take and K. Mitsuda, Transmission electron microscopic observations of nanobubbles and their capture of impurities in wastewater, Nanoscale Res. Lett., 2011, 6, 1–9 CrossRef PubMed.
- H. Li, L. Hu and Z. Xia, Impact of groundwater salinity on bioremediation enhanced by micro-nano bubbles, Materials, 2013, 6, 3676–3687 CrossRef CAS PubMed.
- J. N. Meegoda, S. A. Hewage and J. H. Batagoda, Stability of Nanobubbles, Environ. Eng. Sci., 2018, 35, 1216–1227 CrossRef CAS.
- S. A. Hewage, J. Kewalramani and J. N. Meegoda, Stability of nanobubbles in different salts solutions, Colloids Surf., A, 2021, 609, 125669 CrossRef CAS.
- E. D. Michailidi, G. Bomis, A. Varoutoglou, G. Z. Kyzas, G. Mitrikas, A. C. Mitropoulos, E. K. Efthimiadou and E. P. Favvas, Bulk nanobubbles: Production and investigation of their formation/stability mechanism, J. Colloid Interface Sci., 2020, 564, 371–380 CrossRef CAS PubMed.
- L. Zhou, S. Wang, L. Zhang and J. Hu, Generation and stability of bulk nanobubbles: A review and perspective, Curr. Opin. Colloid Interface Sci., 2021, 53, 101439 CrossRef CAS.
- J. Wu, K. Zhang, C. Cen, X. Wu, R. Mao and Y. Zheng, Role of bulk nanobubbles in removing organic pollutants in wastewater treatment, AMB Express, 2021, 11, 96 CrossRef CAS PubMed.
- A. J. Atkinson, O. G. Apul, O. Schneider, S. Garcia-Segura and P. Westerhoff, Nanobubble Technologies Offer Opportunities to Improve Water Treatment, Acc. Chem. Res., 2019, 52, 1196–1205 CrossRef CAS PubMed.
- S. M. A. Movahed and A. K. Sarmah, Global trends and characteristics of nano- and micro-bubbles research in environmental engineering over the past two decades: A scientometric analysis, Sci. Total Environ., 2021, 785, 147362 CrossRef CAS PubMed.
- N. Nirmalkar, A. W. Pacek and M. Barigou, On the Existence and Stability of Bulk Nanobubbles, Langmuir, 2018, 34, 10964–10973 CrossRef CAS PubMed.
- K. Yasui, T. Tuziuti and W. Kanematsu, Mysteries of bulk nanobubbles (ultrafine bubbles); stability and radical formation, Ultrason. Sonochem., 2018, 48, 259–266 CrossRef CAS PubMed.
- V. Leroy and T. Norisuye, Investigating the Existence of Bulk Nanobubbles with Ultrasound, ChemPhysChem, 2016, 2787–2790 CrossRef CAS PubMed.
- M. Sedlák and D. Rak, Large-scale inhomogeneities in solutions of low molar mass compounds and mixtures of liquids: Supramolecular structures or nanobubbles?, J. Phys. Chem. B, 2013, 117, 2495–2504 CrossRef PubMed.
- A. J. Jadhav and M. Barigou, Proving and interpreting the spontaneous formation of bulk nanobubbles in aqueous organic solvent solutions: Effects of solvent type and content, Soft Matter, 2020, 16, 4502–4511 RSC.
- M. Alheshibri, A. Al Baroot, L. Shui and M. Zhang, Nanobubbles and nanoparticles, Curr. Opin. Colloid Interface Sci., 2021, 55, 101470 CrossRef CAS.
- H. Liu and G. Cao, Effectiveness of the Young-Laplace equation at nanoscale, Sci. Rep., 2016, 6, 23936 CrossRef CAS PubMed.
- A. Gurung, O. Dahl and K. Jansson, The fundamental phenomena of nanobubbles and their behavior in wastewater treatment technologies, Geosyst. Eng., 2016, 9328, 1–10 Search PubMed.
- S. Ljunggren and J. C. Eriksson, The lifetime of a colloid-sized gas bubble in water and the cause of the hydrophobic attraction, Colloids Surf., A, 1997, 129–130, 151–155 CrossRef.
- K. K. Thi Phan, T. Truong, Y. Wang and B. Bhandari, Nanobubbles: Fundamental characteristics and applications in food processing, Trends Food Sci. Technol., 2020, 95, 118–130 CrossRef CAS.
- J. P. Padilla-Martinez, C. Berrospe-Rodriguez, G. Aguilar, J. C. Ramirez-San-Juan and R. Ramos-Garcia, Optic cavitation with CW lasers: A review, Phys. Fluids, 2014, 26, 122007 CrossRef.
- R. Etchepare, H. Oliveira, M. Nicknig, A. Azevedo and J. Rubio, Nanobubbles: Generation using a multiphase pump, properties and features in flotation, Miner. Eng., 2017, 112, 19–26 CrossRef CAS.
- K. Kikuchi, A. Ioka, T. Oku, Y. Tanaka, Y. Saihara and Z. Ogumi, Concentration determination of oxygen nanobubbles in electrolyzed water, J. Colloid Interface Sci., 2009, 329, 306–309 CrossRef CAS PubMed.
- A. K. A. Ahmed, C. Sun, L. Hua, Z. Zhang, Y. Zhang, W. Zhang and T. Marhaba, Generation of nanobubbles by ceramic membrane filters: The dependence of bubble size and zeta potential on surface coating, pore size and injected gas pressure, Chemosphere, 2018, 203, 327–335 CrossRef PubMed.
- X. Bu and M. Alheshibri, The effect of ultrasound on bulk and surface nanobubbles: A review of the current status, Ultrason. Sonochem., 2021, 76, 105629 CrossRef CAS PubMed.
- E. P. Favvas, G. Z. Kyzas, E. K. Efthimiadou and A. C. Mitropoulos, Bulk nanobubbles, generation methods and potential applications, Curr. Opin. Colloid Interface Sci., 2021, 54, 101455 CrossRef CAS.
- A. J. Jadhav and M. Barigou, Bulk Nanobubbles or Not Nanobubbles: That is the Question, Langmuir, 2020, 36, 1699–1708 CrossRef CAS PubMed.
- K. Ulatowski and P. Sobieszuk, Gas nanobubble dispersions as the important agent in environmental processes – generation methods review, Water Environ. J., 2020, 34, 772–790 CrossRef.
- Q. Wang, H. Zhao, N. Qi, Y. Qin, X. Zhang and Y. Li, Generation and Stability of Size-Adjustable Bulk Nanobubbles Based on Periodic Pressure Change, Sci. Rep., 2019, 9, 1118 CrossRef PubMed.
- C. Wu, K. Nesset, J. Masliyah and Z. Xu, Generation and characterization of submicron size bubbles, Adv. Colloid Interface Sci., 2012, 179–182, 123–132 CrossRef CAS PubMed.
- S. Kim, H. Kim, M. Han and T. Kim, Generation of sub-micron (Nano) bubbles and characterization of their fundamental properties, Environ. Eng. Res., 2019, 24, 382–388 CrossRef.
- S. H. Oh and J. M. Kim, Generation and Stability of Bulk Nanobubbles, Langmuir, 2017, 33, 3818–3823 CrossRef CAS PubMed.
- J. Gross, S. Sayle, A. R. Karow, U. Bakowsky and P. Garidel, Nanoparticle tracking analysis of particle size and concentration detection in suspensions of polymer and protein samples: Influence of experimental and data evaluation parameters, Eur. J. Pharm. Biopharm., 2016, 104, 30–41 CrossRef CAS PubMed.
- R. Xu, Light scattering: A review of particle characterization applications, Particuology, 2015, 18, 11–21 CrossRef.
- R. Xiong, R. X. Xu, C. Huang, S. De Smedt and K. Braeckmans, Stimuli-responsive nanobubbles for biomedical applications, Chem. Soc. Rev., 2021, 50, 5746–5776 RSC.
-
V. Gnyawali, J.-Z. Wang, Y. Wang, G. Fishbein, L. H. Y. So, A. C. De Leon, E. Abenojar, A. A. Exner, S. S. H. Tsai and M. C. Kolios, Individual nanobubbles detection using acoustic based flow cytometry, Proc. SPIE 10878 in Photons Plus Ultrasound: Imaging and Sensing, 2019, p. 84 Search PubMed.
- A. K. A. Ahmed, C. Sun, L. Hua, Z. Zhang, Y. Zhang, T. Marhaba and W. Zhang, Colloidal Properties of Air, Oxygen, and Nitrogen Nanobubbles in Water: Effects of Ionic Strength, Natural Organic Matters, and Surfactants, Environ. Eng. Sci., 2018, 35, 720–727 CrossRef.
- M. Takahashi, ζ Potential
of Microbubbles in Aqueous Solutions: Electrical Properties of the Gas−Water Interface, J. Phys. Chem. B, 2005, 109, 21858–21864 CrossRef CAS PubMed.
- M. Li, X. Ma, J. Eisener, P. Pfeiffer, C. D. Ohl and C. Sun, How bulk nanobubbles are stable over a wide range of temperatures, J. Colloid Interface Sci., 2021, 596, 184–198 CrossRef CAS PubMed.
- S. Calgaroto, K. Q. Wilberg and J. Rubio, On the nanobubbles interfacial properties and future applications in flotation, Miner. Eng., 2014, 60, 33–40 CrossRef CAS.
- M. Alheshibri and V. S. J. Craig, Generation of nanoparticles upon mixing ethanol and water; Nanobubbles or Not?, J. Colloid Interface Sci., 2019, 542, 136–143 CrossRef CAS PubMed.
- D. V. B. Batchelor, F. J. Armistead, N. Ingram, S. A. Peyman, J. R. Mclaughlan, P. L. Coletta and S. D. Evans, Nanobubbles for therapeutic delivery: Production, stability and current prospects, Curr. Opin. Colloid Interface Sci., 2021, 54, 101456 CrossRef CAS.
-
H. Kobayashi, S. Maeda, M. Kashiwa and T. Fujita, Measurements of ultrafine bubbles using different types of particle size measuring instruments, Int. Conf. Opt. Part. Charact. (OPC 2014), 2014, 9232, 92320U.
-
J. C. Crittenden, R. R. Trussel, D. W. Hand, K. J. Howe and G. Tchobanoglous, 13 Historical Perspective Methods of Disinfection Commonly Used in Water Treatment Disinfection Kinetics, MWH's Water Treat. Princ. Des.
-
WHO, Bromate in Water.
-
C. von Sonntag and U. von Gunten, Chemistry of Ozone in Water and Wastewater Treatment: From Basic Principles to Applications, 2015 Search PubMed.
- R. G. Rice, C. M. Robson, G. W. Miller and A. G. Hill, Uses of ozone in drinking water treatment, J. - Am. Water Works Assoc., 1981, 73, 44–57 CrossRef CAS.
-
C. Gottschalk, J. A. Libra and A. Saupe, Ozonation of Water and Waste Water: A Practical Guide to Understanding Ozone and its Applications, 2nd edn, 2010 Search PubMed.
- D. Gardoni, A. Vailati and R. Canziani, Decay of Ozone in Water: A Review, Ozone: Sci. Eng., 2012, 34, 233–242 CrossRef CAS.
- H. Tomiyasu, H. Fukutomi and G. Gordon, Kinetics and Mechanism of Ozone Decomposition in Basic Aqueous Solution, Inorg. Chem., 1985, 24, 2962–2966 CrossRef CAS.
- K. Sehested, H. Corfltzen, J. Holcman, C. H. Fischer and E. J. Hart, The Primary Reaction in the Decomposition of Ozone in Acidic Aqueous Solutions, Environ. Sci. Technol., 1991, 25, 1589–1596 CrossRef CAS.
- S. Khuntia, S. K. Majumder and P. Ghosh, Quantitative prediction of generation of hydroxyl radicals from ozone microbubbles, Chem. Eng. Res. Des., 2015, 98, 231–239 CrossRef CAS.
- A. Megahed, B. Aldridge and J. Lowe, The microbial killing capacity of aqueous and gaseous ozone on different surfaces contaminated with dairy cattle manure, PLoS One, 2018, 13, 1–22 CrossRef PubMed.
-
N. F. Gray, Ozone Disinfection, Elsevier, 2nd edn, 2013 Search PubMed.
- J. H. Batagoda, S. D. A. Hewage and J. N. Meegoda, Nano-ozone bubbles for drinking water treatment, J. Environ. Eng. Sci., 2018, 14, 57–66 CrossRef.
- S. A. Hewage, J. H. Batagoda and J. N. Meegoda, In situ remediation of sediments contaminated with organic pollutants using ultrasound and ozone nanobubbles, Environ. Eng. Sci., 2020, 37, 521–534 CrossRef.
- S. Liu, Q. Wang, T. Sun, C. Wu and Y. Shi, The effect of different types of micro-bubbles on the performance of the coagulation flotation process for coke waste-water, J. Chem. Technol. Biotechnol., 2012, 87, 206–215 CrossRef CAS.
- T. Zheng, Q. Wang, T. Zhang, Z. Shi, Y. Tian, S. Shi, N. Smale and J. Wang, Microbubble enhanced ozonation process for advanced treatment of wastewater produced in acrylic fiber manufacturing industry, J. Hazard. Mater., 2015, 287, 412–420 CrossRef CAS PubMed.
-
J. Hoigné, in Quality and Treatment of Drinking Water II, ed. J. Hrubec, Springer Berlin Heidelberg, Berlin, Heidelberg, 1998, pp. 83–141 Search PubMed.
-
T. V. Suslow, Oxidation-Reduction Potential (ORP) for Water Disinfection Monitoring, Control, and Documentation, 2004, DOI:10.3733/ucanr.8149. Retrieved from https://escholarship.org/uc/item/1730p498.
- M. Takahashi, K. Chiba and P. Li, Formation of hydroxyl radicals by collapsing ozone microbubbles under strongly acidic conditions, J. Phys. Chem. B, 2007, 111, 11443–11446 CrossRef CAS PubMed.
- L. B. Chu, X. H. Xing, A. F. Yu, Y. N. Zhou, X. L. Sun and B. Jurcik, Enhanced ozonation of simulated dyestuff wastewater by microbubbles, Chemosphere, 2007, 68, 1854–1860 CrossRef CAS PubMed.
- A. Jabesa and P. Ghosh, Removal of diethyl phthalate from water by ozone microbubbles in a pilot plant, J. Environ. Manage., 2016, 180, 476–484 CrossRef CAS PubMed.
- F. Kobayashi, H. Ikeura, S. Ohsato, T. Goto and M. Tamaki, Disinfection using ozone microbubbles to inactivate Fusarium oxysporum f. sp. melonis and Pectobacterium carotovorum subsp. carotovorum, Crop Prot., 2011, 30, 1514–1518 CrossRef CAS.
- M. Takahashi, H. Horibe, K. Matsuura and K. Tatera, Effect of microbubbles on ozonized water for photoresist removal, J. Photopolym. Sci. Technol., 2015, 28, 293–298 CrossRef CAS.
- H. He, L. Zheng, Y. Li and W. Song, Research on the Feasibility of Spraying Micro/Nano Bubble Ozonated Water for Airborne Disease Prevention, Ozone: Sci. Eng., 2015, 37, 78–84 CrossRef CAS.
- Y. G. Lee, Y. Park, G. Lee, Y. Kim and K. Chon, Enhanced degradation of pharmaceutical compounds by a microbubble ozonation process: Effects of temperature, pH, and humic acids, Energies, 2012, 19, 4373 Search PubMed.
- W. Fan, W. G. An, M. X. Huo, W. Yang, S. Y. Zhu and S. S. Lin, Solubilization and stabilization for prolonged reactivity of ozone using micro-nano bubbles and ozone-saturated solvent: A promising enhancement for ozonation, Sep. Purif. Technol., 2020, 238(116484) CAS.
- T. Zheng, Q. Wang, T. Zhang, Z. Shi, Y. Tian, S. Shi, N. Smale and J. Wang, Microbubble enhanced ozonation process for advanced treatment of wastewater produced in acrylic fiber manufacturing industry, J. Hazard. Mater., 2015, 287, 412–420 CrossRef CAS PubMed.
- S. Liu, Q. Wang, X. Zhai, Q. Huang and P. Huang, Improved Pretreatment (Coagulation-Floatation and Ozonation) of Younger Landfill Leachate by Microbubbles, Water Environ. Res., 2010, 82, 657–665 CrossRef CAS PubMed.
- C. Wang, C. Y. Lin and G. Y. Liao, Degradation of antibiotic tetracycline by ultrafine-bubble ozonation process, J. Water Process. Eng., 2020, 37, 101463 CrossRef.
- I. Kim and J. Lee, Comparison of ozonation removal for PPCPs in secondary treated sewage by microbubble generator and ejector, Environ. Eng. Res., 2021, 27, 200163 CrossRef.
- M. Sumikura, M. Hidaka, H. Murakami, Y. Nobutomo and T. Murakami, Ozone micro-bubble disinfection method for wastewater reuse system, Water Sci. Technol., 2007, 56, 53–61 CrossRef CAS PubMed.
- T. K. Kim, T. Kim, I. Lee, K. Choi and K. D. Zoh, Removal of tetramethylammonium hydroxide (TMAH) in semiconductor wastewater using the nano-ozone H2O2 process, J. Hazard. Mater., 2021, 409, 123759 CrossRef CAS PubMed.
- Y. Kwack, K. K. Kim, H. Hwang and C. Chun, An ozone micro-bubble technique for seed sterilization in alfalfa sprouts, Weon'ye Gwahag Gi'sulji, 2014, 32, 901–905 CAS.
- W. Fan, W. An, M. Huo, D. Xiao, T. Lyu and J. Cui, An integrated approach using ozone nanobubble and cyclodextrin inclusion complexation to enhance the removal of micropollutants, Water Res., 2021, 196, 117039 CrossRef CAS PubMed.
- T. Batakliev, V. Georgiev, M. Anachkov, S. Rakovsky and G. E. Zaikov, Ozone decomposition, Interdiscip. Toxicol., 2014, 7, 47–59 CrossRef PubMed.
- P. J. Cullen, B. K. Tiwari, C. P. O'Donnell and K. Muthukumarappan, Modelling approaches to ozone processing of liquid foods, Trends Food Sci. Technol., 2009, 20, 125–136 CrossRef CAS.
- A. Furuichi, S. Arakawa, Y. Mano, I. Morita, N. Tachikawa, Y. Yamada and S. Kasugai, Comparative Analysis of Efficacy of Ozone Nano Bubble Water (NBW3) with Established Antimicrobials. Bactericidal Efficacy and Cellular Response. An in Vitro Study, J. Oral Tissue Eng., 2013, 10, 131–141 Search PubMed.
- S. Hayakumo, S. Arakawa, M. Takahashi, K. Kondo, Y. Mano and Y. Izumi, Effects of ozone nano-bubble water on periodontopathic bacteria and oral cells - In vitro studies, Sci. Technol. Adv. Mater., 2014, 15, 590–599 CrossRef PubMed.
- M. Seki, T. Ishikawa, H. Terada and M. Nashimoto, Microbicidal effects of stored aqueous ozone solution generated by nano-bubble technology, In Vivo, 2017, 31, 579–583 CrossRef CAS PubMed.
- F. Zhang, J. Xi, J. J. Huang and H. Y. Hu, Effect of inlet ozone concentration on the performance of a micro-bubble ozonation system for inactivation of Bacillus subtilis spores, Sep. Purif. Technol., 2013, 114, 126–133 CrossRef CAS.
- E. F. Karamah, F. Amalia, R. Ghaudenson and S. Bismo, Disinfection of Escherichia coli bacteria using combination of ozonation and hydrodynamic cavitation method with venturi injector, Int. J. Adv. Sci. Eng. Inf. Technol., 2018, 8, 811–817 CrossRef.
- K. Noguera-Oviedo and D. S. Aga, Lessons learned from more than two decades of research on emerging contaminants in the environment, J. Hazard. Mater., 2016, 316, 242–251 CrossRef CAS PubMed.
- M. M. Huber, A. Göbel, A. Joss, N. Hermann, D. Löffler, C. S. McArdell, A. Ried, H. Siegrist, T. A. Ternes and U. Von Gunten, Oxidation of pharmaceuticals during ozonation of municipal wastewater effluents: A pilot study, Environ. Sci. Technol., 2005, 39, 4290–4299 CrossRef CAS PubMed.
- T. A. Ternes, J. Stüber, N. Herrmann, D. McDowell, A. Ried, M. Kampmann and B. Teiser, Ozonation: A tool for removal of pharmaceuticals, contrast media and musk fragrances from wastewater?, Water Res., 2003, 37, 1976–1982 CrossRef CAS PubMed.
- R. Cruz and J. Valverde Flores, Reduction of Coliforms presents in domestic residual waters by Air-Ozone Micro-Nanobubbles In Carhuaz city, Peru, J. Nanotechnol., 2017, 1, 9 CrossRef.
- T. Azuma, K. Otomo, M. Kunitou, M. Shimizu, K. Hosomaru, S. Mikata, Y. Mino and T. Hayashi, Removal of pharmaceuticals in water by introduction of ozonated microbubbles, Sep. Purif. Technol., 2019, 212, 483–489 CrossRef CAS.
-
FAO, FAO yearbook of fishery and aquaculture statistics, Fish. Aquac. Dep., 2017, pp. 26–28 Search PubMed.
-
S. Kumar, M. Lekshmi, A. Parvathi, B. B. Nayak and M. F. Varela, Antibiotic resistance in seafood-borne pathogens, Foodborne Pathog. Antibiot. Resist., 2017, pp. 397–415 Search PubMed.
- C. Jhunkeaw, N. Khongcharoen, N. Rungrueng, P. Sangpo, W. Panphut, A. Thapinta, S. Senapin, S. St-Hilaire and H. T. Dong, Ozone nanobubble treatment in freshwater effectively reduced pathogenic fish bacteria and is safe for Nile tilapia (Oreochromis niloticus), Aquaculture, 2021, 534, 736286 CrossRef CAS.
- N. H. Nghia, P. T. Van, P. T. Giang, N. T. Hanh, S. St-Hilaire and J. A. Domingos, Control of Vibrio parahaemolyticus (AHPND strain) and improvement of water quality using nanobubble technology, Aquacult. Res., 2021, 1–13 Search PubMed.
- L. Thanh Dien, N. V. Linh, P. Sangpo, S. Senapin, S. St-Hilaire, C. Rodkhum and H. T. Dong, Ozone nanobubble treatments improve survivability of Nile tilapia (Oreochromis niloticus) challenged with a pathogenic multi-drug-resistant Aeromonas hydrophila, J. Fish Dis., 2021, 1–13 Search PubMed.
- K. Imaizumi, S. Tinwongger, H. Kondo and I. Hirono, Disinfection of an EMS/AHPND strain of Vibrio parahaemolyticus using ozone nanobubbles, J. Fish Dis., 2018, 41, 725–727 CrossRef CAS PubMed.
- Y. Kurita, I. Chiba and A. Kijima, Physical eradication of small planktonic crustaceans from aquaculture tanks with cavitation treatment, Aquacult. Int., 2017, 25, 2127–2133 CrossRef.
- S. T. Summerfelt and J. N. Hochheimer, Review of Ozone Processes and Applications as an Oxidizing Agent in Aquaculture, Prog. Fish-Cult., 1997, 59, 94–105 CrossRef.
- A. A. Gonçalves and G. A. Gagnon, Ozone application in recirculating aquaculture system: An overview, Ozone: Sci. Eng., 2011, 33, 345–367 CrossRef.
- N. V. Linh, L. T. Dien, W. Panphut, A. Thapinta, S. Senapin, S. St-Hilaire, C. Rodkhum and H. T. Dong, Ozone nanobubble modulates the innate defense system of Nile tilapia (Oreochromis niloticus) against Streptococcus agalactiae, Fish Shellfish Immunol., 2021, 112, 64–73 CrossRef CAS PubMed.
- A. Ushida, T. Koyama, Y. Nakamoto, T. Narumi, T. Sato and T. Hasegawa, Antimicrobial effectiveness of ultra-fine ozone-rich bubble mixtures for fresh vegetables using an alternating flow, J. Food Eng., 2017, 206, 48–56 CrossRef CAS.
- M. Tamaki, F. Kobayashi, H. Ikeura and M. Sato, Disinfection by ozone microbubbles can cause morphological change of Fusarium oxysporum f. sp. melonis spores, Plant Pathol. J., 2018, 34, 335–340 CrossRef CAS PubMed.
- A. Phaephiphat, W. Mahakarnchanakul and F. Yildiz, Surface decontamination of Salmonella Typhimurium and Escherichia coli on sweet basil by ozone microbubbles, Cogent Food Agric., 2018, 4, 1558496 CrossRef.
- C. Li, S. Yuan, F. Jiang, Y. Xie, Y. Guo, H. Yu, Y. Cheng, H. Qian and W. Yao, Degradation of fluopyram in water under ozone enhanced microbubbles: Kinetics, degradation products, reaction mechanism, and toxicity evaluation, Chemosphere, 2020, 258, 127216 CrossRef CAS PubMed.
- H. Ikeura, F. Kobayashi and M. Tamaki, Removal of residual pesticide, fenitrothion, in vegetables by using ozone microbubbles generated by different methods, J. Food Eng., 2011, 103, 345–349 CrossRef CAS.
- S. K. Alharbi, W. E. Price, J. Kang, T. Fujioka and L. D. Nghiem, Ozonation of carbamazepine, diclofenac, sulfamethoxazole and trimethoprim and formation of major oxidation products, Desalin. Water Treat., 2016, 57, 29340–29351 CrossRef CAS.
- S. J. Kimmerle, Modelling, simulation and stability of free surface and bulk nanobubbles in hydrogen electrolysis, IFAC-PapersOnLine, 2015, 28, 621–626 CrossRef.
- Z. Xia and L. Hu, Theoretical model for micro-nano-bubbles mass transfer during contaminant treatment, J. Environ. Eng. Sci., 2019, 14, 157–167 CrossRef.
|
This journal is © The Royal Society of Chemistry 2021 |
Click here to see how this site uses Cookies. View our privacy policy here.