Metal-doping of nanoplastics enables accurate assessment of uptake and effects on Gammarus pulex†
Received
19th January 2021
, Accepted 19th May 2021
First published on 20th May 2021
Abstract
Because of the difficulty of measuring nanoplastics (NP), the use of NPs doped with trace metals has been proposed as a promising approach to detect NP in environmental media and biota. In the present study, the freshwater amphipod Gammarus pulex were exposed to palladium (Pd)-doped NP via natural sediment at six spiking concentrations (0, 0.3, 1, 3, 10 and 30 g plastic per kg of sediment dry weight) with the aim of assessing their uptake and chronic effects using 28 days standardized single species toxicity tests. NP concentrations were quantified based on Pd concentrations measured by ICP-MS on digests of the exposed organisms and faecal pellets excreted during a post-exposure 24 hour depuration period. Additionally, NP concentrations were measured in sediments and water to demonstrate accuracy of NP dosing and to quantify the resuspension of NP from the sediment caused by the organisms. A significant positive linear relationship between the uptake of NP by G. pulex and the concentration of NP in the sediments was observed, yet no statistically significant effects were found on the survival or growth of G. pulex. A biodynamic model fitted well to the data and suggested bioaccumulation would occur in two kinetic compartments, the major one being reversible with rapid depuration to clean medium. Model fitting yielded a mass based trophic transfer factor (TTF), conceptually similar to the traditional biota sediment accumulation factor, for NP in the gut of 0.031. This value is close to a TTF value of 0.025 that was obtained for much larger microplastic particles in a similar experiment performed previously. Mechanistically, this suggests that ingestion of plastic is limited by the total volume of ingested particles. We demonstrated that using metal-doped plastics provides opportunities for precise quantification of NP accumulation and exposure in fate and effect studies, which can be a clear benefit for NP risk assessment.
Environmental significance
Contamination of the environment with plastic debris has been recognised as a major environmental problem and has caused major concerns in society. Nanoplastics (NP) is probably the least known area of marine litter but potentially also the most hazardous. Recently, the presence of small microplastic particles has been demonstrated for biota, including humans. To date, there are no analytical techniques available to adequately measure NPs in environmental samples, especially those with more difficult background matrices, like biota or sediment. Because no routine detection method exists for NPs, we are not able to assess their exposure, threshold effect concentrations and risks for human health and the environment. In response to the difficulty of measuring NP, the use of NPs doped with trace metals has been proposed as a promising approach to detect NP in environmental media and biota (Mitrano et al., 2019).41 Bioaccumulation and ecotoxicological effect studies now are possible with accurate exposure and effect assessment, using actual rather than nominal dose, by measuring the metal content in biota and exposure media. Here, we provide the first bioaccumulation and effect study using palladium (Pd)-doped NPs for the freshwater amphipod Gammarus pulex. G. pulex was exposed to Pd-doped NP via natural sediment at six spiking concentrations (0, 0.3, 1, 3, 10 and 30 g plastic per kg of sediment dry weight). NP concentrations were measured in sediments and water to demonstrate accuracy of NP dosing and to quantify the resuspension of NP from the sediment caused by the organisms. A biodynamic model was developed to assess the kinetics of NP bioaccumulation and depuration and to distinguish between reversible and storage particle reservoirs inside the organism. The accumulation kinetic parameters were compared with data for microplastic, re-interpreted from an earlier study. We demonstrate that using metal-doped plastics provides opportunities for precise quantification of NP accumulation and exposure in fate and effect studies, which can be a clear benefit for NP risk assessment.
|
Introduction
Plastic accumulation in the environment has been of great societal, political and scientific concern in the last decade due to its ubiquity and ability to persist for long time periods.1 Once plastics are released into the environment, they go through physical and biological degradation processes, resulting in the formation of both microplastics (MP; <5 mm) and nanoplastics (NP; <1 μm).1,2 Moreover, some pharmaceutical and cosmetic products contain NP and MP, which can also enter marine, freshwater and terrestrial ecosystems.3,4 Environmental concentrations of MP have been measured in water, sediment and biota samples in all habitats worldwide.5,6 NP abundance in environmental ecosystems is expected to be high due to the aforementioned fragmentation of larger plastics, which are found to degrade into NP after long-term exposures to visible and UV light.4,7–10 NP are predicted to be particularly abundant in freshwater sediments due to their retention caused by a fast hetero-aggregation with natural solids, thereby posing an exposure route to benthic biota.11,12 However, NP concentrations in environmental matrices are still generally unquantified, as the sampling methods and identification techniques available for particulate plastic generally have detection limits >1 μm.13 The difficulty to detect NP is one of the major challenges in assessing their proliferation and risk. Several methods have been proposed to detect them, even in complex natural matrices, such as the use of crossflow ultrafiltration coupled with field flow fractionation and pyrolysis gas chromatography-mass spectrometry.13,14 We are aware of only one study that has been able to detect NP of various polymer types in surface water samples taken at the North Atlantic subtropical gyre.14 Because of the difficulties in applying NP analytical methods to complex environmental matrices, no study has ever measured NP concentrations in field sediments and biota samples.15
These challenges for detecting NP in the environment limit the evaluation of their exposure, but also the assessment of their effects and the risks they pose to biota and to human health13,15,16 Although the effects of manufactured NP on aquatic biota have been broadly investigated for exposures in aqueous media, the mechanisms behind these effects are unclear due to the aforementioned analytical difficulties which hamper the determination of uptake by biota.17,18 To date, many studies have used fluorescently-labeled plastics to assess biological uptake of NP.19–21 Some of these studies observed specific tissues or the whole body of the organisms under a fluorescent microscope, while others measured the fluorescent particles in the remaining solution with a fluorescence spectrophotometer after digesting the samples.19–21 The suitability of fluorescently-labelled NP to assess ingestion was recently questioned, as Catarino et al., 2019 and Schür et al., 2019 demonstrated that the fluorescent dye can leach out of the NP and reach biological tissues without the plastic.22,23 In addition, cell auto-fluorescence is often not taken into account by studies assessing the ingestion of fluorescent plastic particles.22 Consequently, the use of fluorescently-labelled NP to assess biological uptake could lead to misinterpretation of the results.22 Doping with 14C is another valid method, which however may not be first choice for delicate effect studies even though the level of radiation is low.24 Furthermore, use of radioactive materials may in many cases require specialty labs, which could be logistically problematic. Another method has been proposed to track NP in complex matrices, which consists of the use of metal-doped plastics, which can be measured accurately with sensitive analytical techniques such as inductively coupled plasma mass spectrometry (ICP-MS).25 This approach has also been successfully applied to study the fate and behavior of NP in complex environmental systems26,27 as well as the impacts to the model bacteria Shewanella oneidensis.28
Aquatic organisms are able to actively ingest NP or adsorb them to their surfaces and transfer them into higher trophic levels.8,18 The effects of NP on aquatic biota have raised particular concerns over the last years due to their small size, which allows them to be taken up by cells, affecting biota on a cellular level.8,18 In fact, a recent review concluded that NP caused more adverse effects on aquatic organisms than MP.18 NP have been found to cause deleterious effects on aquatic biota at the individual level, affecting their growth, reproduction, mobility and feeding; at the suborganismal level, causing oxidative stress and affecting their gene expression and immune system among other effects; and at population level, affecting the community composition.29–35 Studies assessing the effects of NP generally use pristine and smooth spherical particles.18 However, irregularly shaped NP could occur more often in nature due to their formation through fragmentation and degradation of MP.15 Hence, testing irregularly shaped NP under more realistic environmental conditions should be a priority, as effects of NP might be shape-dependent.36 In contrast to the abundant literature data on NP effects on aquatic biota in aquatic exposures, the effects of NP in sediment exposure conditions have only been studied for a few organisms. Testing NP in sediment comes with different complexities than aqueous tests. Moreover, some studies published used particle mixtures as exposures, rendering it impossible to distinguish between NP and MP effects.29,37–39 To date, we are aware of two articles that have evaluated the effects of NP only on freshwater benthic species using sediment exposure conditions.29,40
In this study, we used metal-doped NP to assess the uptake and effects on the survival and growth of the freshwater benthic macroinvertebrate Gammarus pulex using 28 days standardized single species sediment toxicity tests. Individuals of G. pulex were exposed to 227.6 ± 1.47 nm polystyrene NP with a bumpy surface containing a palladium (Pd) tracer at concentrations ranging from 0 to 30 g plastic per kg sediment dry weight (dw). We measured the concentration of Pd in the body of the exposed organisms and in the faecal pellets excreted during a 24 h depuration period after the chronic exposure as a means to assess NP uptake and bioaccumulation. Concentrations of Pd in sediments were quantified at the start of the experiment and in water samples at the start and at the end of the experiment to show the extent and homogeneity of the NP dosing. Data interpretation was assisted by biodynamic modelling of the bioaccumulation of NP by G. pulex.
Materials and methods
Nanoplastics
A solution containing metal-doped NP with a bumpy surface were synthesized according to our previous work.41 Briefly, the NP consisted of a polyacrylonitrile core with Pd doping (0.3% by weight) and a polystyrene shell, resulting in a rough (bumpy) outer surface of the particles. The Pd tracer was chemically entrapped in the polymer core material (polyacrylonitrile), and then another polymer (polystyrene) was added on top to make sure there was no metal on the surface. TEM/EDX images showed the location of the metal, which confirmed there was no Pd on the surface.41 Furthermore, it was demonstrated that minimal leaching of the metal from the NP occurred over time in a variety of environmental and biological conditions.41 Also note that we did not detect effects in the present study, hence the undesired effect of leaching was in fact checked again and found to not play a role. Nevertheless, checking the tracer stability for further assays remains recommendable. The z-average size (nm) was measured by dynamic light scattering (DLS) with a Zetasizer (Nano ZS, Malvern instruments), the shape was confirmed by scanning electron microscopy (SEM) imaging and the solid content was measured with thermogravimetric analysis. Average particle size was 227.6 ± 1.47 nm (n = 3) as measured by DLS, SEM images confirmed the bumpy surface of the particles (Fig. S1†), and the dry content was 8.55%.
Styrene and acrylonitrile are volatile compounds that can be toxic to aquatic organisms when effect threshold concentrations are exceeded.42,43 To remove any potential remains of these chemicals from the particle synthesis process, the solution was purged with clean air for 10 h at 30 °C with an airflow of 5 L h−1. This purging duration, together with subsequent aeration for 2 weeks, was a priori designed to cause >99.99% removal based on air flow, the chemicals Henry's law constants and an assumed chemical equilibrium between these chemicals in solution and bubbles rising over the height of the water column.
Ruling out interference from background levels of chemicals
Despite nanoplastic cleaning methods, some traces of styrene and/or acrylonitrile may have remained in solution in addition to small amounts of other chemicals used during particle synthesis (e.g. surfactants SDS and KPE). These materials could potentially negatively affect the tested organisms, and thus confound the results as to if chemicals or nanoplastics were responsible for any observed effects. To address these potential effects from co-exposure to the chemicals involved in the NP synthesis, we calculated if the chemical concentrations in the experimental design were below known effect threshold concentrations (provided as ESI;† Table S2, Fig. S3 and S4).
Test organisms
We selected the amphipod Gammarus pulex as a test organism because of its key role in aquatic ecosystems and its demonstrated sensitivity to MP.44–46 Following previous procedures conducted in our labortories,46–48G. pulex were collected from a non-contaminated48 brook in Heelsum, The Netherlands, in June 2019. Once in the lab, individuals were sorted by their narrow body size, excluding the smallest and largest for use in the NP exposure tests. Organisms were acclimatized in aerated buckets with copper-free Dutch standard water (DSW) in a water bath at 15.5 ± 1 °C while maintaining a 12
:
12 light
:
dark cycle. During the acclimatization period (14 days), organisms were fed with field dry poplar leaves.
Sediments
Following previous studies conducted in our laboratory,33,46–48 sediments were sampled from an unpolluted48 ditch in Veenkampen (Wageningen, The Netherlands) with a standard dip and sieved over a 2 mm sieve. Sediments in the containers were allowed to settle overnight and the overlying water was removed the morning after. Remaining sediments were homogenized with a hand drill and stored in a freezer to kill any living organisms and to preserve the sediment. Directly before the start of the experiments, sediments were unfrozen and re-homogenized. Four representative sediment subsamples were taken to determine the total organic matter (TOM) content, using the loss on ignition method (3 h, 550 °C), which was 39.95 ± 0.92%.
Experimental design
A total of 11 individuals of G. pulex per experimental unit were exposed to NPs at concentrations of 0, 0.03, 0.1, 0.3, 1 and 3% of plastic in sediment dw, which corresponds to 0, 0.3, 1, 3, 10 and 30 g of plastic per kg of sediment dw. For each concentration, five replicas were prepared, from which three were used for the exposure assessment and two were used to verify the concentration (in mass) of NP in sediment and water at the start of the experiment (t = 0). For the controls, three additional replicas were made for the exposure assessment. Experimental units consisted of 750 ml glass beakers containing 184 g of wet sediment, spiked with the corresponding NP concentrations and a 350 ml copper-free DSW layer. To avoid NP homoaggregation and assure homogeneous mixing during the preparation of the amendments, the plastic solutions were added drop by drop to sediment contained in a 2 L glass beaker placed inside an ultrasonic waterbath. At the same time, the sediment was vigorously mixed with a stainless steel hand mixer.29,33 Sediment amendments were prepared per concentration and were then divided into replicate beakers, to assure replicates to be as identical as possible. After the addition of the sediment, beakers were allowed to settle for 24 hours and subsequently DSW water was slowly added to avoid resuspension of the particles into the water phase. Finally, beakers were randomly placed in a water bath and acclimatized at 15.5 ± 1 °C with a 12
:
12 light
:
dark cycles for two weeks prior to the start of the experiment. After the acclimatization, 11 organisms were randomly introduced into each of the beakers. In addition, another 66 randomly selected organisms were preserved in 70% ethanol to assess the length of the starting population. Organisms were fed with two 3 cm poplar leaf discs at days 0 and 14, which were previously soaked in DSW for 3 days. Aeration was supplied to the beakers and the top water layer was carefully renewed weekly in all beakers to keep the water levels constant. Temperature, dissolved oxygen, pH, electroconductivity, and ammonia levels (NH3) were measured once a week in one replicate per exposure concentration. The mean (±s.d) temperature, dissolved oxygen, pH, electroconductivity and ammonia levels (NH3) along the experiment were 16.0 ± 0.17 °C, 9.5 ± 0.32 mg L−1, 7.8 ± 0.12, 535 ± 33.7 μS cm−1 and 0.87 ± 0.98 mg L−1, respectively.
Effects on survival and growth
At the end of the experiment, beakers were sieved over a 0.35 mm sieve and gently washed with tap water. Surviving individuals were counted, rinsed and transferred to glass beakers containing 30 ml of clean DSW, where they were allowed to depurate their gut content for 24 hours. Thereafter, G. pulex were placed in 70% ethanol until their length and that of the starting population was measured under an Olympus SZX10 stereomicroscope. For this, the head capsule (HD) size was measured and the total length (TL) was calculated following the equation: TL = −2.07 + 9.82 HD.49 The growth was then calculated as the difference in the mean TL of the exposed organisms per replicate minus the mean TL of the starting population. The average size of the starting population was 5.37 ± 0.91 mm (n = 66).
Palladium analysis of the metal-doped nanoplastics in biota, faeces, sediment and water samples
After measuring the length of the exposed G. pulex, organisms were dried in an oven at 40 °C for 24 h and weighted per experimental unit. Nanoplastic body burden was assessed by total Pd concentration as a group per replica, as mg Pd/mg dw G. pulex. Glass beakers containing 30 ml DSW with the faecal pellets depurated after the 28 days exposure for 24 h were freeze dried prior to the analysis of the Pd concentrations as μg Pd/ml DSW.
For the analysis of Pd in sediments, at t = 0 beakers were taken out of the waterbath and the overlying water was carefully removed with a syringe. All sediments in their original beakers and 60 ml of the removed water from concentrations 0, 1 and 3% NP per sediment dw placed in glass beakers were stored in the freezer until further analysis. In addition, 60 ml of water was taken from the three replicate beakers at concentrations 0 and 3% at t = 28 before sieving them, to collect the surviving individuals in order to analyse whether Pd concentrations in water (as μg Pd/ml DSW) increased over the exposure to G. pulex. Sediment samples of all concentrations were freeze dried and homogenized with a stainless-steel spoon prior to analysis. A total of 300 mg of dry sediment were weighed per replicate and Pd concentrations were measured as μg Pd/mg dw of sediment.
All samples underwent microwave acid digestion prior to analysis by ICP-MS. Biota and sediment samples were placed into Teflon digestion vessels with 6 mL of concentrated HNO3 and 2 ml HCl. For the faeces and water samples, glass beakers were washed with the HNO3 and HCl, mixed with a pipette and added to the Teflon tubes. Immediately after, tubes were closed and left overnight at room temperature (20 ± 1 °C). The morning after, tubes were introduced into a microwave (CEM MARS 6) to allow the first digestion step to take place (200 °C for 60 min). Once the first digestion step was completed, the sample was taken out and allowed to cool to room temperature. Then, two 0.75 mL aliquots of H2O2 and 3.25 ml ultrapure water were added to the sample with a second round of microwave digestion (175 °C for 15 min). Samples were then transferred into a 50 mL DigiPrep tube. The volume of the sample was made up to 50 mL by adding ultrapure water. Pd concentration in the samples was measured using High Resolution ICP-MS (Thermo Scientific, Element2). Control samples for every digestion matrix were performed, with two replicate samples of the NP stock solution and two replicate samples of a dissolved Pd standard (200 μg l−1) spiked into the matrix. The Pd variation between replicates in the three runs was 2.5% for NP stock solution and 1.5% for the dissolved Pd stock standards. Recovery tests were performed using two Pd spiked sediment samples and one Pd spiked G. pulex sample. Recovery of Pd from the spiked samples were 100.3 ± 0.6% and 102% from the sediment and G. pulex, respectively. Detection limit values for the different sample types are provided in Table S1.†
Statistical analysis
Analysis of the data was done in SPSS 23 (IBM Corp., NY). Generalized linear models (GLMs) were used to study the effects of the NP on the survival of the tested organisms. One-way ANOVA (p < 0.05) were used to study the effects of the NP on the growth of the tested organisms. The normality of the residuals was first checked with the Shapiro–Wilk test (p > 0.05) and visualized on a Q–Q plot. Homogeneity of the variances was tested using Levene's test (p > 0.05) and post hoc multiple comparisons were done using Tukey's and Bonferroni tests. Linear regressions were fit for the Pd concentrations measured in the plastic–sediment mixtures at t = 0 as a function of the NP concentrations in sediment. Linear regressions were fit for the NP concentrations based on the measured Pd in bodies and faeces of G. pulex at the end of the experiment and after 24 h gut depuration period as a function of the NP concentrations in the sediment. All data are depicted with average ± standard deviation, unless otherwise stated.
Biodynamic modelling of the bioaccumulation of Pd-doped nanoplastics by G. pulex
The bioaccumulation of NP by G. pulex between t = 0 and t = 28 d was modelled as a function of NP dose and time (eqn (1)) using a traditional first order two compartment model allowing for irreversible uptake in the body and reversible transport to and from the gut, under constant exposure conditions:50,51 |  | (1) |
For the subsequent 1 d depuration phase, i.e. t > 28 d, eqn (2) was used: | CG. pulex,t−28 = CG. pulex,t=28 × e−kelim×(t−28) | (2) |
Here, CG. pulex is the measured NP concentration in G. pulex (μg kg−1), CSED is the measured exposure NP concentration in sediment (μg kg−1), kup is the uptake rate constant (μg × kg−1 biota/μg × kg−1 sediment × day−1), kelim is the elimination rate constant (day−1), FB is the poorly or irreversible fraction accumulating in the body (dimensionless) and t is exposure time (days). Note that the compartments are kinetically defined and that referring to the reversible and irreversible particle reservoirs as ‘gut’ versus ‘body’ formally is a matter of interpretation. The model was fitted to the experimental data by optimizing the parameters kup, kelim and FB using a weighted relative least squares criterion.
Results and discussion
NP in sediment and water samples
The concentration of Pd in the NP stock solution was 3.02 ± 0.08 (n = 6) g kg−1, which means that NP contained 0.302 wt% Pd. The average measured background Pd concentration in sediment was 2.620 mg kg−1 (n = 2), which is close to the value of the intercept (2.259 ± 0.1365) (Fig. 1). At t = 0, measured Pd concentrations in sediment were proportional to the nominal NP doses (R2 = 1, n = 12), with a slope corresponding to a Pd content of 3.009 ± 0.0105 g kg−1 (Fig. 1). These values of 3.022 and 3.009 are identical within error limits, confirming the adequate and representative addition, mixing and analysis of the Pd-doped NP in the sediment matrix (Fig. 1) (linear regression (LR), p-value = 2.2 × 10−16).
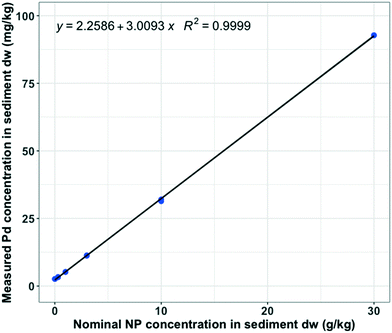 |
| Fig. 1 Measured Pd concentrations in sediment dw (mg kg−1) at t = 0 as a function of the nominal NP concentrations in sediment dw (g kg−1). The linear regression is based on 12 individual data points. | |
Following the same conversion between Pd and NP, average measured NP concentrations in water at t = 0 were 0.251 ± 0.056 mg L−1 and 1.390 ± 0.037 (n = 2) mg L−1 for the nominal NP spiking concentrations of 10 and 30 g kg−1 in sediment dw, respectively. Knowing the concentration of NP in the overlying water, we calculated the proportion of NP which were resuspended from the sediment at t = 0, which was 0.07%. We hypothesize that this resuspension was a result of either the system preparation procedure or the aeration of the tanks during the acclimatization. At t = 28 d, the measured NP concentration in the overlying water was 19.83 ± 3.94 (n = 3) mg L−1 for the nominal NP concentration of 30 g kg−1 in sediment dw. At t = 28 d, 1% of the NP were found to be in suspension, which suggests that the exposures of NP in the sediment remained close to their nominal spiking values throughout the experimental time period.
Ingestion of NP by G. pulex
Pd concentrations were measured in the body of the surviving organisms after the 28 d exposure to the NP and a posterior 24 h defaecation period in DSW. Additionally, Pd concentrations in the excreted faeces were measured. There was a linear relationship (LR, p-value = 3.46 × 10−3) between the concentration of NP measured in the body of G. pulex (mg g−1) and the nominal NP concentration in sediment dw (g kg−1) (Fig. 2A). A significant positive linear relationship (LR, p-value = 1.49 × 10−7) was found between the measured NP concentrations in faeces of G. pulex and the nominal NP concentrations in sediment (Fig. 2B). Because NP were measured in the body and the egested faeces, the total ingested NP can be calculated as the sum of these components. For this total ingested NP, a highly significant positive linear relationship with dose was obtained (LR, p-value = 4.37 × 10−9) with a multiple R-squared of 0.89 (Fig. 2C). As the datapoints have incremental intervals, we also provide the log-transformed version of Fig. 2 (ESI,† Fig. S2).
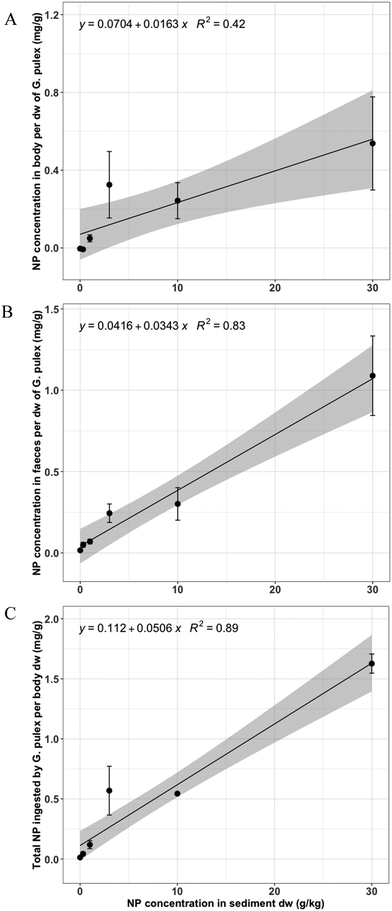 |
| Fig. 2 NP concentration measured in A) the body of G. pulex (mg g−1), B) faeces of G. pulex per body weight (mg g−1) and C) total NP ingested by G. pulex (mg g−1) per body dw after summing up the concentration of NP in bodies and faeces; after 28 d exposure to NP concentrations in sediment dw (g kg−1). Error bars relate to SE (n = 3). Linear regressions are based on 18 individual data points with grey areas indicating the 95% confidence interval. | |
A summary of the NP concentrations measured in body and faeces of G. pulex per body dw, alone and combined as the total NP ingested, can be found in Table S2.† Interestingly, the relative errors (n = 3) were substantially smaller for the total NP ingested in the highest two spike concentrations (10 and 30 g kg−1 sediment dw) compared to the errors in the separate body and faeces concentrations. This indicates that defaecation as well as body burden had higher variation than the total ingestion. The average proportion of NP defaecated by G. pulex was 58.6 ± 23.2 (n = 12), including the four highest concentrations (1–30 g kg−1). The lowest exposure concentration (0.3 g kg−1) was excluded from the dataset as measurement values were below the ICP-MS detection limit (see Table S1†).
Biodynamic modelling of the bioaccumulation of NP by G. pulex
Mass balance calculations of NP in the sediment, water and biota showed that after 28 d of exposure, less than 1% of NP mass was lost from the sediment. This demonstrates that the model assumption of a constant exposure concentration was met. We fit the first order bioaccumulation model (eqn (1) and (2)) with three parameters (p) to four triplicate concentrations at two time points i.e. (p = 3, n = 24). The two lowest doses (0 and 0.3 g kg−1) were omitted as their Pd concentrations were below the ICP-MS detection limit (see Table S2†). The fit to the experimental data was always within 1 s.d. (Fig. 3), which confirmed the absence of dose dependency and was highly significant (ANOVA, p-value = 1.7 × 10−77). Model parameter optimization yielded an uptake rate constant (kup) of 0.076 μg × kg−1 biota/μg × kg−1 sediment × day−1 and an elimination rate constant (kelim) of 2.44 day−1. Steady state concentrations in the reversible (gut) compartment were reached within 2 to 3 days (Fig. 3). Subsequently, uptake in the irreversible (body) compartment steadily increased, suggesting that accumulation would have continued beyond the time frame of our experimental set-up. After 28 d, depuration rapidly removed part of the total accumulated NP from the organism, but a poorly reversible fraction remained (Fig. 3 insert), which illustrates the necessity of distinguishing between these two compartments. A fraction of only 0.96% (FB = 0.0096) of NPs ingested were estimated to transfer from the gut into the irreversible (body) compartment. However, even this small fraction eventually leads to a considerable body burden after 28 d due to the lack of (measurable) depuration from that reservoir.
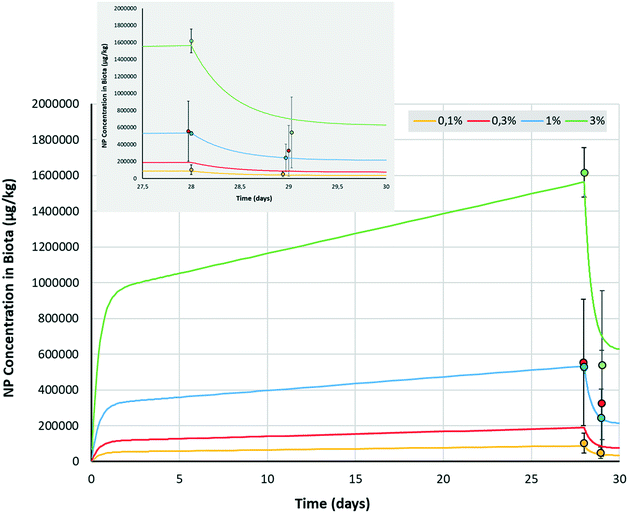 |
| Fig. 3 Measured (datapoints) and modelled (curves) uptake of Pd-doped NPs by G. pulex over 28 d of exposure to sediment amended with Pd-doped NPs, followed by 1 d of depuration in clean medium (insert). Data on measured NP concentrations (±1 SD) after depuration (see insert) after 29 days were set apart for 0.05 day for better visibility of the datapoints on the x-axis. | |
Redondo-Hasselerharm et al. (2018) defined a MP steady state trophic transfer factor (TTF) for the body of G. pulex as TTFBODY = CG. pulex,body/CSED.46 This TTF relates to the mass concentration of MP remaining in the organisms after gut depuration, divided by the MP mass concentration in the sediment after 28 d. The value for the TTFBODY for NP in the present study was 0.020 μg × kg−1 biota/μg × kg−1 sediment dw (Table 1). Similar to TTFBODY, an apparent TTF for the reversible (gut) compartment can be calculated as TTFGUT = CG. pulex,faeces/CSED = kup/kelim, which was 0.031 μg × kg−1 biota/μg × kg−1 sediment dw. Consequently, the comparison between the modelled TTFGUT and TTFBODY reveals that after 28 d, 60.2% (100 × 0.031/[0.031 + 0.020]) of all ingested NP reside in the gut, compared to 39.8% in the body (Table 1). These percentages derived from the model are consistent with the 58.62 ± 23.15% observed to be defaecated from the gut in the experimental work.
Table 1 Bioaccumulation kinetic parameters and sizes of apparent accumulation reservoirs for nanoplastic (present study) and microplastic (remodelled from Redondo-Hasselerharm et al. 2018)46 in Gammarus pulex
Parametera |
Nanoplastic |
Microplastic |
Unit |
Ranges relate to 95% confidence intervals.
These TTF values and percentages are conditional; they depend on exposure time.
|
Uptake rate constant (kup) |
0.076 (0.073–0.078) |
0.044 (0.038–0.049) |
μg × kg−1 biota/μg × kg−1 sediment × day−1 |
Elimination rate constant (kelim) |
2.44 (2.30–2.59) |
4.61 (2.59–22.1) |
Day−1 |
Irreversible (stored) fraction (FB) |
0.96 (0.87–1.04) |
2.79 (2.37–3.20) |
% |
TTFBODYb |
0.020 |
0.092 |
[mg kg−1 organism]/[mg kg−1 sediment] |
TTFGUT |
0.031 |
0.025 |
[mg kg−1 organism]/[mg kg−1 sediment] |
TTFTOTALb |
0.051 |
0.116 |
[mg kg−1 organism]/[mg kg−1 sediment] |
Percentage in bodyb |
39.8 |
78.7 |
% |
Percentage in gutb |
60.2 |
21.3 |
% |
Comparison of accumulation kinetics and trophic transfer factors between NP and MP
We have previously derived bioaccumulation data for MP using the same sediment, test organism and experimental design as used in this present experiment. This calls for a comparison between the two datasets, which we here provide by applying the same modelling approach to the MP data (details provided as ESI;† Fig. S5). The kinetic parameters, 28 d TTFGUT and 28 d TTFTOTAL agree with parameters estimated for NP within a factor of two (Table 1). This is striking considering the large size difference between the two test particles (0.23 μm NP versus 20–165 μm MP). This suggests a random ingestion of particles dominated by species traits rather than particle properties. It has been hypothesized that satiation in combination with dilution of food is one of the main demonstrated adverse effect mechanisms upon ingestion of small plastic particles.36 This mechanism would imply that the mass-based TTFGUT values would be similar regardless of actual particle size, as long as particles would be ingestible.36,52 The current finding that NP and MP with large size differences yield very similar TTFGUT under the same exposure conditions supports this hypothesis. We emphasize that this value only applies to the 28 d time point. TTFBODY is likely to increase further after 28 d, but the current data do not allow us to speculate on the actual trend. With a value of 0.092, the TTFBODY, reflecting accumulation in the irreversible fraction, was 4.5 times larger for MP compared to NP. This can be explained from MP particles being trapped in the gut, as opposed to NP particles that would easily be defaecated with other organic matter-based gut contents.
Effects on survival and growth
The average survival in controls was 86.4% ± 9.5 (n = 6). The exposure to NP had no significant effects on the survival of G. pulex (GLM, p-value = 0.577). No significant differences on the growth of G. pulex were found among the NP concentrations ranging up to 30 g kg−1 sediment dw (Fig. 4) (ANOVA, p = 0.057).
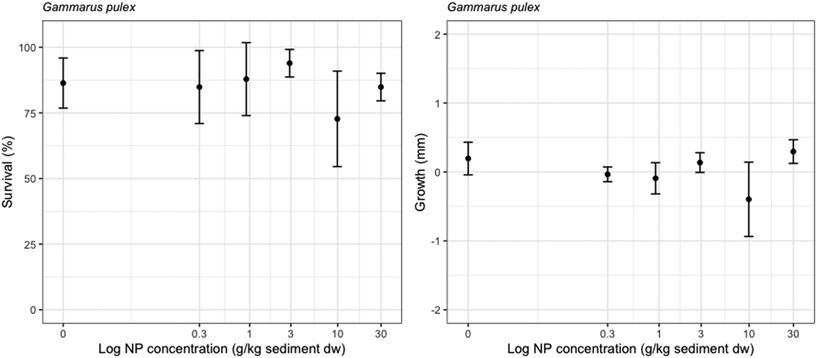 |
| Fig. 4 Survival (%) and growth as length (mm) of Gammarus pulex as a function of the NP concentration (g kg−1 sediment dw) on a log scale. Error bars are mean ± SD (n = 3), except for controls, where n = 6. | |
Little is known about effects of NPs on benthic invertebrates.2 Heinlaan et al. 2020 assessed the effects of 26 and 100 nm PS NP on the midge larvae Chironomus riparius and the ostracod Heterocypris incongruens.40 No significant effects were found on the survival of C. riparius after 48 h of exposure and no significant effects were found on the survival and growth of H. incongruens after 6 d of exposure.40 In Redondo-Hasselerharm et al. (2020), the effects of 96 nm PS NP were evaluated on a freshwater benthic community using outdoor tests.29 While after 3 months of exposure, no effects were found on the community composition, a reduction in the abundance of Naididae worms was observed after 15 months of exposure at a concentration of 5% plastic per sediment dw.29 The absence of shorter term effects (up to 3 months) of NP on freshwater benthic organisms found in previous papers is therefore in accordance with the results obtained in this study.
Previous studies have stated that NP might pose a greater risk compared to MP due to their smaller size, as they are more prone to getting lodged in small body structures.19,53 However, Redondo-Hasselerharm et al. (2018) found significant adverse effects on the growth of G. pulex after a 28 d sediment exposure to irregularly shaped PS MP (size range: 20–500 μm), with a median effect dose (EC50) of 3.57 ± 3.22%.46 The TTFGUT mass value found for the PS MP was 0.025, which is very close to the TTF mass value found in the present study. However, the difference in effects found for both particle types may be related with the higher TTFBODY found for the PS MP (4.5× higher, see Table 1), which may reflect particles being trapped in the gut, leading to hindered passage of food or other functions, leading to extra stress.
Conclusion
We used Pd-doped PS NP with a bumpy surface to assess uptake and effects on the freshwater benthic amphipod G. pulex using single species tests with natural sediments. Bioaccumulation was demonstrated, but no effects of the NP were found on the survival and growth of G. pulex at concentrations up to 30 g kg−1 of sediment dw. Therefore, the no observed effect concentration (NOEC) was equal to 30 g kg−1 of NP per sediment dw. The model particles were used as a proxy for environmental NP, and we demonstrated that metal-doping enabled us to accurately measure the NPs in various highly complex environmental matrices such as natural water, sediment and biota. This was very advantageous, since currently direct NP analysis would not have been possible and thus we would have only been able to report effects on the organisms. Instead, we were able to use the Pd-doped particles to conduct a mass balance between all environmental and biological compartments, as well as to quantify NP uptake and depuration rates. This approach provides opportunities for more refined exposure and effect assessments, which eventually leads to high quality assessments of the risk of NP in the environment.
Author contributions
PERH, AAK and DMM conceptualized the study. PERH, AAK, GV and DMM designed the methodology. DMM synthesized the metal-doped nanoplastics, PERH conducted the experiments and GV quantified the metal-doped nanoplastics in the different matrices. PERH and AAK carried out the data analysis and AAK did the biodynamic modeling. PERH drafted the manuscript and AAK, DMM and GV reviewed and edited it. AAK supervised the work and acquired funding.
Conflicts of interest
There are no conflicts of interest to declare.
Acknowledgements
We thank Wobbe Schuurmans and Peter Nobels from the Chemical Biological Soil Laboratory (CBLB) of Wageningen University and Research for contributing to the analysis of the metal-doped NP. We also thank B. F. Arlina, J. Beijer and F. Gillissen for their practical assistance. Edwin Peeters is acknowledged for statistical advice. This study was funded by the Dutch Technology Foundation TTW, project no. 13940. We acknowledge additional support from KWR; WMR; NVWA; RIKILT; the Dutch Ministry of Infrastructure and the Environment; the Dutch Ministry of Health, Welfare and Sport; Wageningen Food and Biobased Research; STOWA; RIWA; and water boards Hoogheemraadschap van Delfland, Zuiderzeeland, Rijn en IJssel, Vechtstromen, Scheldestromen, Aa en Maas, de Dommel, and Rivierenland. D. M. M. was supported by the Swiss National Science Foundation, Ambizione Grant number PZP002_168105.
References
- D. K. A. Barnes, F. Galgani, R. C. Thompson and M. Barlaz, Philos. Trans. R. Soc., B, 2009, 364, 1985–1998 CrossRef CAS PubMed.
-
Science Advice for Policy by European Academies, A Scientific Perspective on Microplastics in Nature and Society, Berlin, 2019 Search PubMed.
-
J. Boucher and D. Friot, Primary microplastics in the oceans: A global evaluation of sources, IUCN, Gland, Switzerland, 2017 Search PubMed.
- K. Mattsson, L. A. Hansson and T. Cedervall, Environ. Sci.: Processes Impacts, 2015, 17, 1712–1721 RSC.
-
T. Schell, A. Rico and M. Vighi, in Reviews of Environmental Contamination and Toxicology, Springer Nature, Switzerland AG, 2020 Search PubMed.
- A. A. Koelmans, N. H. Mohamed Nor, E. Hermsen, M. Kooi, S. M. Mintenig and J. De France, Water Res., 2019, 155, 410–422 CrossRef CAS PubMed.
- A. L. Andrady, Mar. Pollut. Bull., 2011, 62, 1596–1605 CrossRef CAS PubMed.
- R. Lehner, C. Weder, A. Petri-Fink and B. Rothen-Rutishauser, Environ. Sci. Technol., 2019, 53, 1748–1765 CrossRef CAS PubMed.
- S. Lambert and M. Wagner, Chemosphere, 2016, 145, 265–268 CrossRef CAS PubMed.
- S. Lambert and M. Wagner, Chemosphere, 2016, 161, 510–517 CrossRef CAS PubMed.
- E. Besseling, J. T. K. Quik, M. Sun and A. A. Koelmans, Environ. Pollut., 2017, 220, 540–548 CrossRef CAS PubMed.
- M. Wagner, C. Scherer, D. Alvarez-Muñoz, N. Brennholt, X. Bourrain, S. Buchinger, E. Fries, C. Grosbois, J. Klasmeier, T. Marti, S. Rodriguez-Mozaz, R. Urbatzka, A. D. Vethaak, M. Winther-Nielsen and G. Reifferscheid, Environ. Sci. Eur., 2014, 26, 12 CrossRef PubMed.
- S. M. Mintenig, P. S. Bäuerlein, A. A. Koelmans, S. C. Dekker and A. P. Van Wezel, Environ. Sci.: Nano, 2018, 5, 1640–1649 RSC.
- A. Ter Halle, L. Jeanneau, M. Martignac, E. Jardé, B. Pedrono, L. Brach and J. Gigault, Environ. Sci. Technol., 2017, 51, 13689–13697 CrossRef CAS PubMed.
- A. A. Koelmans, Nat. Nanotechnol., 2019, 14, 307–308 CrossRef CAS PubMed.
- E. Hermsen, S. M. Mintenig, E. Besseling and A. A. Koelmans, Environ. Sci. Technol., 2018, 52, 10230–10240 CrossRef CAS PubMed.
- L. C. de Sá, M. Oliveira, F. Ribeiro, T. Lopes Rocha, M. N. Futter, D. Barcelo, L. C. de Sá, M. Oliveira, F. Ribeiro, T. L. Rocha and M. N. Futter, Sci. Total Environ., 2018, 645, 1029–1039 CrossRef PubMed.
- T. Kögel, Ø. Bjorøy, B. Toto, A. M. Bienfait and M. Sanden, Sci. Total Environ., 2020, 709, 136050 CrossRef PubMed.
- S. Rist, A. Baun and N. B. Hartmann, Environ. Pollut., 2017, 228, 398–407 CrossRef CAS PubMed.
- M. Cole and T. S. Galloway, Environ. Sci. Technol., 2015, 49, 14625–14632 CrossRef CAS PubMed.
- R. Cui, S. W. Kim and Y. J. An, Sci. Rep., 2017, 7, 12095 CrossRef PubMed.
- A. I. Catarino, A. Frutos and T. B. Henry, Sci. Total Environ., 2019, 670, 915–920 CrossRef CAS PubMed.
- C. Schür, S. Rist, A. Baun, P. Mayer, N. B. Hartmann and M. Wagner, Environ. Toxicol. Chem., 2019, 38, 1495–1503 CrossRef PubMed.
- M. Al-Sid-Cheikh, S. J. Rowland, R. Kaegi, T. B. Henry, M.-A. Cormier and R. C. Thompson, Commun. Mater., 2020, 1, 1–8 CrossRef.
- D. S. Vicentini, D. J. Nogueira, S. P. Melegari, M. Arl, J. S. Köerich, L. Cruz, N. M. Justino, B. V. Oscar, R. C. Puerari, M. L. N. da Silva, C. Simioni, L. C. Ouriques, M. S. Matias, A. B. de Castilhos Junior and W. G. Matias, Environ. Toxicol. Chem., 2019, 38, 2101–2110 CrossRef CAS PubMed.
- S. Frehland, R. Kaegi, R. Hufenus and D. M. Mitrano, Water Res., 2020, 182, 115860 CrossRef CAS PubMed.
- A. S. Keller, J. Jimenez-Martínez and D. M. Mitrano, Environ. Sci. Technol., 2020, 54, 911–920 CrossRef CAS PubMed.
- V. S. Fringer, L. P. Fawcett, D. M. Mitrano and M. A. Maurer-Jones, Front. Environ. Sci. Eng., 2020, 8, 1–11 CrossRef.
- P. E. Redondo-Hasselerharm, G. Gort, E. T. H. M. Peeters and A. A. Koelmans, Sci. Adv., 2020, 6, eaay4054 CrossRef CAS PubMed.
- M. González-Pleiter, M. Tamayo-Belda, G. Pulido-Reyes, G. Amariei, F. Leganés, R. Rosal and F. Fernández-Piñas, Environ. Sci.: Nano, 2019, 6, 1382–1392 RSC.
- A. Wegner, E. Besseling, E. M. Foekema, P. Kamermans and A. A. Koelmans, Environ. Toxicol. Chem., 2012, 31, 2490–2497 CrossRef CAS PubMed.
- E. Besseling, B. Wang, M. Lürling and A. A. Koelmans, Environ. Sci. Technol., 2014, 48, 12336–12343 CrossRef CAS PubMed.
- S. van Weert, P. E. Redondo-Hasselerharm, N. J. Diepens and A. A. Koelmans, Sci. Total Environ., 2019, 654, 1040–1047 CrossRef CAS PubMed.
- A.-C. Greven, T. Merk, F. Karagöz, K. Mohr, M. Klapper, B. Jovanović and D. Palić, Environ. Toxicol. Chem., 2016, 35, 3093–3100 CrossRef CAS PubMed.
- Z. Liu, M. Cai, P. Yu, M. Chen, D. Wu, M. Zhang and Y. Zhao, Aquat. Toxicol., 2018, 204, 1–8 CrossRef CAS PubMed.
- V. N. de Ruijter, P. E. Redondo-Hasselerharm, T. Gouin and A. A. Koelmans, Environ. Sci. Technol., 2020, 54, 11692–11705 CrossRef CAS PubMed.
- D. S. Green, B. Boots, J. Sigwart, S. Jiang and C. Rocha, Environ. Pollut., 2016, 208, 426–434 CrossRef CAS PubMed.
- D. S. Green, B. Boots, N. E. O'Connor and R. Thompson, Environ. Sci. Technol., 2017, 51, 68–77 CrossRef CAS PubMed.
- M. Revel, N. Yakovenko, T. Caley, C. Guillet, A. Châtel and C. Mouneyrac, Environ. Sci. Pollut. Res., 2018, 27, 3574–3583 CrossRef PubMed.
- M. Heinlaan, K. Kasemets, V. Aruoja, I. Blinova, O. Bondarenko, A. Lukjanova, A. Khosrovyan, I. Kurvet, M. Pullerits, M. Sihtmäe, G. Vasiliev, H. Vija and A. Kahru, Sci. Total Environ., 2020, 707, 136073 CrossRef CAS PubMed.
- D. M. Mitrano, A. Beltzung, S. Frehland, M. Schmiedgruber, A. Cingolani and F. Schmidt, Nat. Nanotechnol., 2019, 14, 362–368 CrossRef CAS PubMed.
-
ECHA, Styrene - Registration Dossier - ECHA, https://echa.europa.eu/registration-dossier/-/registered-dossier/15565/6/2/5 Search PubMed.
-
ECHA, Acrylonitrile - Registration dossier - ECHA, https://echa.europa.eu/es/registration-dossier/-/registered-dossier/15561 Search PubMed.
- D. W. Kelly, J. T. A. Dick and W. I. Montgomery, Hydrobiologia, 2002, 485, 199–203 CrossRef.
- C. Macneil, J. T. A. Dick and R. W. Elwood, Biol. Rev., 1997, 72, 349–364 CrossRef.
- P. E. Redondo-Hasselerharm, D. Falahudin, E. T. H. M. Peeters and A. A. Koelmans, Environ. Sci. Technol., 2018, 52, 2278–2286 CrossRef CAS PubMed.
- P. E. Redondo-Hasselerharm, V. N. de Ruijter, S. M. Mintenig, A. Verschoor and A. A. Koelmans, Environ. Sci. Technol., 2018, 52, 13986–13994 CrossRef CAS PubMed.
- D. Kupryianchyk, E. P. Reichman, M. I. Rakowska, E. T. H. M. Peeters, J. T. C. Grotenhuis and A. A. Koelmans, Environ. Sci. Technol., 2011, 45, 8567–8574 CrossRef CAS PubMed.
- F. M. Wilhelm and D. C. Lasenby, Crustaceana, 1998, 71, 399–410 Search PubMed.
- N. W. Van Den Brink, A. Jemec Kokalj, P. V. Silva, E. Lahive, K. Norrfors, M. Baccaro, Z. Khodaparast, S. Loureiro, D. Drobne, G. Cornelis, S. Lofts, R. D. Handy, C. Svendsen, D. Spurgeon and C. A. M. Van Gestel, Environ. Sci.: Nano, 2019, 6, 1985–2001 RSC.
- F. Ribeiro, C. A. M. Van Gestel, M. D. Pavlaki, S. Azevedo, A. M. V. M. Soares and S. Loureiro, Sci. Total Environ., 2017, 574, 1633–1639 CrossRef CAS PubMed.
- A. A. Koelmans, P. E. Redondo-Hasselerharm, N. H. Mohamed Nor and M. Kooi, Environ. Sci. Technol., 2020, 54, 12307–12315 CrossRef CAS PubMed.
- M. Cole, P. Lindeque, E. Fileman, C. Halsband, R. Goodhead, J. Moger and T. S. Galloway, Environ. Sci. Technol., 2013, 47, 6646–6655 CrossRef CAS PubMed.
Footnote |
† Electronic supplementary information (ESI) available. See DOI: 10.1039/d1en00068c |
|
This journal is © The Royal Society of Chemistry 2021 |
Click here to see how this site uses Cookies. View our privacy policy here.