Microbial response to copper oxide nanoparticles in soils is controlled by land use rather than copper fate†
Received
21st July 2021
, Accepted 27th October 2021
First published on 5th November 2021
Abstract
Copper (Cu) products, including copper oxide nanoparticles (nCuO), are critically important agricultural fungicides and algaecides. Foliar application onto crops and subsequent aerosol drift of these Cu products, especially nCuO, on to soil may alter nutrient cycling and microbial communities in both managed and unmanaged environments. We measured the influence of land use on soil microbial biomass and respiration in response to the addition of nCuO to an alluvial soil. Different land uses included grassland, forest and both organic and conventional managed row crops. Soil samples were amended with 1000 mg Cu per kg soil as CuCl2, 16 nm CuO (16nCuO), 42 nm CuO (42nCuO), and larger than nanoparticle sized bulk CuO (bCuO). Copper availability immediately increased in all soils following Cu addition in the order of CuCl2 > 16nCuO > 42nCuO > bCuO. After 70 days Cu availability was diminished across land uses and lowest in soils treated with bCuO. Using X-ray absorption near edge structure (XANES) spectroscopy, we determined that the relatively high availability of Cu after treatment with nanoparticle sized CuO was due to the dissolution of CuO particles and subsequent adsorption by soil materials. Respiration, an indicator of microbial activity, was suppressed by Cu additions, especially CuCl2. Copper effects on soil microbial biomass were sensitive to land use. In agricultural soils, microbial biomass was unaltered by Cu form, regardless of concentration, whereas in unmanaged soils, it decreased following exposure to CuCl2 and 42nCuO. Our results suggest that land use history has little impact on Cu chemical fate in soils, but strongly modulates microbial response to Cu exposure. These results are especially important for organic agricultural systems where copper fungicides are widely used but may suppress microbial mineralization of nutrients from soil organic matter.
Environmental significance
Copper based fungicides, including copper oxide nanoparticles, are widely used in agriculture and represent a direct and intentional release of nanoparticles into the plant and soil system. We present evidence that the microbial response to exposure to nanosized and larger CuO particles (16 nm, 42 nm, 430 nm) and Cu salts (CuCl2) is unique to historical land management rather than copper form or concentration. Generalizing soil microbial community response to nanoparticle exposure across soils is therefore challenging as previous land management can be the primary influence on the observed soil microbial community response to nanoparticle treatment.
|
Introduction
Since the adoption of the Bordeaux mixture (CuSO4 and Ca(OH)2) for fungal pathogen control in 19th century French vineyards, copper (Cu) has become a globally important tool for fungal and algal control.1 In 2017 alone, 980
000 ha of horticultural land in California were treated with 1.55 Gg of Cu from Cu-based fungicides and algaecides.2 In North America, South America, Europe, and Australia, Cu inputs to vineyards and orchards generally range between 1 and 11 kg Cu per ha per year, resulting in Cu accrual rates in excess of 5 mg Cu per kg soil per year in the surface 10 cm.3–7 During foliar fungicide application, up to 50% of all Cu is immediately deposited on the soil surface through aerosol drift and/or foliar runoff, exposing surface soil to concentrated Cu solutions as high as 30
000 mg Cu per L.8–11
Use of highly available forms of Cu such as CuSO4 in fungicidal sprays can induce leaf damage, whereas less available or ‘slow release’ forms like Cu(OH)2 mitigate this risk, though often at the cost of prophylactic efficacy.12 Decreasing the size of copper compounds to nanoparticle dimensions can alter the dissolution rate of these compounds, increasing the efficacy of the compounds.13–15 Nano-sized copper formulations of Cu(OH)2 are already being commercially adopted and used with success in agricultural systems.16 Nano-sized copper(II) oxide particles (nCuO) were recently identified as a potential candidate for the control of Verticillium wilt fungus, a common pathogen that causes economic loss for high-value crops such as almonds, tomatoes, and pistachios.13 Further, nCuO was measured to help control Fusarium wilt in tomatoes.17 The use of nCuO may offer significant benefits over traditional slow-release Cu fungicides by controlling pathogens with lower total amounts of Cu applied.13,15 However, nCuO may also negatively affect soil microbial communities performing essential nutrient cycling and other soil health functions.18,19 Despite ubiquitous usage and accumulation in and around agricultural soils, the fate and influence of nCuO and Cu in general on soil microbial biomass and activity is still poorly understood.3,14,18–20
The effects of Cu and nCuO exposure on microbial biomass and activity are often negative, with reduction dependent upon Cu input amount, soil texture, pH, and soil organic carbon (SOC) content.14,15,20–24 Soils with low pH, low organic carbon content, and coarse texture typically have more biologically available Cu than soils with high pH, high organic carbon content, and fine texture.20–22,24 Regardless of soil properties, microbial communities in agricultural systems are particularly prone to Cu exposure through repeated application of Cu fungicides, potentially threatening microbial biomass and function.25 Responses are dose dependent, with limited responses at low doses and non-linear perturbations experienced at high doses.19,22,23,26 Therefore, repeated application of Cu fungicides and management practices that affect soil pH and SOC content may be expected to alter the response of soil microbial communities to Cu and nCuO exposure by altering Cu availability.24 This may be particularly problematic for nCuO with greater solubility than larger particulate forms (e.g., micron-sized), which may significantly increase Cu availability in soil compared to other forms of Cu, potentially further perturbing microbial biomass and function.14,18,27–30
Historically, potential metal availability to soil microbial communities is often measured using aqueous extractions, including deionized water or dilute solutions of salts, acids and/or bases.31,32 However the speciation of ions in these extracts is different than what is in the soil and thus from that to which soil microbial communities are exposed. These limitations may be avoided by assessing the distribution and chemical speciation of Cu in soils directly using micro-X-ray fluorescence microscopy (XFM) and X-ray absorption near edge structure (XANES) spectroscopy, respectively. Unlike aqueous extraction approaches to Cu speciation, these techniques enable identification of discrete changes to Cu speciation and distribution in solid samples and can be related to changes in microbial biomass and function after exposure to various forms of Cu.27,33–36
Here we investigate how Cu form, size, and dose effect soil microbial biomass and activity across a soil carbon gradient arising from different land uses. This work bridges a causal gap between chemical and biological responses of soil to inputs of Cu. Specifically, the effect of 1000 mg Cu per kg soil from CuCl2, 16 nm CuO (16nCuO), 42 nm CuO (42nCuO), and bulkier, 430 nm CuO (bCuO) on water extractable Cu, pH, and cumulative soil respiration from soils differing exclusively in historical land use were measured during a 70 day incubation. After 70 days, soil microbial biomass was then measured by phospholipid fatty acid analysis. The distribution and speciation of Cu in the soils treated with copper after 70 days was determined with XFM and XANES to allow direct correlation of Cu speciation on the microbial response to nanosized and larger bCuO particles. We hypothesized that exposure to high concentrations of soluble copper (CuCl2) would alter soil respiration and soil microbial biomass to a greater extent than sparingly soluble forms of Cu, such as micron-sized bCuO. Further, we hypothesized that Cu speciation in soils after 70 days would be indicative of the degree of CuO dissolution, with nCuO dissolving to a greater extent than bCuO, and would account for any differences observed in available Cu after 1 hour and 70 days. Finally, we predicted that increasing SOC would increase soil microbial community resilience in the face of Cu exposure, with microbial biomass and soil respiration in high SOC soils being less responsive to Cu treatment than in low SOC soils.
Materials and methods
Soils collection
Soils representing managed agricultural use and unmanaged forest and grassland systems were sampled in October 2016 at the Russell Ranch Sustainable Agriculture Facility at the University of California-Davis (Fig. S1†). The agricultural systems were tomato-corn rotations under either conventional (mineral fertilizer and synthetic pesticides) (Con) or organic management (compost and winter legume cover crop, no synthetic pesticides) (Org) for 23 years at the time of sampling. Neither agricultural soil was previously treated with copper-based fungicides. Unmanaged uses included a restored grassland with native grass species (Gra) and a riparian woodland dominated by valley oak (Quercus lobata) with an understory of native and invasive grasses (For). Soils developed on mixed mineralogy and carbonate-rich alluvium from nearby Putah Creek and are classified as Rincon silty clay loam (fine, smectitic, thermic Mollic Haploxeralfs) and Yolo loam silt loam (fine-silty, mixed, superactive, nonacid, thermic Mollic Xerofluvents) (Fig. S1†).37 Though classified as different soil types, surface soils at the study site are similar in mineralogy, pH, and particle size distribution across all land uses, differing mainly in organic carbon (OC) content. Soils were sampled to a depth of 0–10 cm in triplicate and composited from 3 sampling sites separated by at least 50 m and gently crushed to pass through a 2 mm sieve before further air-drying prior to analysis of chemical properties (Table 1, Fig. S1†) and incubation experiments.
Table 1 Select soil chemical properties for soils taken from the conventional (Con), organic (Org), grassland (Gra), and forest (For) systems at the Russell Ranch Sustainable Agricultural Facility near Winters, California, USA
Land use system |
SOC (g kg−1) |
pH (1 : 5 water) |
Water-extractable Cu (mg kg−1) |
Total Cu (mg kg−1) |
Olsen P (mg kg−1) |
Total P (mg kg−1) |
All data presented as mean ± standard deviation (SD), n = 3. Means separation was analyzed by Tukey's HSD with significance declared at p ≤ 0.05 and differences are denoted with lowercase letters.
|
Con |
9.8 ± 0.4a |
7.5 ± 0.02b |
0.02 ± 0.02a |
50.6 ± 0.8b |
8 ± 0.2a |
512 ± 12a |
Org |
12.7 ± 0.3b |
7.6 ± 0.05a |
0.18 ± 0.02c |
62.5 ± 0.4c |
30 ± 0.5c |
891 ± 64c |
Gra |
14.3 ± 0.5c |
7.2 ± 0.07c |
0.09 ± 0.05b |
52.1 ± 0.8b |
8 ± 0.8ab |
539 ± 13b |
For |
25.3 ± 1.6d |
7.3 ± 0.03c |
0.08 ± 0.01b |
41.2 ± 0.1a |
10 ± 1b |
539 ± 9b |
Soil physico-chemical characterization
Soil OC was determined by dry combustion after ball milling (ECS 4010 Costech Elemental Analyzer).38 Soil pH and water extractable metals were determined after a 1 hour equilibration of 1 g of soil with 5 ml of water (>18.2 MΩ cm resistivity) at 28 °C.39 After equilibration, the suspension pH was measured and a 1 ml aliquot was sub-sampled, centrifuged at 21
000g for 30 min, after which the supernatant was collected and diluted 1
:
10 in 1% nitric acid for elemental analysis by inductively coupled-plasma mass spectrometry (ICP-MS). For total Cu and total P analysis, 1 g of soil was added to 50 ml volumetric glass digestion vessels and acid digested with 7.0 ml of trace metal grade HCl (12.1 M) and 2.3 ml of HNO3 (15.8 M) and allowed to equilibrate overnight. The following day, soils were digested at 120 °C for 4 h, vortexed repeatedly to ensure adequate mineralization, and then brought to volume. The resulting digestate was diluted 1
:
10 with 1% HNO3 for trace metal analysis by ICP-MS. Calibration curve accuracy was confirmed by certified reference material (1640a). Digestion blanks and a soil reference material (ERM CC141) were included in the digestion process for quality control and recovery assurance. Recovery of total Cu and total P in ERM 141 were >95%. Soil-available ammonium and nitrate extracted by 2 M KCl (10 g soil: 50 ml extractant) for 60 min were measured colorimetrically.40,41 Soil-available P (Olsen P) was determined by extraction with 0.5 M NaHCO3 at pH 8.5 (1 g of soil: 20 ml extractant) for 30 min and colorimetric determination as molybdate-reactive phosphate.42,43
Nanoparticle procurement and characterization
Micron size copper oxide particles (bCuO) and 42 nm copper oxide particles (42nCuO) were purchased from Acros Organics (USA) and US Research Nanomaterials, Inc. (USA), respectively. These particles were previously described in Rippner et al. 2020 and recharacterized for the current study, with good agreement between the two sets of data.44 The smallest 16 nm copper oxide particles (16nCuO) were previously synthesized and further characterized for the current study.28,45 Primary particle size and shape of all CuO particles was determined by transmission electron microscopy (TEM, Philips CM-12). Mean primary particle size (n > 100) for CuO samples was calculated from TEM images using Fiji.46 Particle mineralogy was confirmed by X-ray diffraction (Rigaku Ultima IV, Japan).28 Nanoparticle zetapotential and hydrodynamic diameter in 1 mM KCl was measured with a Zeta-Plus (Brookhaven Instruments Corp., USA).
Copper treatments
Prior to Cu exposure, 80 g of air-dried soil was added to 946 ml acid-washed glass jars. Soils were then exposed to 1000 mg Cu per kg soil in 3 forms: (1) ionic, as aqueous copper chloride (CuCl2), (2) nanoparticle, as 16 nm and 42 nm diameter CuO (16nCuO and 42nCuO), and (3) larger, extending into microparticle CuO (bCuO). Cu treatments were implemented using the same volume of 18.2 MΩ cm water regardless of Cu source via pipette. Soils without copper amendment were also wetted with the same volume of 18.2 MΩ cm water, incubated, and included in the analysis at all time points. Each incubated soil treatment was established in triplicate.
Incubation and soil respiration
After Cu exposure, all soils were brought to 70% of water holding capacity (WHC) using 18.2 MΩ cm water, capped with a Ball® jar lid with a butyl rubber septum, and incubated in the dark for 70 days at 28 °C.40,47–49 Gas samples were collected by sampling 1 ml of gas from the headspace of 946 ml jars on days 1–5, 9, 14, 19, 24, 29, 35, 39, 44, 49, 54, 60, 64, and 70. The CO2 content in the headspace was measured by an infrared gas analyzer (model S-151, Qubit Systems Inc., Kingston, Canada).49 After each measurement, the jars were allowed to equilibrate with the atmosphere for 10 min under a fume hood to facilitate soil gas exchange.48 To replenish water lost evaporatively during the equilibration period, the soils were adjusted to 70% WHC on days 9, 39, 60, and 70. WHC never decreased below 65%.
Gas flux calculations
Total soil respiration was calculated as the sum of soil CO2 flux (μmol CO2-C per kg soil) at each sampling time (eqn (1)). | 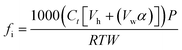 | (1) |
where f is the gas flux (μmol CO2-C per kg soil); Ct (μL CO2-C per L) is the gas concentration in the gas phase at time t; Vh (ml) is the volume of the headspace; Vw (ml) is the volume of water in the soil during the incubation; α (ml gas per ml water) is the Bunsen absorption coefficient = 0.759 for CO2; P is the standard atmospheric pressure (101.325 kPa); R is the universal gas constant (8.31451 L kPa mol−1 K−1); T is temperature in Kelvin (301.15 K); and W is the oven dry mass of soil (kg). Gas flux emissions were corrected for ambient CO2 concentration measured at each time point.39
Measuring copper availability and pH at 1 hour and 70 days
Soil pH and water extractable metals were determined 1 hour after treatment application and then again after the 70 day incubation period. Briefly, 1 g of soil was incubated with 5 ml of water (18.2 MΩ cm) for 1 hour at 28 °C.39 After equilibration, the suspension pH was measured and a 1 ml aliquot was sub-sampled and centrifuged at 21
000g for 30 min. The supernatant was diluted 1
:
10 in 1% nitric acid for elemental analysis by ICP-MS using a certified reference material (natural water, NIST 1640a).
X-ray fluorescence microscopy and X-ray absorption spectroscopy
Soils from the conventional and forest systems that represented the lowest and highest SOC content were selected for X-ray fluorescence microprobe (XFM) analyses. Soils were air dried and ground to 500 μm prior to measurement. Soils from the conventional plots were imaged at −20 °C on the XFM Beamline 10.3.2 of the Advanced Light Source (ALS) at the Lawrence Berkeley National Laboratory, Berkeley, CA, USA.50–52 All data were collected in fluorescence mode using a Canberra 7-element Ge solid state detector. Samples were mounted onto a Peltier stage (−20 °C) and scanned in 2D. Elemental distribution mapping was carried out at 9080 eV (100 eV above the Cu K-edge) with 7 × 7 μm pixels.
Soils from the forest plots were imaged on the VESPERS XFM beamline at the Canadian Light Source (CLS), Saskatoon, SK, CA.53 Samples were mounted onto a stage (25 °C) and scanned in 2D. All data were collected in fluorescence mode using a Hitachi 4-element Si solid state detector. Mapping was carried out at 9080 eV (100 eV above the Cu K-edge) with image resolution limit of 2 × 2 μm pixels.50,54
X-ray adsorption near edge structures (XANES) data were collected on one sample from every Cu× land use treatment from CuCl2, 16nCuO, and 42nCuO on the HXMA beamline at the CLS.55 Due to time and equipment constraints, XANES data was only collected for two soil samples treated with bCuO (Con and For). To prevent Cu reduction, soil XANES data were collected at 63 K.56 Standards for Cu adsorbed to silicate minerals, Cu complexed by Suwanee River fulvic acid, Suwanee River natural organic matter, a sodium salt of humic acid from lignite, or phytate, and CuO were prepared following published methods.57–59
Specifically, Cu-silicate standards were prepared following Furnare et al. 2005. Copper solutions (4.0 × 10−5 M Cu) were made using copper chloride in 18.2 MΩ cm water that was boiled and cooled by purging with N2 gas. One gram of ground albite, chlorite, illite, montmorillonite, orthoclase, quartz, serpentine, and vermiculite were individually suspended in 30 ml of copper solution inside of 3500 Dalton SpectraPor 3 dialysis bags (Rancho Dominguez, CA). Each bag was then suspended inside of 1 L bottles containing 970 ml of copper solution continuously purged with N2 gas. The solution in the bottles was changed every 12 hours over a 36 hour equilibration period. After 36 hours, the dialysis bags were removed, and the suspended minerals were decanted into centrifuge tubes and pelleted out by centrifugation. After centrifugation, the supernatant was decanted, and the mineral solids were gently dried under a N2 atmosphere then stored in a desiccator at room temperature until analysis.
Standards for copper complexed by Suwanee River fulvic acid, Suwanee River natural organic matter, and a sodium salt of humic acid from lignite (Sigma Aldrich) were prepared following Karlsson et al. 2006. Specifically, 100 mg of each material was weighed into 2 ml centrifuge tubes with CuCl2 dissolved in N2 purged 18.2 MΩ cm water to yield a Cu concentration of 10 mg g−1 on a dry mass basis. Solutions were shaken for 72 hours and then dried under a N2 atmosphere and stored in a desiccator at room temperature until analysis.
The copper phytate standard was prepared following He et al. 2006.59 Briefly, 30 ml of a N2 purged solution of 0.05 M sodium phytate (C6H6O24P6Na12) was added to 100 ml of a N2 purged solution containing 0.2 M of HCl and 0.09 M of CuCl2. The pH of the solution was slowly raised to pH 6 over the course of an hour using 1 M NaOH while purging with N2 gas. The precipitate was collected by bottle top filtration (0.22 μm filter) and washed with 60 ml of N2 purged 18.2 MΩ cm water. The filtrate was dried under a N2 atmosphere and stored in a desiccator at room temperature until analysis.
The 16nCuO particles were made following Siddiqui et al. 2013.45 Briefly, 300 ml of 0.2 M copper acetate monohydrate [Cu(CH3COO)2·H2O] and 1 ml of glacial acetic acid was heated and mixed vigorously. Upon boil, 15 ml of 6 M NaOH solution was rapidly added to the vigorously mixed solution, forming copper oxide nanoparticles. The resulting precipitate was concentrated by centrifugation, washed repeatedly with 18.2 MΩ cm water, and dried at 60 °C for 6 hours.
The remaining standards, other than the 42nCuO particles, were purchased from Sigma Aldrich and were of ACS purity or better (Table S1†). Copper K-edge XANES measurements of the Cu-standards were taken on either the HXMA or XFM 10.3.2 beamlines. Standard spectra of Cu complexed by organic matter were collected at 63 K to limit organic matter oxidation and subsequent Cu reduction.56 The remaining standards were collected at room temperature. Three individual spectra were collected for each standard and were deadtime-corrected, deglitched, calibrated using a Cu foil (1st derivative maximum value set at 8980.48 eV) pre-edge background subtracted, and post-edge normalized using custom LabVIEW software available at the beamline.60 No evidence of Cu reduction was observed when evaluating spectral quality from each of the three scans per standard or sample. Soil Cu speciation was determined by least-square linear combination fitting using custom LabVIEW software available at ALS beamline 10.3.2 and using Athena (Demeter, Ver. 0.9.26).61–63
PLFA analysis
After completion of the incubation experiment, soils were homogenized and a sub-sample from each jar was flash frozen in liquid nitrogen and lyophilized for PLFA analysis by Microbial ID, INC (Newark, DE). Total soil microbial biomass was calculated as the sum of 37 individual PLFA biomarkers that were present across all soils (μmol PLFA kg−1 soil) (Table S2†).64–69
Statistical methods
Data were tested for assumptions of analysis of variance using the Shapiro–Wilk test for normality of residuals and Levene's test for homogeneity of variance. Data that did not meet these criteria were log transformed to meet the assumptions of ANOVA prior to final analysis. Multiple comparisons were made using Tukey's honestly significant difference test (Tukey's HSD) with significance declared at p ≤ 0.05 and denoted with differing lower-case letters. Standard least squares linear regression was performed to analyze the effect of Cu form and use on soil response variables after Cu treatment. Any significant interactions were interpreted using least-squares means interaction plots and Tukey's HSD. The standardized effect size of each factor to the results was estimated using the F-statistic and reported as Fi,j = F-statistic, where i = specific treatment degrees of freedom-1 and j = total degrees of freedom – all treatment degrees of freedom-1. The F-statistic comes from the ratio of between treatment variance to within treatment variance; by comparing the F-statistic between two treatment groups such as copper form and land use, the relative contribution of each treatment group to modeled results can be estimated. All statistical analyses were performed with JMP 15 Pro (2020; SAS Institute, Cary, NC) and figures were made in Python (Version 3.6) using the Pandas, Matplotlib, and Seaborn libraries.70–72
Results and discussion
Soil characterization
Long term differences in land use altered the chemical properties of the soils at Russell Ranch (Table 1, Fig. S1†). SOC was highest in the For and Gra soils compared to the agricultural soils. This is because plant residues are not removed in these systems leading to an accumulation of soil carbon over time. After twenty-three years, Org management led to increased SOC relative to soils under Con management. This is due to the growth of cover crops and inputs of compost that offset carbon loss due to plant residue removal during cropping.38 Soil pH was higher in the agricultural systems (Con and Org) than Gra or For systems. Available Cu was highest in Org systems and lowest in Con systems. Soil Cu accumulation (>20%) under Org compared to the other soils was attributed to the relatively high Cu content (200–600 mg kg−1) of the poultry litter compost applied at 9 Mg ha−1 annually for 26 years.73 The Con and Gra soils had similar total Cu content, whereas the For soil had less total Cu, potentially due to its unique location on a lower alluvial terrace than the other soils. Though elevated SOC and pH are often associated with decreased soil Cu bioavailability, these results suggest that even relatively modest metal inputs delivered via organic matter amendments can drive soil metal availability (Table 1).74
Available and total P were greatest in the Org and lowest in the Con and Gra soils (Table 1). This is due to the low ratio of N
:
P in composts relative to plant needs; when composts are applied to meet the N requirements of crops, P is almost always applied in excess of crop nutrient needs.75 Over time, this excess P builds up due to the limited mobility of P in soils. Transition metals such has Cu have a high affinity to form insoluble P complexes, potentially leading to Cu immobilization in soils with excess available and total P from long term compost application.76,77
CuO particle characterization
Mean particle size was 16 ± 10 nm for 16nCuO, 42 ± 26 nm for 42nCuO, and 430 nm ± 640 nm for bCuO (Fig. 1A–C). The large variance in particle size for the bCuO particles was due to a large fraction of very large particles >1000 nm. Despite some overlap in the particle size for each of the CuO particle size classes, mean particle diameters were different (Fig. 1D). For all particles, CuO speciation was confirmed by bulk X-ray diffraction, and for 16nCuO and 42nCuO by bulk X-ray absorption near edge structure (XANES) spectroscopy (Fig. 1E and S2†). X-ray peak broadening in both the diffractograms and XANES spectra were observed with decreasing particles size (Fig. 1E), due to the smaller crystalline domain size of the nanoparticles increasing signal scattering.78 An increasing proportion of surface defects per unit mass for the nCuO can decrease the degree of crystallinity per unit mass of CuO, manifesting in the decreased diffractogram intensities observed here. Such defects could lead to enhanced dissolution of nCuO compared to bCuO.79,80
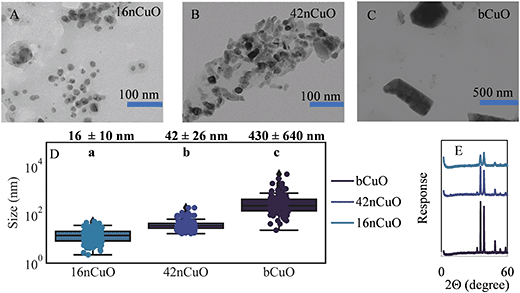 |
| Fig. 1 Copper oxide particle characterization: A) 16nCuO, B) 42nCuO, C) bCuO, D) mean particle size distribution for 16nCuO (n = 153), 42nCuO (n = 154), and bCuO (n = 101); E) diffractograms for 16nCuO, 42nCuO, and bCuO; mean size comparisons were analyzed by Tukey's HSD with significance declared at p ≤ 0.05 and denoted with lower case letters. | |
In 1 mM KCl, the zetapotential (average surface charge) and hydrodynamic radius (size when dispersed in solution) of the 16nCuO particles was 43 ± 1 mV and 310 ± 13 nm, respectively, and for the 42nCuO particles, 24 ± 1 mV and 2500 ± 55 nm, respectively. The zetapotential and hydrodynamic radius of the bCuO particles were not recorded due to rapid sedimentation of the bCuO particles when suspended. Positive zetapotentials for nCuO particles in dilute salt solutions were previously measured by others and are expected in neutral pH solutions given the range of isoelectric points (pH 6.9–9.5) for CuO found in the literature.81–84 Nanoparticles with zeta potentials >30 mV or ≤30 mV are generally considered to be stable in suspension due to electrostatic repulsion, while particles with zeta potentials closer to 0 mV are prone to aggregation. Both 16nCuO and, to a greater extent, 42nCuO, aggregated in 1 mM KCl as evidenced by the relatively large hydrodynamic radii of the 2 particle size classes compared to their mean particle size as determined by TEM (Fig. 1A, B, and D). Differences in the respective hydrodynamic radii of the two sizes of CuO likely reflect differences in surface charge.85 The molarity of the soil solution is likely significantly greater than 1 mM, leading to enhanced aggregation due to diminished electrostatic repulsion tempered by the presence of naturally occurring ligands in solution such as HSO4−, SO42−, HPO42−, HCO3−, and acid functional groups of dissolved organic matter.23,86–89 These ligands can alter the surface charge of nanoparticles leading to aggregation.90,91
Influence of CuCl2, nCuO, and bCuO on available Cu and pH over time
Background soil Cu availability in untreated soils was greatest in the Org soil and lowest in the Con soil (Fig. 2A, Table S2†). Among untreated soils, soil pH was higher in the agricultural soils compared to the unmanaged systems after 1 hour (Fig. 3A, Table S5†). Under aerobic conditions, soil Cu availability is controlled by soil pH, with decreases in pH mobilizing Cu and increases in pH immobilizing Cu.1,4,7,58,89,92–94
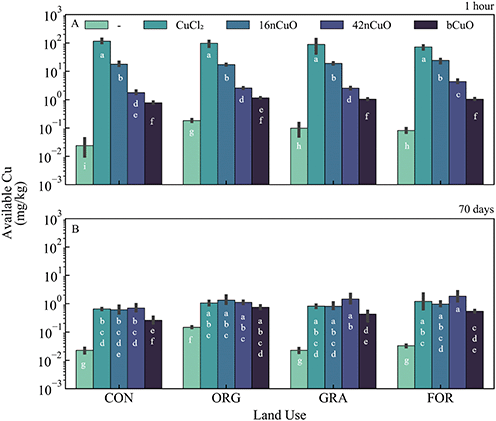 |
| Fig. 2 Available copper (Cu) at A) 1 hour and B) 70 days in soils from conventional (Con), organic (Org), grassland (Gra), and riparian forest (For) systems untreated (—) or treated with 1000 mg copper Cu per kg soil in the form of CuCl2, 16nCuO, 42nCuO, or bCuO. Data are presented as mean ± standard deviation (n = 3). Means separation was analyzed by Tukey's HSD with significance declared at p ≤ 0.05 and differences are denoted with differing lower-case letters. | |
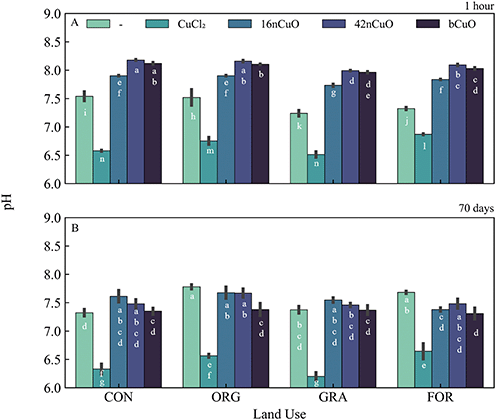 |
| Fig. 3 Soil pH at A) 1 hour and B) 70 days in soils from conventional (Con), organic (Org), grassland (Gra), and forest (For) systems untreated (—) or treated with 1000 mg copper Cu per kg soil in the form of CuCl2, 16nCuO, 42nCuO, or bCuO. Data are presented as mean ± standard deviation (n = 3). Means separation was analyzed by Tukey's HSD with significance declared at p ≤ 0.05 and differences are denoted with differing lower-case letters. | |
One hour after exposure to copper, soil Cu availability was dependent on the interaction between Cu form and use (F9,31 = 11, p < 0.0001), though Cu form (F3,31 = 2830, p < 0.0001) had a stronger effect on Cu availability than land use (F3,31 = 8, p = 0.0004) (Fig. 2A, Table S3†). Soil pH at 1 hour after treatment with Cu was specific to the interaction between land use and Cu form (F9,31 = 40, p < 0.0001) (Fig. 3A, Table S5†). However, Cu form (F3,31 = 11
000, p < 0.0001) had a much stronger effect on soil pH than land use (F3,31 = 160, p < 0.0001) (Fig. 3A, Table S4†). The addition of CuO, regardless of size, elevated soil pH, though the 16nCuO particles increased pH the least (Fig. 3A, Table S5†). The buffering capacity of colloid solutions is well known and is controlled by the isoelectric point of the minerals in suspension.71–73,95–97 After 1 hour equilibrium, the pH of the stock suspension for 16nCuO (pH 5.5 ± 0.3) was significantly lower than the pH of 42nCuO suspensions (pH 8.1 ± 0.2), and bCuO (pH 7.9 ± 0.2). Particle size-dependent increases in soil pH at 1 hour after application were likely due to differences in the surface charge and buffering capacity of the differently sized particles, rather than by CuO dissolution associated condensation reactions.20,29,98–101 Treatment with CuCl2 resulted in soil acidification, likely via Cu2+ hydrolysis, which further increased Cu availability from the highly soluble salt (Fig. 3A, Table S4†). Copper availability after 1 hour was inversely related to particle size (CuCl2 > 16nCuO > 42nCuO > bCuO) with pH playing little role in the observed differences in Cu availability among the CuO treatments (Fig. 2A, Tables S3 and S5†). Specifically, soil pH after 1 hour was the same after treatment with 42nCuO and bCuO, but Cu availability was significantly greater after treatment with 42nCuO compared to bCuO (Fig. 2A and 3A, Tables S3 and S5†). Similar size-controlled particle dissolution kinetics were previously reported in both water and soils.79,80,102
Over time, absolute soil Cu availability decreased across all Cu treatments. Copper availability after 70 days in soils exposed to copper was driven by Cu form (F3,32 = 21, p < 0.0001) to a greater extent than by land use (F3,32 = 13, p < 0.0001), with no interaction between the two (F9,32 = 1.2, p = 0.32) (Fig. 2B, Table S4†). Cu availability after 70 days was lowest in soils treated with bCuO (Fig. 2B, Table S4†). Soils under Con management had the lowest Cu availability after 70 days regardless of Cu treatment source (Fig. 2B, Table S4†). However, after 70 days, soil pH after treatment with copper was specific to the interaction between land use and Cu form (F9,32 = 4.9, p = 0.0004), though Cu form (F3,32 = 360, p < 0.0001) had a stronger effect on soil pH than land use (F3,32 = 7.3, p = 0.0007) (Fig. 3B, Table S6†). In soil from the Con system, treatment with 16nCuO increased soil pH (7.61 ± 0.12) while treatment with CuCl2 (pH 6.33 ± 0.11) decreased pH relative to the untreated control (pH 7.29 ± 0.06). These results are consistent with water condensation during CuO dissolution and water hydrolysis by Cu2+ during CuCl2 dissolution.98,103 In soil from the Org system (Fig. 3B), treatment with 1000 mg kg−1 bCuO (pH 7.38 ± 0.13) and CuCl2 (pH 7.49 ± 0.22) decreased pH compared to the untreated control (pH 7.79 ± 0.06). In the Gra system, soil pH decreased only with CuCl2 (pH 6.20 ± 0.08) relative to the untreated control (pH 7.40 ± 0.08) whereas in the forest soil, pH decreased after treatment with 16nCuO (pH 7.38 ± 0.04), bCuO (pH 7.31 ± 0.12), and CuCl2 (pH 6.64 ± 0.16) relative to the untreated control (pH 7.68 ± 0.03) (Fig. 3B, Table S6†). It is unclear why the treatment with bCuO in the organic system and both 16nCuO and bCuO in the forest system decreased soil pH (Fig. 3B, Table S6†). Both nCuO and bCuO have been previously shown to increase soil pH over short time periods, and increase or decrease pH over longer time periods.29,36,104
The relatively high pH (>7.2) of the soils in the current study likely led to rapid Cu immobilization after dissolution, limiting Cu availability.85,105 Regardless of treatment form or concentration, Cu availability decreased over time with the largest absolute change associated with CuCl2 and 16nCuO compared to 42nCuO and bCuO (Fig. 2A and B, Tables S3 and S4†). However, changes in soil pH were specific to Cu form. Treatment with bCuO decreased soil pH over time compared to treatment with 42nCuO and both decreased pH over time more than CuCl2, 16nCuO, or “no Cu” treatment (Table S7†). Other investigators have reported Cu availability to increase over time in acidic soils amended with CuO particles due to CuO dissolution under acid soil conditions.80–84 Under the alkaline soil conditions of the current study, immobilization would be favored due to adsorption by silicates or complexation by soil organic matter.105,106
Despite the limited availability of Cu in alkaline soils, long term application of Cu based fungicides, including nCuO, can significantly increase total soil Cu, leading to Cu saturation of the cation exchange complex and altering plant growth.3,4,6 Total soil Cu concentrations of 1500–3000 mg Cu per kg soil have been observed in agricultural soils due to long-term (>100 year) use of Cu fungicides, and in some cases even necessitating changes in land use.4–7
Cu speciation 70 days after treatment with CuCl2, nCuO, and bCuO
Bulk copper XANES spectroscopy was employed to speciate Cu in treated soils. Cu added as CuCl2 was primarily bound by soil materials including adsorbed to silicates and complexed by soil organic matter (Tables 2 and S8†).107 In XFM maps of both the Con and For soils treated with CuCl2, individual soil particles were visible in both maps with Cu, Ca, and Fe fluorescence channels (Fig. 4A and I) as well as the Cu channel alone (Fig. 4E and M). This indicated that the copper from the CuCl2 treatment was bound by the soil materials, in agreement with the XANES results.
Table 2 Copper speciation determined by X-ray absorption near edge structure (XANES) spectroscopy after 70 days in soils treated with 1000 mg Cu per kg soil using CuCl2 (n = 4), 16nCuO (n = 4), 42nCuO (n = 4), and bCuO (bCuO, n = 2). Soils (0–10 cm depth) were sampled from the conventional (Con) and organic (Org) agricultural treatment plots, and from adjacent restored grassland (Gra) and riparian forest (For) at the Russell Ranch Sustainable Agricultural Facility
|
Normalized sum of squares |
CuO |
Soil bound Cu |
Sum |
(10−5) |
% |
% |
% |
Means separation for copper speciation was analyzed by Tukey's HSD with significance declared at p ≤ 0.05 and differences among treatments within the same column are denoted with lowercase letters.
|
CuCl2 |
2.5 ± 0.3 |
0 ± 0a |
101 ± 1a |
100 ± 1 |
16nCuO |
3.1 ± 1.8 |
0 ± 0a |
100 ± 1a |
100 ± 2 |
42nCuO |
3.4 ± 1.8 |
46 ± 5b |
53 ± 5b |
99 ± 1 |
bCuO |
2.5 ± 1.8 |
60 ± 0.4c |
41 ± 7c |
101 ± 0 |
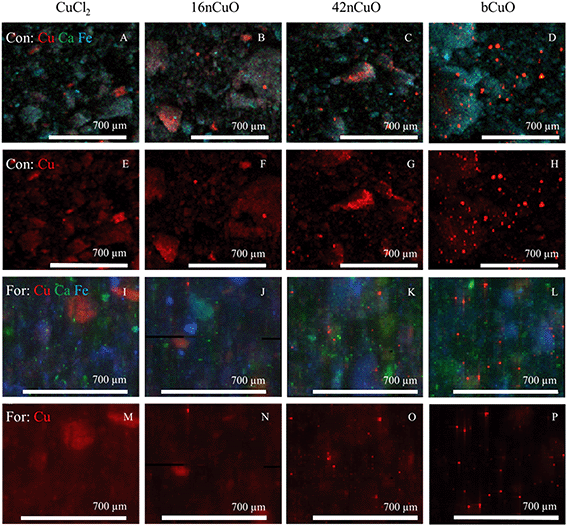 |
| Fig. 4 X-ray fluorescence distribution maps of Cu, Ca, and Fe in soil under the conventional treatment (Con) treated with 1000 mg copper (Cu) per kg soil from A) CuCl2, B) 16nCuO, C) 42nCuO, D) bCuO; same maps of Con soil with only Cu visible from E) CuCl2, F) 16nCuO, G) 42nCuO, H) bCuO taken on XFM 10.3.2 at the ALS; X-ray fluorescence distribution maps of Cu, Ca, and Fe from the forest soil (For) treated with 1000 mg copper (Cu) per kg soil from I) CuCl2, J) 16nCuO, K) 42nCuO, or L) bCuO; same maps of For soil with only Cu visible from M) CuCl2, N) 16nCuO, O) 42nCuO, or P) bCuO collected on XFM VESPERS at the CLS; increased red saturation is indicative of increased localized Cu content. | |
Copper speciation in soils treated with 16nCuO was similar to that of soils treated with CuCl2, with no copper remaining as CuO after 70 days (Tables 2 and S8†). Rather, the copper was bound by soil materials, primarily adsorbed by silicates, and complexed by soil organic matter. In XFM maps of both the Con and For soils treated with 16nCuO, individual soil particles were visible in both maps using the Cu, Ca, and Fe fluorescence channels (Fig. 4B and J) as well as the Cu channel alone (Fig. 4F and N). Few aggregates of Cu appear to remain as bright Cu points in the map, with copper evenly distributed among the individual soil particles. Given the XANES results for the 16nCuO treatment and the fact that ligands in the soil solution would promote nanoparticle aggregation, the mapping results provide further evidence of 16nCuO dissolution.91
After treatment with 42nCuO, Cu was measured by Cu XANES to be primarily bound by soil materials, with some Cu remaining as CuO (Table S8†). The amount of CuO remaining after the 70 day incubation was significantly greater than was measured after treatment with 16nCuO, but significantly less than was measured after treatment with bCuO. Soil particles are visible in tricolor Cu, Ca, Fe X-ray fluorescence distribution maps (Fig. 4C and K) as well as the Cu map (Fig. 4G and O) in both the Con and For soils, evidence of some CuO dissolution and subsequent adsorption/complexation by soil materials. Scattered aggregates of Cu are clearly visible in both soils, indicative of incomplete dissolution.
As measured by Cu XANES, copper in soils treated with bCuO remained primarily as CuO with some dissolution having occurred. Significantly more CuO was measured after 70 days in soils treated with bCuO than after treatment with 16nCuO or 42nCuO (Tables 2 and S8†). Soil particles in both the Con and For soils are visible in tricolor Cu, Ca, Fe X-ray fluorescence distribution maps (Fig. 4D and L), but barely visible in Cu maps (Fig. 4H and P), evidence of limited Cu adsorption/complexation by soil particles. Rather, most of the copper appears as aggregates of copper in the maps.
Regardless of soil type, CuO particle dissolution was controlled by particle size. After 70 days, soils treated with 16nCuO had no remaining CuO. Rather, all Cu spectra could be modeled either as Cu adsorbed by silicates and complexed by organic matter. Soils treated with 42nCuO had more remaining CuO than those receiving the 16nCuO treatment, but less than those receiving the bCuO treatment (Tables 2 and S8,† Fig. S3†).
Most studies do not include Cu adsorbed to phyllosilicate minerals commonly found in soils from arid or Mediterranean climates or those that have undergone little pedogenisis.27,33,103,108–116 Rather, Cu from CuO nanoparticles is often modeled as complexed by SOM, neoformed as CuxS, or adsorbed by iron or aluminum oxides.27,33,109,116 However, reducing conditions such as those that lead to CuxS formation are less likely to occur in soils from arid or Mediterranean climates.107,113 Further, soils in these climates, and more generally, soils that have undergone minimal mineral weathering, are dominated by phyllosilicate minerals and are unlikely to contain significant amounts of iron or aluminum oxides.107,113,114 As a result, the general reporting and understanding of soil organic matter complexation, sulfide formation, or adsorption by iron and aluminum oxides as the main fate of Cu added to soils may be incomplete.27,103,108–110 Our results suggest that adsorption by silicates is the primary sink for Cu in alkaline soils. Further, our results imply that size-dependent dissolution rates may entail distinct kinetic and thus thermodynamic fates of CuO fungicides compared to larger particulate formulations. These results are important given the wide use of copper fungicides in agriculture and high probability that they end up in contact with soils after application.
Influence of CuCl2, nCuO and bCuO on soil respiration, microbial biomass, and biomass normalized respiration
Land use exerted a strong control over soil organic carbon and therefore soil microbial biomass (Fig. S4†).117–119 The unmanaged soils had the greatest total microbial biomass, with greater biomass in the For system than the Gra system (Fig. 5A, Table S9†). Among the agricultural soils, the Org soil had greater microbial biomass than the Con soil (Fig. 5A, Table S9†). This is due to the long-term addition of compost and cover crops grown in the Org soil which act to increase soil carbon, the main substrate for microbial growth in soils.38 While total microbial biomass was well correlated with soil organic carbon (SOC), the Gra system exhibited statistically higher biomass per unit SOC (0.33 ± 0.003 μmol PLFA mol−1 SOC-C) compared to the Con (0.28 ± 0.003 μmol PLFA mol−1 SOC-C), Org (0.28 ± 0.008 μmol PLFA mol−1 SOC-C), or For (0.26 ± 0.007 μmol PLFA mol−1 SOC-C) systems (Table 1, Fig. 5A, Table S9,† Fig. S4†).
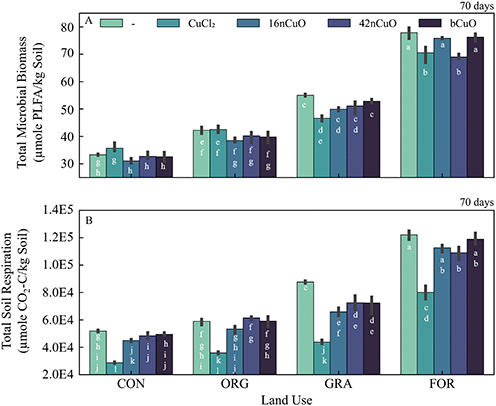 |
| Fig. 5 A) Total soil PLFAs after 70 day, B) cumulative (70 day) soil respiration in soils from conventional (Con), organic (Org), grassland (Gra), and forest (For) systems untreated (—) or treated with 1000 mg copper Cu per kg soil in the form of CuCl2, 16nCuO, 42nCuO, or bCuO. Data are presented as mean ± standard deviation (n = 3). Means separation was analyzed by Tukey's HSD with significance declared at p ≤ 0.05 and differences are denoted with differing lower-case letters. | |
The response of soil microbial biomass to treatment with copper depended on the combination of land use and Cu form (F9,30 = 9.2, p < 0.0001) (Fig. 5A, Table S9†). Land use had a larger effect on soil microbial biomass (F3,30 = 1400, p < 0.0001) than Cu form (F3,30 = 3.9, p = 0.0192). The 42nCuO and CuCl2 treatments significantly decreased total PLFAs in the For and Gra soils compared to their respective untreated controls (Fig. 5A, Table S9†). Total PLFA content of Con and Org soils was not significantly altered 70 days after copper treatment, regardless of form (Fig. 5A, Table S9†). Though unexpected, the overriding effects of previous land use on soil microbial biomass to Cu exposure help explain the diverse microbial responses to various nanomaterials, including nCuO observed in this and other studies.18,20,22,26,120 Specifically, Collins et al., 2012, found no change to the fatty acid profile of soil microbial communities after treatment with Cu nanoparticles, but this may have been because the soil they used came from an agricultural system which our results suggest are insensitive to Cu treatment in general and CuO nanoparticle treatment in particular. Ge et al., 2011, measured significant changes in soil microbial community as estimated by total extractable DNA in grassland soils treated with TiO2 and ZnO nanoparticles, but this may have been due to the selection of a grassland soil for experimentation which our results suggest are sensitive to nanoparticle treatment.26 Further, Simonin et al., 2015, noted that in their experiment to evaluate the effects of TiO2 nanoparticles on soil microbial communities in soils of contrasting textures that the only soil that was responsive to nanoparticle exposure was a silty-clay-loam, a result they found surprising. However, this soil was taken from a pasture, one of only two soils (out of six) in their study that was not cropped. Our results suggest that the response they measured may be attributed to historical use as pasture, which is more similar to unmanaged systems compared to cropped systems, rather than an intrinsic property of the soil itself.19 Similarly, Simonin et al., 2018, measured limited effects of CuO NPs on microbial abundance in agricultural soils treated with CuSO4 and CuO nanoparticles, but did observe a decrease in soil microbial activity in some soils, in agreement with the results of the current study.20
Soil respiration increased with SOC content, and the unmanaged soils accordingly had greater total respiration compared to the agricultural soils (Fig. 5B and S10†). Similar to total microbial biomass, total respiration per unit SOC was uniquely high for Gra soils (510 ± 6.8 μmol CO2-C per mol SOC-C) compared to the Con (440 ± 4 μmol CO2-C per mol SOC-C), For (400 ± 10 μmol CO2-C per mol SOC-C), or Org (380 ± 18 μmol CO2-C per mol SOC-C) systems (Table 1, Fig. 5B, Table S10†).
The response of total soil respiration to copper treatment was unique to the combination of land use and Cu form (F9,30 = 3.9, p = 0.0024), but was more strongly influenced by land use (F3,30 = 700, p < 0.0001) than Cu form (F3,30 = 160, p < 0.0001) (Fig. 5B, Table S10†). Treatment with 1000 mg Cu per kg soil from CuCl2 induced a decrease in total soil respiration across all treatments (Fig. 5B, Table S10†). Treatment with 42nCuO decreased total respiration in soils from the For and Gra systems, but not in soils under agricultural use. Both 16nCuO and bCuO inhibited respiration from the Gra soil, but not from soils in any of the other systems (Fig. 5B, Table S10†). Treatment with >100 mg CuO NPs per kg soil was previously observed to alter soil microbial activity and substrate induced respiration, but the effects of nCuO on continuous respiration processes were not previously measured.18–20 Interestingly, Simonin et al., 2018 measured that in agricultural soils, exposure to Cu from CuSO4 and CuO nanoparticles caused inconsistent alterations to substrate induced respiration after 7 and 90 days. Out of six soils, only one soil exhibited altered substrate induced respiration from nanoparticle exposure after 90 days and 2 soils from exposure to CuSO4. This contrasts with the current study in which CuCl2 inhibited respiration in all soils over the course of 70 days. This difference may be due to the relatively high concentration of Cu used in the current study (1000 mg Cu per kg soil) compared to that used by Simonin et al., 2018 (100 mg Cu per kg soil).
Biomass normalized respiration (BNR) was used to evaluate SOC conversion efficiency, a proxy for system stress/activity similar to the specific respiration rate or respiratory quotient (Fig. 6A, Table S11†).121,122 Among the untreated controls, soil BNR was lowest for Org and similar for the other three land uses (Fig. 6A, Table S10†). In soils treated with copper, soil BNR response was primarily influenced by Cu form (F3,30 = 120, p < 0.0001) and to a lesser extent, land use (F3,30 = 9.5, p < 0.0001), with little interaction (F9,30 = 2.1, p = 0.0586) (Fig. 6A, Table S11†). Specifically, BNR was suppressed by treatment with CuCl2, indicative of stress (Fig. 6A, Table S11†).121,122 Sereni et al. 2021 reported contradictory results in their recent meta-analysis. Specifically, they found that Cu contamination was associated with increased biomass normalized respiration rates, but this was in acidic soils.123 In alkaline soils, like those used in the current study, they did not find a linkage between biomass normalized respiration and Cu contamination.123 However, Badía and Martí, 2003, previously observed that stress inhibits biomass normalized respiration in alkaline soils.122 Given that treatment with CuO greatly increased soil pH compared to treatment with CuCl2 and increased available Cu less than CuCl2, it would be expected that treatment with CuO, regardless of size, would have minimal effect on biomass normalized respiration compared to treatment with CuCl2.122,123
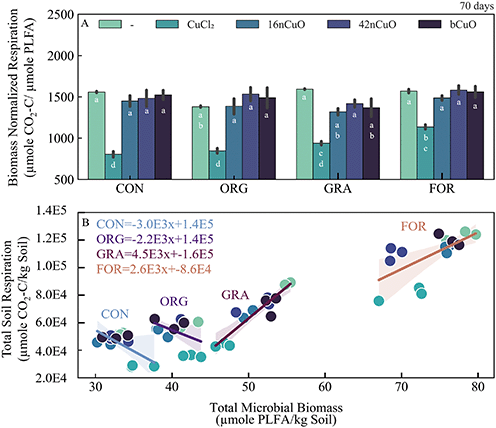 |
| Fig. 6 A) Biomass normalized respiration (μmol CO2-C/μmol PLFA) for untreated soils (—) or after treatment with 1000 mg Cu per kg soil in the form of CuCl2, 16nCuO, 42nCuO, or bCuO. Data are presented as mean ± standard deviation (n = 3). Means separation was analyzed by Tukey's HSD with significance declared at p ≤ 0.05 and differences are denoted with differing lower-case letters; B) total soil respiration plotted against total PLFAs and analyzed by linear regression. | |
Interestingly, divergent responses to general Cu treatment were observed among the soils. Specifically, respiration and microbial biomass were coupled in the For soil and decoupled in the Con and Org soils. These distinct relationships between respiration and microbial biomass by land use are particularly apparent when respiration was plotted against microbial biomass for all Cu treatments. In soils from both the For (r2 = 0.42, p = 0.0088) and Gra systems (r2 = 0.86, p ≤ 0.0001), increasing Cu content is associated with both decreased respiration and microbial biomass, leading to positive linear regression fit slopes (Fig. 6B). In soils from the Org (r2 = 0.2, p = 0.1104) and Con systems (r2 = 0.4, p = 0.0155), increasing Cu content was associated with lower respiration but little or positive change in microbial biomass, leading to negative linear regression fit slopes, and indicative of greater metal stress tolerance (Fig. 6B).124
Surprisingly, we failed to reject the null hypothesis that increasing SOC would confer greater protection to soil microbial communities from copper exposure as was previously observed by Rousk et al., 2012. Rather our results were similar to Sominin et al., 2018, who measured no clear linkage between increasing SOC and microbial resilience to copper exposure. In the current study, land use history was seemingly more important than SOC given the divergent response of the microbial communities in Org and Gra systems to Cu exposure despite similarities in SOC content. A practical implication of this for nanoparticle toxicity assays is that the effects of nCuO on microbial communities may be underestimated if only agricultural soils are used as the test media.
Conclusions
The fate of copper in soil was dependent upon the form and size of the Cu involved. As revealed by XFM and XANES spectroscopy, CuO underwent size dependent dissolution, corroborated by greater increases in available Cu for soils treated with smaller nanoparticulate CuO. Addition of CuCl2 acidified the soil through precipitation and hydrolysis reactions. In contrast, additions of CuO raised the soil pH within 1 h, but after 70 days decreased the soil pH, potentially due to respiration related acidification.
Though Cu form strongly affected chemical properties such as soil pH and available soil-Cu, land use dominated the response of microbial response to Cu treatment, often resulting in responses unique to the combination of land use and Cu form. Microbial biomass in the unmanaged systems was sensitive to Cu amendment, especially in the Gra soil that had the greatest respiration (activity) and microbial biomass per unit SOC. Microbial biomass in conventional and organic systems was unaltered by Cu exposure; but respiration was inhibited in all soils by CuCl2 at 1000 mg Cu per kg soil. This suggests that microbially mediated nutrient cycling, important for facilitating nutrient availability, particularly in organic agricultural systems, is vulnerable to copper exposure even with minor alteration of microbial biomass size. However, our study demonstrates that novel nCuO biocides have fewer deleterious effects on soil microbial communities compared to salt-based Cu treatments, at least in the short-term (70 days), due to comparatively slower dissolution and minimal soil pH disturbance.
Additionally, the unique relationship between soil respiration and microbial biomass after copper treatment within each land use underscores that microbial responses to Cu amendment are often more sensitive to historical use than Cu treatment rate or form. Thus, it is important to consider historical land use when interpreting soil Cu nanoparticle toxicity testing results and potentially, metal and nanoparticle toxicity results in general.
Conflicts of interest
There are no conflicts to declare.
Acknowledgements
We thank the reviewers of this manuscript for their efforts and constructive feedback. We thank Taylor Edward Kenneth Strehl, Rafael De Souza Cavassani, Michael Schmidt, Gurbir Singh Dhillon, and Ruan Francisco Firmano for help collecting XANES data at the Canadian Light Source. We are grateful to the Canadian Light Source (Project: 28G09585) and the Advanced Light Source (ALS-10032) for access to beamlines XFM 10.3.2, VESPERS, and HXMA. We are grateful to Greg Lowry and Andreas Voegelin for sharing Cu XANES spectra of various Cu compounds with us to confirm our Cu standard results. The authors also thank Fei Guo at the Biological Electron Microscopy Facility at UC Davis for his help with electron microscopy. This material is based upon work that was supported by the National Institute of Food and Agriculture, U.S. Department of Agriculture, under award number Grant #2013-67017-21211. Additional funds were graciously provided by the United States Department of Agriculture Agricultural Research Service CRIS project #438281 and the Department of Energy under award number Grant #DE-SC0008385. This research used resources of the Advanced Light Source, a U.S. DOE Office of Science User Facility under contract no. DE-AC02-05CH11231.
References
- V. Chaignon, I. Sanchez-Neira, P. Herrmann, B. Jaillard and P. Hinsinger, Copper bioavailability and extractability as related to chemical properties of contaminated soils from a vine-growing area, Environ. Pollut., 2003, 123, 229–238 CrossRef CAS PubMed.
- The top 100 pesticides used by pounds of active ingredients statewide in 2017 (all sites combined), California Department of Pesticide Regulation, 2020.
- L. Epstein and S. Bassein, Pesticide Applications of Copper on Perennial Crops in California, 1993 to 1998, J. Environ. Qual., 2001, 30, 1844–1847 CrossRef CAS PubMed.
- L. M. Flores-Vélez, J. Ducaroir, A. M. Jaunet and M. Robert, Study of the distribution of copper in an acid sandy vineyard soil by three different methods, Eur. J. Soil Sci., 1996, 47, 523–532 CrossRef.
- N. Mirlean, A. Roisenberg and J. O. Chies, Metal contamination of vineyard soils in wet subtropics (southern Brazil), Environ. Pollut., 2007, 149, 10–17 CrossRef CAS PubMed.
- S. Ruyters, P. Salaets, K. Oorts and E. Smolders, Copper toxicity in soils under established vineyards in Europe: A survey, Sci. Total Environ., 2013, 443, 470–477 CrossRef CAS PubMed.
- A. M. Wightwick, M. R. Mollah, D. L. Partington and G. Allinson, Copper Fungicide Residues in Australian Vineyard Soils, J. Agric. Food Chem., 2008, 56, 2457–2464 CrossRef CAS PubMed.
- Walnut Blight/Walnut/Agriculture: Pest Management Guidelines/UC Statewide IPM Program (UC IPM), https://www2.ipm.ucanr.edu/agriculture/walnut/Walnut-Blight/, (accessed 22 May 2020).
-
P. Darriet, P. Bouchilloux, C. Poupot, Y. Bugaret, M. Clerjeau, P. Sauris, B. Medina and D. Dubourdieu, Effects of copper fungicide spraying on volatile thiols of the varietal aroma of Sauvignon blanc, Cabernet Sauvignon and Merlot wines, VITIS-GEILWEILERHOF, University of California Agriculture & Natural Resources, 2001, vol. 40, pp. 93–100 Search PubMed.
- G. Pergher and R. Gubiani, The Effect of Spray Application Rate and Airflow Rate on Foliar Deposition in a Hedgerow Vineyard, J. Agric. Eng. Res., 1995, 61, 205–216 CrossRef.
- G. Pergher, R. Gubiani and G. Tonetto, Foliar deposition and pesticide losses from three air-assisted sprayers in a hedgerow vineyard, Crop Prot., 1997, 16, 25–33 CrossRef.
- K. A. Mackie, T. Müller and E. Kandeler, Remediation of copper in vineyards – A mini review, Environ. Pollut., 2012, 167, 16–26 CrossRef CAS PubMed.
- W. H. Elmer and J. C. White, The use of metallic oxide nanoparticles to enhance growth of tomatoes and eggplants in disease infested soil or soilless medium, Environ. Sci.: Nano, 2016, 3, 1072–1079 RSC.
- S. Frenk, T. Ben-Moshe, I. Dror, B. Berkowitz and D. Minz, Effect of Metal Oxide Nanoparticles on Microbial Community Structure and Function in Two Different Soil Types, PLoS One, 2013, 8, e84441 CrossRef PubMed.
- P. Doelman and L. Haanstra, Short-term and long-term effects of cadmium, chromium, copper, nickel, lead and zinc on soil microbial respiration in relation to abiotic soil factors, Plant Soil, 1984, 79, 317–327 CrossRef CAS.
- A. Avellan, M. Simonin, S. M. Anderson, N. K. Geitner, N. Bossa, E. Spielman-Sun, E. S. Bernhardt, B. T. Castellon, B. P. Colman, J. L. Cooper, M. Ho, M. F. Hochella, H. Hsu-Kim, S. Inoue, R. S. King, S. Laughton, C. W. Matson, B. G. Perrotta, C. J. Richardson, J. M. Unrine, M. R. Wiesner and G. V. Lowry, Differential Reactivity of Copper- and Gold-Based Nanomaterials Controls Their Seasonal Biogeochemical Cycling and Fate in a Freshwater Wetland Mesocosm, Environ. Sci. Technol., 2020, 54, 1533–1544 CrossRef CAS PubMed.
- H. Ashraf, T. Anjum, S. Riaz, I. S. Ahmad, J. Irudayaraj, S. Javed, U. Qaiser and S. Naseem, Inhibition mechanism of green-synthesized copper oxide nanoparticles from Cassia fistula towards Fusarium oxysporum by boosting growth and defense response in tomatoes, Environ.
Sci.: Nano, 2021, 8, 1729–1748 RSC.
- R. W. Lewis, P. M. Bertsch and D. H. McNear, Nanotoxicity of engineered nanomaterials (ENMs) to environmentally relevant beneficial soil bacteria – a critical review, Nanotoxicology, 2019, 13, 392–428 CrossRef CAS PubMed.
- M. Simonin and A. Richaume, Impact of engineered nanoparticles on the activity, abundance, and diversity of soil microbial communities: a review, Environ. Sci. Pollut. Res., 2015, 22, 13710–13723 CrossRef CAS PubMed.
- M. Simonin, A. A. Cantarel, A. Crouzet, J. Gervaix, J. M. Martins and A. Richaume, Negative effects of copper oxide nanoparticles on carbon and nitrogen cycle microbial activities in contrasting agricultural soils and in presence of plants, Front. Microbiol., 2018, 9, 3102 CrossRef PubMed.
- A. K. Alva, J. H. Graham and C. A. Anderson, Soil pH and Copper Effects on Young ‘Hamlin’ Orange Trees, Soil Sci. Soc. Am. J., 1995, 59, 481–487 CrossRef CAS.
- D. Collins, T. Luxton, N. Kumar, S. Shah, V. K. Walker and V. Shah, Assessing the Impact of Copper and Zinc Oxide Nanoparticles on Soil: A Field Study, PLoS One, 2012, 7, e42663 CrossRef CAS PubMed.
- B. Asadishad, S. Chahal, A. Akbari, V. Cianciarelli, M. Azodi, S. Ghoshal and N. Tufenkji, Amendment of Agricultural Soil with Metal Nanoparticles: Effects on Soil Enzyme Activity and Microbial Community Composition, Environ. Sci. Technol., 2018, 52, 1908–1918 CrossRef CAS PubMed.
- C. P. Rooney, F.-J. Zhao and S. P. McGrath, Soil factors controlling the expression of copper toxicity to plants in a wide range of European soils, Environ. Toxicol. Chem., 2006, 25, 726–732 CrossRef CAS PubMed.
- Q.-Y. Wang, D.-M. Zhou and L. Cang, Microbial and enzyme properties of apple orchard soil as affected by long-term application of copper fungicide, Soil Biol. Biochem., 2009, 41, 1504–1509 CrossRef CAS.
- Y. Ge, J. P. Schimel and P. A. Holden, Evidence for Negative Effects of TiO 2 and ZnO Nanoparticles on Soil Bacterial Communities, Environ. Sci. Technol., 2011, 45, 1659–1664 CrossRef CAS PubMed.
- C. Peng, C. Xu, Q. Liu, L. Sun, Y. Luo and J. Shi, Fate and Transformation of CuO Nanoparticles in the Soil–Rice System during the Life Cycle of Rice Plants, Environ. Sci. Technol., 2017, 51, 4907–4917 CrossRef CAS PubMed.
- A. J. Margenot, D. A. Rippner, M. R. Dumlao, S. Nezami, P. G. Green, S. J. Parikh and A. J. McElrone, Copper oxide nanoparticle effects on root growth and hydraulic conductivity of two vegetable crops, Plant Soil, 2018, 431, 333–345 CrossRef CAS.
- J. Rousk, K. Ackermann, S. F. Curling and D. L. Jones, Comparative toxicity of nanoparticulate CuO and ZnO to soil bacterial communities, PLoS One, 2012, 7(3), e34197 CrossRef CAS PubMed.
- J. R. Velicogna, D. M. Schwertfeger, C. Beer, A. H. Jesmer, J. Kuo, H. Chen, R. P. Scroggins and J. I. Princz, Phytotoxicity of copper oxide nanoparticles in soil with and without biosolid amendment, NanoImpact, 2020, 17, 100196 CrossRef.
- A. Tessier, P. G. Campbell and M. Bisson, Sequential extraction procedure for the speciation of particulate trace metals, Anal. Chem., 1979, 51, 844–851 CrossRef CAS.
- G. Rauret, J. F. Lopez-Sanchez, A. Sahuquillo, R. Rubio, C. Davidson, A. Ure and P. Quevauviller, Improvement of the BCR three step sequential extraction procedure prior to the certification of new sediment and soil reference materials, J. Environ. Monit., 1999, 1, 57–61 RSC.
- R. Sekine, E. R. Marzouk, M. Khaksar, K. G. Scheckel, J. P. Stegemeier, G. V. Lowry, E. Donner and E. Lombi, Aging of Dissolved Copper and Copper-based Nanoparticles in Five Different Soils: Short-term Kinetics vs. Long-term Fate, J. Environ. Qual., 2017, 46, 1198–1205 CrossRef CAS PubMed.
- C. Xu, C. Peng, L. Sun, S. Zhang, H. Huang, Y. Chen and J. Shi, Distinctive effects of TiO 2 and CuO nanoparticles on soil microbes and their community structures in flooded paddy soil, Soil Biol. Biochem., 2015, 86, 24–33 CrossRef CAS.
- C. Peng, D. Duan, C. Xu, Y. Chen, L. Sun, H. Zhang, X. Yuan, L. Zheng, Y. Yang, J. Yang, X. Zhen, Y. Chen and J. Shi, Translocation and biotransformation of CuO nanoparticles in rice (Oryza sativa L.) plants, Environ. Pollut., 2015, 197, 99–107 CrossRef CAS PubMed.
- L. A. Mendes, M. J. B. Amorim and J. J. Scott-Fordsmand, Interactions of Soil Species Exposed to CuO NMs are Different From Cu Salt: A Multispecies Test, Environ. Sci. Technol., 2018, 52, 4413–4421 CrossRef CAS PubMed.
- D. E. Beaudette and A. T. O'Geen, Soil-Web: an online soil survey for California, Arizona, and Nevada, Comput. Geosci., 2009, 35, 2119–2128 CrossRef.
- N. E. Tautges, J. L. Chiartas, A. C. M. Gaudin, A. T. O'Geen, I. Herrera and K. M. Scow, Deep soil inventories reveal that impacts of cover crops and compost on soil carbon sequestration differ in surface and subsurface soils, Glob. Change Biol., 2019, 25, 3753–3766 CrossRef PubMed.
- A. J. Margenot, Y. Nakayama and S. J. Parikh, Methodological recommendations for optimizing assays of enzyme activities in soil samples, Soil Biol. Biochem., 2018, 125, 350–360 CrossRef CAS.
- J. Wade, H. Waterhouse, L. M. Roche and W. R. Horwath, Structural equation modeling reveals iron (hydr)oxides as a strong mediator of N mineralization in California agricultural soils, Geoderma, 2018, 315, 120–129 CrossRef CAS.
- T. A. Doane and W. R. Horwáth, Spectrophotometric Determination of Nitrate with a Single Reagent, Anal. Lett., 2003, 36, 2713–2722 CrossRef CAS.
- K. T. Revell, R. O. Maguire and F. A. Agblevor, Influence of Poultry Litter Biochar on Soil Properties and Plant Growth, Soil Sci., 2012, 177, 402–408 CrossRef CAS.
-
S. R. Olsen, Estimation of available phosphorus in soils by extraction with sodium bicarbonate, US Department of Agriculture, 1954 Search PubMed.
- D. A. Rippner, J. Lien, H. Balla, T. Guo, P. G. Green, T. M. Young and S. J. Parikh, Surface modification induced cuprous oxide nanoparticle toxicity to duckweed at sub-toxic metal concentrations, Sci. Total Environ., 2020, 137607 CrossRef CAS PubMed.
- M. A. Siddiqui, H. A. Alhadlaq, J. Ahmad, A. A. Al-Khedhairy, J. Musarrat and M. Ahamed, Copper oxide nanoparticles induced mitochondria mediated apoptosis in human hepatocarcinoma cells, PLoS One, 2013, 8, e69534 CrossRef CAS PubMed.
- J. Schindelin, I. Arganda-Carreras, E. Frise, V. Kaynig, M. Longair, T. Pietzsch, S. Preibisch, C. Rueden, S. Saalfeld, B. Schmid, J.-Y. Tinevez, D. J. White, V. Hartenstein, K. Eliceiri, P. Tomancak and A. Cardona, Fiji: an open-source platform for biological-image analysis, Nat. Methods, 2012, 9, 676–682 CrossRef CAS PubMed.
- D. Geisseler, W. R. Horwath and T. A. Doane, Significance of organic nitrogen uptake from plant residues by soil microorganisms as affected by carbon and nitrogen availability, Soil Biol. Biochem., 2009, 41, 1281–1288 CrossRef CAS.
- J. Wade, W. R. Horwath and M. B. Burger, Integrating Soil Biological and Chemical Indices to Predict Net Nitrogen Mineralization across California Agricultural Systems, Soil Sci. Soc. Am. J., 2016, 80, 1675–1687 CrossRef CAS.
- F. N. D. Mukome, J. Six and S. J. Parikh, The effects of walnut shell and wood feedstock biochar amendments on greenhouse gas emissions from a fertile soil, Geoderma, 2013, 200–201, 90–98 CrossRef CAS.
- D. G. Strawn and L. L. Baker, Speciation of Cu in a Contaminated Agricultural
Soil Measured by XAFS, μ-XAFS, and μ-XRF, Environ. Sci. Technol., 2008, 42, 37–42 CrossRef CAS PubMed.
- J. L. Freeman, S. D. Lindblom, C. F. Quinn, S. Fakra, M. A. Marcus and E. A. H. Pilon-Smits, Selenium accumulation protects plants from herbivory by Orthoptera via toxicity and deterrence, New Phytol., 2007, 175, 490–500 CrossRef CAS PubMed.
- M. A. Marcus, A. A. MacDowell, R. Celestre, A. Manceau, T. Miller, H. A. Padmore and R. E. Sublett, Beamline 10.3. 2 at ALS: a hard X-ray microprobe for environmental and materials sciences, J. Synchrotron Radiat., 2004, 11, 239–247 CrossRef CAS PubMed.
-
R. Feng, A. Gerson, G. Ice, R. Reininger, B. Yates and S. McIntyre, in AIP Conference Proceedings, American Institute of Physics, 2007, vol. 879, pp. 872–874 Search PubMed.
- A. Manceau, K. L. Nagy, M. A. Marcus, M. Lanson, N. Geoffroy, T. Jacquet and T. Kirpichtchikova, Formation of Metallic Copper Nanoparticles at the Soil−Root Interface, Environ. Sci. Technol., 2008, 42, 1766–1772 CrossRef CAS PubMed.
-
D. T. Jiang, N. Chen and W. Sheng, in AIP Conference Proceedings, American Institute of Physics, 2007, vol. 879, pp. 800–803 Search PubMed.
- J. Yang, T. Regier, J. J. Dynes, J. Wang, J. Shi, D. Peak, Y. Zhao, T. Hu, Y. Chen and J. S. Tse, Soft X-ray Induced Photoreduction of Organic Cu(II) Compounds Probed by X-ray Absorption Near-Edge (XANES) Spectroscopy, Anal. Chem., 2011, 83, 7856–7862 CrossRef CAS PubMed.
- L. J. Furnare, A. Vailionis and D. G. Strawn, Polarized XANES and EXAFS spectroscopic investigation into copper (II) complexes on vermiculite, Geochim. Cosmochim. Acta, 2005, 69, 5219–5231 CrossRef CAS.
- T. Karlsson, P. Persson and U. Skyllberg, Complexation of Copper(II) in Organic Soils and in Dissolved Organic Matter − EXAFS Evidence for Chelate Ring Structures, Environ. Sci. Technol., 2006, 40, 2623–2628 CrossRef CAS PubMed.
- Z. He, C. W. Honeycutt, T. Zhang and P. M. Bertsch, Preparation and FT–IR Characterization of Metal Phytate Compounds, J. Environ. Qual., 2006, 35, 1319–1328 CrossRef CAS PubMed.
- S. Kraft, J. Stümpel, P. Becker and U. Kuetgens, High resolution x-ray absorption spectroscopy with absolute energy calibration for the determination of absorption edge energies, Rev. Sci. Instrum., 1996, 67, 681–687 CrossRef CAS.
- F. Panfili, A. Manceau, G. Sarret, L. Spadini, T. Kirpichtchikova, V. Bert, A. Laboudigue, M. A. Marcus, N. Ahamdach and M.-F. Libert, The effect of phytostabilization on Zn speciation in a dredged contaminated sediment using scanning electron microscopy, X-ray fluorescence, EXAFS spectroscopy, and principal components analysis, Geochim. Cosmochim. Acta, 2005, 69, 2265–2284 CrossRef CAS.
- A. Manceau and A. Matynia, The nature of Cu bonding to natural organic matter, Geochim. Cosmochim. Acta, 2010, 74, 2556–2580 CrossRef CAS.
- B. Ravel and M. Newville, Athena, Artemis, Hephaestus: data analysis for X-ray absorption spectroscopy using IFEFFIT, J. Synchrotron Radiat., 2005, 12, 537–541 CrossRef CAS PubMed.
- Å. Frostegård, E. Bååth and A. Tunlio, Shifts in the structure of soil microbial communities in limed forests as revealed by phospholipid fatty acid analysis, Soil Biol. Biochem., 1993, 25, 723–730 CrossRef.
- C. T. Green and K. M. Scow, Analysis of phospholipid fatty acids (PLFA) to characterize microbial communities in aquifers, Hydrogeol. J., 2000, 8, 126–141 CrossRef CAS.
- D. A. Bossio and K. M. Scow, Impacts of Carbon and Flooding on Soil Microbial Communities: Phospholipid Fatty Acid Profiles and Substrate Utilization Patterns, Microb. Ecol., 1998, 35, 265–278 CrossRef CAS PubMed.
- D. A. Bossio, K. M. Scow, N. Gunapala and K. J. Graham, Determinants
of Soil Microbial Communities: Effects of Agricultural Management, Season, and Soil Type on Phospholipid Fatty Acid Profiles, Microb. Ecol., 1998, 36, 1–12 CrossRef CAS PubMed.
- R. E. Drenovsky, G. N. Elliott, K. J. Graham and K. M. Scow, Comparison of phospholipid fatty acid (PLFA) and total soil fatty acid methyl esters (TSFAME) for characterizing soil microbial communities, Soil Biol. Biochem., 2004, 36, 1793–1800 CrossRef CAS.
- Å. Frostegård, A. Tunlid and E. Bååth, Use and misuse of PLFA measurements in soils, Soil Biol. Biochem., 2011, 43, 1621–1625 CrossRef.
- W. McKinney, pandas: a foundational Python library for data analysis and statistics, Python High-Perform. Sci. Comput., 2011, 14, 1–9 Search PubMed.
- J. D. Hunter, Matplotlib: A 2D graphics environment, IEEE Ann. Hist. Comput, 2007, 9, 90–95 Search PubMed.
- M. L. Waskom, Seaborn: statistical data visualization, J. Open Source Softw, 2021, 6, 3021 CrossRef.
- Fertilizer and Compost Application amount, https://asi.ucdavis.edu/programs/rr/research/data.
- S. Thakali, H. E. Allen, D. M. Di Toro, A. A. Ponizovsky, C. P. Rooney, F.-J. Zhao and S. P. McGrath, A Terrestrial Biotic Ligand Model. 1. Development and Application to Cu and Ni Toxicities to Barley Root Elongation in Soils, Environ. Sci. Technol., 2006, 40, 7085–7093 CrossRef CAS PubMed.
- A. Sadeghpour, Q. M. Ketterings, F. Vermeylen, G. S. Godwin and K. J. Czymmek, Soil properties under nitrogen-vs. phosphorus-based manure and compost management of corn, Soil Sci. Soc. Am. J., 2016, 80, 1272–1282 CrossRef.
- X. Cao, A. Wahbi, L. Ma, B. Li and Y. Yang, Immobilization of Zn, Cu, and Pb in contaminated soils using phosphate rock and phosphoric acid, J. Hazard. Mater., 2009, 164, 555–564 CrossRef CAS PubMed.
- R. X. Cao, L. Q. Ma, M. Chen, S. P. Singh and W. G. Harris, Phosphate-induced metal immobilization in a contaminated site, Environ. Pollut., 2003, 122, 19–28 CrossRef CAS PubMed.
- A. Khorsand Zak, W. H. Abd. Majid, M. E. Abrishami and R. Yousefi, X-ray analysis of ZnO nanoparticles by Williamson–Hall and size–strain plot methods, Solid State Sci., 2011, 13, 251–256 CrossRef CAS.
- E. A. Meulenkamp, Size Dependence of the Dissolution of ZnO Nanoparticles, J. Phys. Chem. B, 1998, 102, 7764–7769 CrossRef CAS.
- I. A. Mudunkotuwa, T. Rupasinghe, C.-M. Wu and V. H. Grassian, Dissolution of ZnO Nanoparticles at Circumneutral pH: A Study of Size Effects in the Presence and Absence of Citric Acid, Langmuir, 2012, 28, 396–403 CrossRef CAS PubMed.
- M. Kosmulski, pH-dependent surface charging and points of zero charge II. Update, J. Colloid Interface Sci., 2004, 275, 214–224 CrossRef CAS PubMed.
- H. L. Karlsson, P. Cronholm, Y. Hedberg, M. Tornberg, L. De Battice, S. Svedhem and I. O. Wallinder, Cell membrane damage and protein interaction induced by copper containing nanoparticles—Importance of the metal release process, Toxicology, 2013, 313, 59–69 CrossRef CAS PubMed.
- M. Geppert, L. Sigg and K. Schirmer, Toxicity and translocation of Ag, CuO, ZnO and TiO 2 nanoparticles upon exposure to fish intestinal epithelial cells, Environ. Sci.: Nano, 2021, 8, 2249–2260 RSC.
- G. A. Parks, The Isoelectric Points of Solid Oxides, Solid Hydroxides, and Aqueous Hydroxo Complex Systems, Chem. Rev., 1965, 65, 177–198 CrossRef CAS.
- G. R. Aiken, H. Hsu-Kim and J. N. Ryan, Influence of Dissolved Organic Matter on the Environmental Fate of Metals, Nanoparticles, and Colloids, Environ. Sci. Technol., 2011, 45, 3196–3201 CrossRef CAS PubMed.
- S.-W. Bian, I. A. Mudunkotuwa, T. Rupasinghe and V. H. Grassian, Aggregation and Dissolution of 4 nm ZnO Nanoparticles in Aqueous Environments: Influence of pH, Ionic Strength, Size, and Adsorption of Humic Acid, Langmuir, 2011, 27, 6059–6068 CrossRef CAS PubMed.
- C. Chen, T. Waller and S. L. Walker, Visualization of transport and fate of nano and micro-scale particles in porous media: modeling coupled effects of ionic strength and size, Environ. Sci.: Nano, 2017, 4, 1025–1036 RSC.
- L. Zehlike, A. Peters, R. H. Ellerbrock, L. Degenkolb and S. Klitzke, Aggregation of TiO2 and Ag nanoparticles in soil solution – Effects of primary nanoparticle size and dissolved organic matter characteristics, Sci. Total Environ., 2019, 688, 288–298 CrossRef CAS PubMed.
- S. Rahmatpour, M. Shirvani, M. R. Mosaddeghi and M. Bazarganipour, Retention of silver nano-particles and silver ions in calcareous soils: Influence of soil properties, J. Environ. Manage., 2017, 193, 136–145 CrossRef CAS PubMed.
- Z. Wang, J. Li, J. Zhao and B. Xing, Toxicity and Internalization of CuO Nanoparticles to Prokaryotic Alga Microcystis aeruginosa as Affected by Dissolved Organic Matter, Environ. Sci. Technol., 2011, 45, 6032–6040 CrossRef CAS PubMed.
- S. Ortelli, A. Luisa Costa, M. Blosi, A. Brunelli, E. Badetti, A. Bonetto, D. Hristozov and A. Marcomini, Colloidal characterization of CuO nanoparticles in biological and environmental media, Environ. Sci.: Nano, 2017, 4, 1264–1272 RSC.
- L. A. Brun, J. Maillet, J. Richarte, P. Herrmann and J. C. Remy, Relationships between extractable copper, soil properties and copper uptake by wild plants in vineyard soils, Environ. Pollut., 1998, 102, 151–161 CrossRef CAS.
- C. O. Dimkpa, D. E. Latta, J. E. McLean, D. W. Britt, M. I. Boyanov and A. J. Anderson, Fate of CuO and ZnO Nano- and Microparticles in the Plant Environment, Environ. Sci. Technol., 2013, 47, 4734–4742 CrossRef CAS PubMed.
- A. A. Keller, A. S. Adeleye, J. R. Conway, K. L. Garner, L. Zhao, G. N. Cherr, J. Hong, J. L. Gardea-Torresdey, H. A. Godwin, S. Hanna, Z. Ji, C. Kaweeteerawat, S. Lin, H. S. Lenihan, R. J. Miller, A. E. Nel, J. R. Peralta-Videa, S. L. Walker, A. A. Taylor, C. Torres-Duarte, J. I. Zink and N. Zuverza-Mena, Comparative environmental fate and toxicity of copper nanomaterials, NanoImpact, 2017, 7, 28–40 CrossRef.
- L. D. Baver, The Nature of Soil Buffer Action1, J. Am. Soc. Agron., 1931, 23, 587 CrossRef CAS.
- C. Pfeiffer, C. Rehbock, D. Hühn, C. Carrillo-Carrion, D. J. de Aberasturi, V. Merk, S. Barcikowski and W. J. Parak, Interaction of colloidal nanoparticles with their local environment: the (ionic) nanoenvironment around nanoparticles is different from bulk and determines the physico-chemical properties of the nanoparticles, J. R. Soc., Interface, 2014, 11, 20130931 CrossRef PubMed.
-
Y. Matsusaka and G. D. Sherman, Titration curves and buffering capacities of Hawaiian soils, University of Hawaii Technical Bulletin, 1950, vol. 11, p. 36 Search PubMed.
- J. Shi, J. Ye, H. Fang, S. Zhang and C. Xu, Effects of Copper Oxide Nanoparticles on Paddy Soil Properties and Components, Nanomaterials, 2018, 8, 839 CrossRef PubMed.
- A. S. Adeleye, S. Pokhrel, L. Mädler and A. A. Keller, Influence of nanoparticle doping on the colloidal stability and toxicity of copper oxide nanoparticles in synthetic and natural waters, Water Res., 2018, 132, 12–22 CrossRef CAS PubMed.
- M. Auffan, W. Liu, L. Brousset, L. Scifo, A. Pariat, M. Sanles, P. Chaurand, B. Angeletti, A. Thiéry, A. Masion and J. Rose, Environmental exposure of a simulated pond ecosystem to a CuO nanoparticle-based wood stain throughout its life cycle, Environ. Sci.: Nano, 2018, 5, 2579–2589 RSC.
- V. S. Sousa and M. R. Teixeira, Aggregation kinetics and surface charge of CuO nanoparticles: the influence of pH, ionic strength and humic acids, Environ. Chem., 2013, 10, 313 CrossRef CAS.
- H. Qiu and E. Smolders, Nanospecific
Phytotoxicity of CuO Nanoparticles in Soils Disappeared When Bioavailability Factors Were Considered, Environ. Sci. Technol., 2017, 51, 11976–11985 CrossRef CAS PubMed.
- X. Gao, S. M. Rodrigues, E. Spielman-Sun, S. Lopes, S. Rodrigues, Y. Zhang, A. Avellan, R. M. B. O. Duarte, A. Duarte, E. A. Casman and G. V. Lowry, Effect of Soil Organic Matter, Soil pH, and Moisture Content on Solubility and Dissolution Rate of CuO NPs in Soil, Environ. Sci. Technol., 2019, 53, 4959–4967 CrossRef CAS PubMed.
- H. V. A. McShane, G. I. Sunahara, J. K. Whalen and W. H. Hendershot, Differences in Soil Solution Chemistry between Soils Amended with Nanosized CuO or Cu Reference Materials: Implications for Nanotoxicity Tests, Environ. Sci. Technol., 2014, 48, 8135–8142 CrossRef CAS PubMed.
- R. D. Harter, Effect of Soil pH on Adsorption of Lead, Copper, Zinc, and Nickel, Soil Sci. Soc. Am. J., 1983, 47, 47–51 CrossRef CAS.
- Y. Ma, E. Lombi, I. W. Oliver, A. L. Nolan and M. J. McLaughlin, Long-Term Aging of Copper Added to Soils, Environ. Sci. Technol., 2006, 40, 6310–6317 CrossRef CAS PubMed.
- J. M. Morrison, M. B. Goldhaber, C. T. Mills, G. N. Breit, R. L. Hooper, J. M. Holloway, S. F. Diehl and J. F. Ranville, Weathering and transport of chromium and nickel from serpentinite in the Coast Range ophiolite to the Sacramento Valley, California, USA, Appl. Geochem., 2015, 61, 72–86 CrossRef CAS.
- A. D. Servin, L. Pagano, H. Castillo-Michel, R. De la Torre-Roche, J. Hawthorne, J. A. Hernandez-Viezcas, R. Loredo-Portales, S. Majumdar, J. Gardea-Torresday, O. P. Dhankher and J. C. White, Weathering in soil increases nanoparticle CuO bioaccumulation within a terrestrial food chain, Nanotoxicology, 2017, 11, 98–111 CrossRef CAS PubMed.
- X. Gao, E. Spielman-Sun, S. M. Rodrigues, E. A. Casman and G. V. Lowry, Time and Nanoparticle Concentration Affect the Extractability of Cu from CuO NP-Amended Soil, Environ. Sci. Technol., 2017, 51, 2226–2234 CrossRef CAS PubMed.
- H. Lin, J. Shi, B. Wu, J. Yang, Y. Chen, Y. Zhao and T. Hu, Speciation and biochemical transformations of sulfur and copper in rice rhizosphere and bulk soil—XANES evidence of sulfur and copper associations, J. Soils Sediments, 2010, 10, 907–914 CrossRef CAS.
- W. G. Harris, V. W. Carlisle and S. L. Chesser, Clay Mineralogy as Related to Morphology of Florida Soils with Sandy Epipedons, Soil Sci. Soc. Am. J., 1987, 51, 1673–1677 CrossRef CAS.
- K. R. Tice, R. C. Graham and H. B. Wood, Transformations of 2:1 phyllosilicates in 41-year-old soils under oak and pine, Geoderma, 1996, 70, 49–62 CrossRef CAS.
- J. L. Boettinger and R. J. Southard, Phyllosilicate Distribution and Origin in Aridisols on a Granitic Pediment, Western Mojave Desert, Soil Sci. Soc. Am. J., 1995, 59, 1189–1198 CrossRef CAS.
- P. Barré, O. Fernandez-Ugalde, I. Virto, B. Velde and C. Chenu, Impact of phyllosilicate mineralogy on organic carbon stabilization in soils: incomplete knowledge and exciting prospects, Geoderma, 2014, 235–236, 382–395 CrossRef.
- J. Parada, O. Rubilar, M. C. Diez, M. Cea, A. Sant'Ana da Silva, C. E. Rodríguez-Rodríguez and G. R. Tortella, Combined pollution of copper nanoparticles and atrazine in soil: Effects on dissipation of the pesticide and on microbiological community profiles, J. Hazard. Mater., 2019, 361, 228–236 CrossRef CAS PubMed.
- X. Guan, X. Gao, A. Avellan, E. Spielman-Sun, J. Xu, S. Laughton, J. Yun, Y. Zhang, G. D. Bland and Y. Zhang, CuO nanoparticles alter the rhizospheric bacterial community and local nitrogen cycling for wheat grown in a Calcareous soil, Environ. Sci. Technol., 2020, 54, 8699–8709 CrossRef CAS PubMed.
- K. L. Steenwerth, L. E. Jackson, F. J. Calderón, M. R. Stromberg and K. M. Scow, Soil microbial community composition and land use history in cultivated and grassland ecosystems of coastal California, Soil Biol. Biochem., 2002, 34, 1599–1611 CrossRef CAS.
- A. Y. Kong, K. M. Scow, A. L. Córdova-Kreylos, W. E. Holmes and J. Six, Microbial community composition and carbon cycling within soil microenvironments of conventional, low-input, and organic cropping systems, Soil Biol. Biochem., 2011, 43, 20–30 CrossRef CAS PubMed.
- K. L. Steenwerth, L. E. Jackson, F. J. Calderón, K. M. Scow and D. E. Rolston, Response of microbial community composition and activity in agricultural and grassland soils after a simulated rainfall, Soil Biol. Biochem., 2005, 37, 2249–2262 CrossRef CAS.
- M. Simonin, J. P. Guyonnet, J. M. Martins, M. Ginot and A. Richaume, Influence of soil properties on the toxicity of TiO2 nanoparticles on carbon mineralization and bacterial abundance, J. Hazard. Mater., 2015, 283, 529–535 CrossRef CAS PubMed.
- A. Nordgren, E. Bååth and B. Söderström, Evaluation of soil respiration characteristics to assess heavy metal effects on soil microorganisms using glutamic acid as a substrate, Soil Biol. Biochem., 1988, 20, 949–954 CrossRef CAS.
- D. Badía and C. Martí, Effect of simulated fire on organic matter and selected microbiological properties of two contrasting soils, Arid Land Res. Manage., 2003, 17, 55–69 CrossRef.
- L. Sereni, B. Guenet and I. Lamy, Does Copper Contamination Affect Soil CO2 Emissions? A Literature Review, Front. Environ. Sci., 2021, 9, 29 Search PubMed.
- M. S. McKee and J. Filser, Impacts of metal-based engineered nanomaterials on soil communities, Environ. Sci.: Nano, 2016, 3, 506–533 RSC.
Footnote |
† Electronic supplementary information (ESI) available. See DOI: 10.1039/d1en00656h |
|
This journal is © The Royal Society of Chemistry 2021 |
Click here to see how this site uses Cookies. View our privacy policy here.