Interactive transgenerational effects of polystyrene nanoplastics and ethylhexyl salicylate on zebrafish†
Received
14th September 2020
, Accepted 4th December 2020
First published on 11th December 2020
Abstract
In this study, the transgenerational effects of polystyrene nanoplastics (PS-NPs) and ethylhexyl salicylate (EHS), and their interactive effects on zebrafish (Danio rerio) were investigated. It was found that EHS concentrations in F0 and F1 in co-exposure groups were higher than those in single exposure. The hatching rates of F0 and F1 decreased and the malformation and mortality rates of F1 increased, especially in co-exposure groups. For F0, antioxidant indicators (including glutathione, reactive oxygen species and malondialdehyde contents, and superoxide dismutase and catalase activities) and thyroid indicators (e.g. triiodothyronine contents and thyroid receptor A, thyroid receptor B and deiodinase 2 expression levels) in all test groups containing EHS were all increased, while the thyroxin content and deiodinase 1 expression levels were reduced compared with the corresponding control group. F1 showed similar or even stronger biochemical effects, while the indicators mentioned above for F0 and F1 in co-exposure groups were further intensified on the basis of EHS alone. Our results showed that the presence of PS-NPs may promote the bioaccumulation of EHS and its transfer from parents to offspring. EHS could induce growth and developmental toxicity, oxidative stress and thyroid toxicity on both parents and offspring, while PS-NPs and EHS exerted interactive effects on the transgenerational effects of EHS on zebrafish. Ultimately, two associated potential adverse outcome pathways of transgenerational toxicity were identified in EHS and PS-NP co-exposure: 1) destruction of thyroid hormone homeostasis; 2) generation of reactive oxygen species and malondialdehyde.
Environmental significance
More than 300 million tons of plastics are reported to be produced worldwide each year. Nanoplastics (NPs) can be used as carriers of hydrophobic organic pollutants and studies on the effects of NPs on the ecotoxicity of pollutants have been carried out gradually, but it is rarely reported whether they also affect the transgenerational effect of pollutants. Taken together, the effects of NPs on the ecotoxicity of ethylhexyl salicylate, one of the major organic UV filters, and associated potential adverse outcome pathways were investigated in this study in terms of bioaccumulation, endocrine disruption and DNA damage in parents or offspring. The findings provide a basis for the prevention and control of organic UV filters and NP pollution.
|
1. Introduction
Organic ultraviolet filters (OUVFs) are emerging contaminants1,2 and they have been detected in marine waters, sediments and organisms,3,4 among which ethylhexyl salicylate (EHS) is one of the major OUVFs. The detection rate of EHS in the surface water and sediments of the Yangtze River in China was up to 100%, and the highest concentrations of EHS were up to 6.31 ng L−1 and 9.60 μg kg−1 dry weight (dw), respectively.5 Cunha et al. monitored OUVFs in several environmental media and canned food organisms and results showed that the concentrations ranged from 10 to 400 μg kg−1 dw. Among them, the maximum concentration of EHS was 72.1 μg kg−1 dw.6 EHS is also one of the major pollutants in the Bohai Sea,7 the coastal areas of Portugal8 and the Mediterranean and Atlantic coasts of Spain.9
Numerous studies have shown that OUVFs can be transmitted to the offspring, resulting in transgenerational toxic effects.10–12 OUVFs have been detected in human fluids and tissues, such as breast milk, semen13 and placenta,14 which may lead to premature exposure of developing fetuses to OUVFs. Developing fetuses are particularly susceptible to the effects of endocrine disruptors that interfere with natural hormone systems.15 For example, exposing pregnant women to gonadal-disrupting OUVFs can induce hormonal changes in the womb, which can lead to developmental failure.16,17 Our previous study found that 2-ethylhexyl 4-methoxycinnamate (EHMC), one of the widely used filters, could be transferred to the offspring through parental exposure in zebrafish (Danio rerio), and the activity of antioxidant enzymes and the content of malondialdehyde (MDA) in both parents and offspring were all significantly increased, which caused oxidative damage and the response of offspring was more sensitive than that of parents.18 3-Benzylidene camphor and benzophenone-2 (BP-2) were found to induce the feminization of the secondary sexual characteristics of male fish, thereby reducing its fertility and reproductive rate.19,20
Microplastics (MPs) are emerging global pollutants with concentrations up to 48
146 items per m3, among which polystyrene is the most in demand polymers and has been widely detected in the aquatic environment.21–23 The sizes less than 100 nm are also defined as nanoplastics (NPs).24,25 Because of their high specific surface area and hydrophobicity, MPs can adsorb and carry organic pollutants into living organisms and produce carrier effects.26,27 There is evidence that the carrier effect of MPs can impact the migration ability, accumulation level and biotoxicity of pollutants in living organisms.28–30 For example, Koelmans et al. demonstrated that coexistent polyvinyl chloride NPs increased the accumulation of bisphenol A (BPA) in the head and viscera of adult zebrafish by a factor of 2.2 and 2.6, respectively, compared with BPA exposure alone.31 Zhang et al. found that the presence of MPs may promote the bioaccumulation of roxithromycin and can also induce the formation of the fish antioxidant enzyme system, thereby reducing roxithromycin-induced oxidative damage.32
When MPs adsorb pollutants and form composite pollutants, the composite pollutants would cross various biological barriers of organisms to various tissues such as gonads due to their small size, thus accelerate the transfer of pollutants from parents to offspring, resulting in increased transgenerational toxicity.33 However, there is little information on the interactive transgenerational effects of MPs and pollutants. Besides, compared with MPs, NPs possess a smaller particle size which enables them to penetrate more specific biological barriers and may lead to more severe biological effects.34,35 For example, 50 nm polystyrene (PS) NPs were dispersed in several tissues of marine copepods after ingestion, while 500 nm and 6 μm PS-MPs were detected only in the digestive tract.36 Forte et al. found that 44 nm of PS-NPs could enter gastric cancer cells faster and more effectively compared with 100 nm, induce up-regulation of interleukin-5 (IL-5) and IL-8 gene expression, and further affect cell morphology, inflammatory gene expression and cell proliferation ability.37 As a result, more attention should be paid to the interaction between NPs and pollutants and relevant research needs to be carried out as soon as possible.
The Organisation for Economic Co-operation and Development (OECD) has launched the adverse outcome pathway (AOP) programme that systematically organizes information into AOP frameworks.38 An ideal AOP was conceptualized as a series of key events and key event relationships that would link a direct molecular initiating event (MIE) to an adverse effect across multiple levels of biological organization,39,40 which has the potential to improve regulatory decision-making through more meaningful use of mechanistic data and greater integration.41 In this study, EHS and PS-NPs were used as target pollutants and zebrafish as test organism to investigate (I) whether the presence of PS-NPs would affect the bioaccumulation of EHS in zebrafish and the transfer to offspring; (II) the effects of EHS alone or in combination with PS-NPs on various physiological parameters at the tissue, cell and molecular levels of parental zebrafish and their offspring; (III) the interactive transgenerational effects of PS-NPs and EHS; (IV) the potential AOP of transgenerational toxic effects of EHS.
2. Materials and methods
2.1 Chemicals and reagents
EHS (purity > 98%) was purchased from AccuStandard Inc (NewHaven, USA). EHS stock solutions and serial dilutions were prepared in dimethyl sulfoxide (DMSO; purity > 99.9%; Amresco, Solon, USA). Fluorescent PS-NPs (100 nm) were supplied by Tianjin Saierqun Technology Co., Ltd (Tianjin, China). Other procurement information is available in the ESI.†
2.2 Characterization of PS-NPs
The surface morphology and dispersion of PS plastic particles were observed by transmission electron microscopy (TEM). Results showed that the PS plastics used in the experimental exposure were spherical granular and evenly dispersed and no obvious particle agglomeration occurred (Fig. 1A). The diameter of PS-NPs in suspension solution was measured by dynamic light scattering analysis and the measured average particle size of PS plastics was 105.7 nm (Fig. 1B), is in line with the physical and chemical parameters provided by the manufacturer.
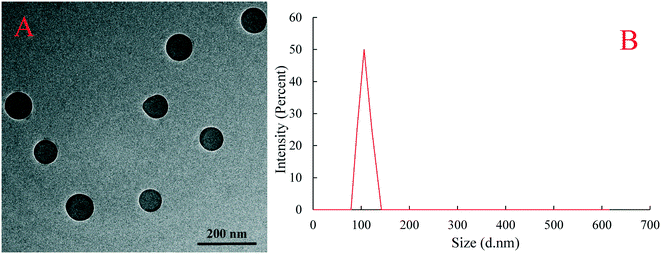 |
| Fig. 1 TEM characterization (A) and hydrodynamic diameter distribution (B) of PS-NPs. | |
2.3 Testing organism and exposure
Three month old zebrafish (AB strain) were purchased from an aquarium shop in Nanjing (China) and were acclimatized for 7 days at 28 ± 1 °C with a light/dark photoperiod of 16 h/8 h. Eighteen zebrafish (6 females and 12 males) were randomly distributed into each glass beakers containing 2 L of solution containing EHS alone (1, 10, and 100 μg L−1, corresponding to 3.99 × 10−9, 3.99 × 10−8, and 3.99 × 10−7 mol L−1, respectively), 10 μg L−1 PS-NPs alone or their mixtures (1 + 10, 10 + 10, 100 + 10 μg L−1) containing 0.01% (v/v) DMSO. The mixed solution of EHS and PS-NPs used in co-exposure groups was all placed 24 h in advance to reach the adsorption equilibrium. A blank control was included by using dechlorinated carbon-filtered water and a solvent control (SC) by using 0.01% (v/v) DMSO. Each treatment and control were replicated 3 times simultaneously. All the exposure media were dechlorinated carbon-filtered water and the exposure solution was replaced once a day. The zebrafish were fed with fairy shrimp twice daily until 28 days and exposed to an artificial day/night cycle (16 h light, 8 h dark) with medium temperature at 28 ± 1 °C (pH 7.4).
After 28 days of exposure, the parental fish (4 males and 8 females) were paired and F1 eggs (100 eggs, n = 3) were collected, of which 50 eggs were immediately frozen at −80 °C for subsequent EHS and PS-NP concentration determination. The remaining 50 eggs were divided into 2 groups: one group was F1(−)washed 5 times with fresh water and transferred to the exposure solutions with only 0.01% (v/v) DMSO to evaluate the transgenerational effect of EHS and PS-NPs alone or in combination; the other group was F1(+) that was continued to be exposed to the same solution as their parents. Then, the blood of parental fish was collected and then the liver, visceral mass and muscle tissues were removed. The muscle tissues were immediately frozen at −80 °C for subsequent EHS and PS-NP concentration determination; the liver tissues were fixed with 4% paraformaldehyde solution immediately for qualitative and quantitative analysis of DNA damage; the visceral mass was partly immediately frozen in liquid nitrogen for analysis of gene expression and partly homogenised in 1
:
9 (w:v) phosphate-buffered saline solution and centrifuged for 10 min at 9000g at 4 °C; and the blood was also immediately centrifuged under the above conditions and all the supernatant was stored at −80 °C until determination. The hatching, malformation and survival rates of F1 were recorded every day, and the juvenile zebrafish of 5 dpf were immediately frozen at −80 °C under testing. All animal procedures were performed in accordance with the Guidelines for Care and Use of Laboratory Animals of “Hohai” University and approved by the Animal Ethics Committee of “Hohai University”.
2.4 Quantification of EHS and PS-NPs in F0 and F1 eggs
An ultrasonic method and KOH digestive method were used to extract EHS and PS-NPs, respectively.18,42 EHS identification and quantification were performed using a Thermo Scientific TSQ Quantum XLS gas chromatography triple quadrupole mass spectrometry (GC-QqQ-MS/MS) instrument equipped with a DB-5MS column (0.25 mm × 30 m × 0.25 μm). The quantification of PS-NPs in the digested solution was performed using a Hitachi F-7000 fluorescence spectrophotometer (Hitachi, Ltd., Tokyo, Japan), with excitation at 488 nm and emission at 518 nm using an external standard method. Detailed measurements can be found in the ESI.†
2.5 Biochemical analysis
Glutathione (GSH), reactive oxygen species (ROS), MDA, triiodothyronine (T3) and thyroxin (T4) contents and superoxide dismutase (SOD), catalase (CAT) and glutathione reductase (GR) activities were all measured using diagnostic enzyme-linked immunosorbent assay kits following the manufacturer's instructions. Protein concentrations were based on bovine serum albumin and determined using the method described by Bradford (1976) at 595 nm.43 SOD, CAT and GR activities were expressed as n (μ) mol mg−1 pro per min. Detailed measurements can be found in the ESI.†
2.6 Quantitative real-time polymerase chain reaction (qRT-PCR) assay
The qRT-PCR data of thyroid hormone receptor (TR) A, TRB, Dio1 and Dio2 were expressed as threshold cycle (Ct) values, and the mRNA levels were calculated using the 2−ΔΔCt method.44 Detailed protocols are available in the ESI.† The gene-specific primers of all the genes were designed based on zebrafish sequences available at NCBI (http://www). The actin gene was selected as the internal standard. Specific information is shown in Table S1.†
2.7 Qualitative and quantitative analysis of DNA damage in liver cells
Qualitative analysis of DNA damage was performed by a 3,3′-diaminobenzidine colorimetric method and expressed as stained labeled dead cells. An avidin–biotin method was used for quantitative analysis of DNA damage and expressed as the number of single base-free sites (AP sites) per 1 × 105 base pair which is the main type of damage produced by oxidative damage. Specific processes of DNA damage (qualitative and quantitative analysis) are available in the ESI† as described by Li et al.45 and Nakamura et al.46
2.8 Statistical analysis
Statistical analysis was performed with SPSS 20.0 (SPSS, Chicago, IL, USA). All data are reported as mean ± standard deviation (SD), and the normality, homogeneity of variance was initially verified using the Shapiro–Wilk and Levene's tests, respectively. Differences between the control and each exposure group were evaluated by one-way analysis of variance (ANOVA) followed by Tukey's test, and statistically different treatments were identified by Dunnett's test. P < 0.05 was considered statistically significant.
3. Results and discussion
3.1 Concentrations of EHS and PS-NPs in F0 and F1
No EHS was detected in the control and PS-NP alone exposure groups, and no PS-NPs were detected in the control and EHS alone exposure groups. As we can see from Fig. 2A, EHS at concentrations of 153.30, 997.48, and 8256.15 ng g−1 wet weight was detected in F0 muscle tissues and 28.12, 131.27, and 1325.76 ng g−1 wet weight in F1(−) eggs, respectively, when parental zebrafish were exposed to 1, 10 and 100 μg L−1 of EHS alone, and F1(+) accumulated higher concentrations of EHS compared with that of F1(−). PS-NPs were also detected in both F0 and F1 in all the test groups containing PS-NPs. Besides, EHS concentrations of both F0 and F1 in the co-exposure groups increased further compared to EHS alone, and there was no significant difference in PS-NP concentration in the same generation.
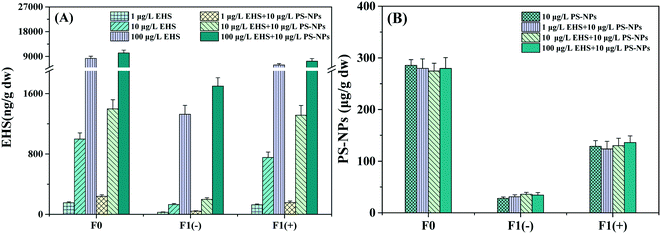 |
| Fig. 2 EHS (A) and PS-NP (B) concentrations in muscle tissues of F0 after 28 days of exposure and F1 eggs. | |
Results showed that both EHS and PS-NPs could accumulate in F0 parents and the EHS cumulative level in co-exposure was higher than that in the corresponding EHS alone exposure group in which PS-NPs were absent. Some scholars thought that the high specific surface area and hydrophobicity of NPs could enable them to adsorb hydrophobic pollutants to form composite pollutants and produce carrier effects.47,48 The composite contaminants first reach the intestinal tract after being ingested by the organism; a portion of the composite contaminants undergo desorption in the intestinal tract and the desorption rate in the biological intestinal tract is 30 times higher than that in the seawater alone; the other portion would penetrate through the intestinal barrier to various tissues and organs, increasing the transfer and bioaccumulation capacity of hydrophobic pollutants as studied by Bakir et al.49 Thus, the carrier effect of PS-NPs may be the main reason for the higher EHS concentration in the co-exposure groups in this study.
The detection of EHS in F1 eggs indicated that EHS could be transferred to the offspring through parental exposure. On the one hand, it may be that the parents absorb a large amount of EHS from the environment, and the offspring constantly absorbs nutrients from the parent, leading to the transfer of EHS from parents to offspring.50 It is also possible that the osmotic transfer of EHS between the tissues and organs in F0 parents causes the transfer of EHS from the intestinal tract or other tissues to the gonadal tissues and yolk sacs, thus reaching the offspring.51 In addition, this study also found that the EHS content in F1 (−) offspring whose parents were co-exposed to EHS and PS-NPs was higher than that of those exposed to EHS alone, and this may also be related to the carrier effect of PS-NPs. Zhao et al. have found that PS-NPs can induce damage to the intestinal barrier and significantly enhance the intestinal permeability of organisms, leading to the easier transfer of PS-NPs and composite contaminants through the intestinal barrier to the target organs (such as gonadal tissues); NPs also can prolong the average defecation cycle which can lead to the increase of PS-NP and EHS adsorbed contents in offspring.52 In fact, there are also some studies showing that NPs have high affinity to plasma proteins. For example, PS-NPs can interact with yolk proteins to promote the transfer of PS-NPs to oocytes and yolk sacs53 and Pitt et al. have detected PS-NPs in the yolk sac of the offspring zebrafish embryos whose parents were exposed to PS-NPs.54 The characteristic that PS-NPs can bind to yolk proteins may also promote the transfer of EHS that adsorbed on NPs from parents' intestine to gonadal tissue as well as offspring embryos, also leading to the increase of EHS content in offspring.
3.2 Growth and developmental toxicity in F1
From Table 1 we can see that there was no significant difference in all indexes among the blank control, solvent control and 10 μg L−1 PS-NP exposure group. The hatching rates of F1(−) at two higher concentrations of parental EHS alone or co-exposure groups were all significantly decreased compared with the control groups and those in the co-exposure group were lower. No significant change was observed in the survival rate of F1(−) in all the treatment groups, while only the highest concentration of mixtures significantly increased the malformation rate of F1(−) compared with the corresponding control groups, mainly pericardial edema, scoliosis and hypoplasia. Moreover, F1(+) appeared to suffer more severe growth and development damage than F1(−) accompanied by the further decrease of hatching rate, the increase of malformation rate and the significant increase of mortality rate, and the co-exposure groups were the most severe.
Table 1 Effects of EHS and NPs alone or in combination exposure on F1 offspring development
Fish |
Test groups (μg L−1) |
3 d Hatching rates (%) |
5 d Malformation rates (%) |
5 d Survival (%) |
Data are presented as mean ± SD (n = 3) and each replicate contained 50 embryos. Significant differences between the exposure groups and control group at P < 0.05. |
|
Blank control |
81.9 ± 2.6 |
0.7 ± 0.6 |
84.2 ± 3.1 |
|
Solvent control |
82.8 ± 2.0 |
1.2 ± 0.5 |
83.0 ± 1.2 |
|
10 μg L−1 PS-NPs |
83.1 ± 1.4 |
1.3 ± 0.5 |
83.8 ± 1.1 |
|
1 μg L−1 EHS |
81.3 ± 1.2 |
2.1 ± 0.8 |
84.4 ± 2.6 |
F1(−) |
10 μg L−1 EHS |
75.2 ± 2.4a |
2.3 ± 0.5 |
82.1 ± 1.4 |
|
100 μg L−1 EHS |
74.3 ± 3.0a |
2.2 ± 0.2 |
82.7 ± 1.3 |
|
1 μg L−1 EHS + 10 μg L−1 PS-NPs |
81.4 ± 2.2 |
1.4 ± 0.8 |
83.8 ± 1.9 |
|
10 μg L−1 EHS + 10 μg L−1 PS-NPs |
68.2 ± 1.9a |
3.3 ± 1.1 |
82.5 ± 2.2 |
|
100 μg L−1 EHS + 10 μg L−1 PS-NPs |
64.1 ± 2.3a |
7.9 ± 2.1a |
80.1 ± 4.6 |
|
Solvent control |
83.1 ± 2.6 |
1.5 ± 0.6 |
83.6 ± 1.6 |
|
10 μg L−1 PS-NPs |
82.9 ± 1.6 |
1.8 ± 0.8 |
82.2 ± 2.1 |
|
1 μg L−1 EHS |
82.0 ± 2.4 |
2.1 ± 0.6 |
83.1 ± 2.6 |
F1(+) |
10 μg L−1 EHS |
72.5 ± 3.0a |
2.5 ± 0.8 |
82.5 ± 1.3 |
|
100 μg L−1 EHS |
70.1 ± 2.8a |
5.9 ± 1.3a |
74.5 ± 2.0a |
|
1 μg L−1 EHS + 10 μg L−1 PS-NPs |
75.1 ± 1.9 |
3.8 ± 0.3 |
80.9 ± 1.8 |
|
10 μg L−1 EHS + 10 μg L−1 PS-NPs |
64.1 ± 3.3a |
6.4 ± 0.3a |
74.5 ± 2.7a |
|
100 μg L−1 EHS + 10 μg L−1 PS-NPs |
60.1 ± 2.5a |
8.4 ± 0.3a |
70.1 ± 5.6a |
The developmental endpoints of offspring, including hatching rate, malformation rate and survival rate are essential parameters for evaluation of the transgenerational effects of exogenous pollutants.55 The present results showed that parental exposure to high levels of EHS may result in a decrease in the hatching rate of the offspring, which is possibly due to the transfer of EHS from the parents to the offspring or the changes in the level of hormones that play a crucial role in the growth and development of zebrafish during the transition from early embryos to larvae, such as thyroid hormones (THs), especially T4.56,57 Studies have found that parental exposure to tris(1,3-dichloro-2-propyl)phosphate can lead to a significant decrease in T4 content, accompanied by a significant increase in malformation and mortality of offspring.58 Han et al. also found that exposure to decabromodiphenyl ether in zebrafish resulted in the decrease in T4 content and body length and increase of malformation rate of offspring.55 Consistent with the above, the decreased T4 content after exposure in this study that will be discussed later may be responsible for the significantly reduced hatching rate in F1 and the significantly reduced survival rate and increased malformation rate only in F1(+) of EHS alone parental exposure. Besides, Davarpanah et al. found that MPs can reduce the inhibition rate of copper-induced growth in Tetraselmis chuii,59 and the mortality rate of Daphnia magna at higher concentrations of MPs and AuNP co-exposure groups was higher than that of AuNPs alone as described by Pacheco et al.60 Similarly, the increased growth and developmental toxicity of F1 in co-exposure in the present study indicated that the presence of PS-NPs may increase the growth and developmental toxicity of EHS, which may be caused by the carrier effect of NPs combining its properties of increasing intestinal permeability, prolonging the biological defecation cycle and binding to yolk proteins.
3.3 Thyroid interference in F0 and F1
As shown in Fig. 3, there was no significant difference in all thyroid-related indexes between the control and PS-NP alone exposure groups. For F0, the Dio1 expression level and T4 content in all the EHS alone and co-exposure groups were significantly decreased, while the Dio2, TRA and TRB expression levels and T3 content exhibited concentration-dependent increases compared with the corresponding control group. Besides, compared with the corresponding EHS alone exposure, the T3 content, and Dio2, TRA, and TRB expression levels in all co-exposure groups were significantly increased and the T4 content and Dio1 expression level were significantly reduced. All indicators of F1(−) showed a similar response to their parents, among which the T3 content and Dio1 and Dio2 expression levels in the co-exposure group showed no significant change at the lowest concentrations and further decreased or increased at higher concentrations compared with EHS alone, while TRA and TRB expression levels were always significantly upregulated and the T4 content significantly decreased. F1(+) appeared to exhibit a more severe thyroid toxicity effect than the corresponding F1(−) by the increased inhibition rate of Dio1 expression and the T4 content and the increased induction rate of the Dio2, TRA and TRB expression levels and T3 content, although some were not significant.
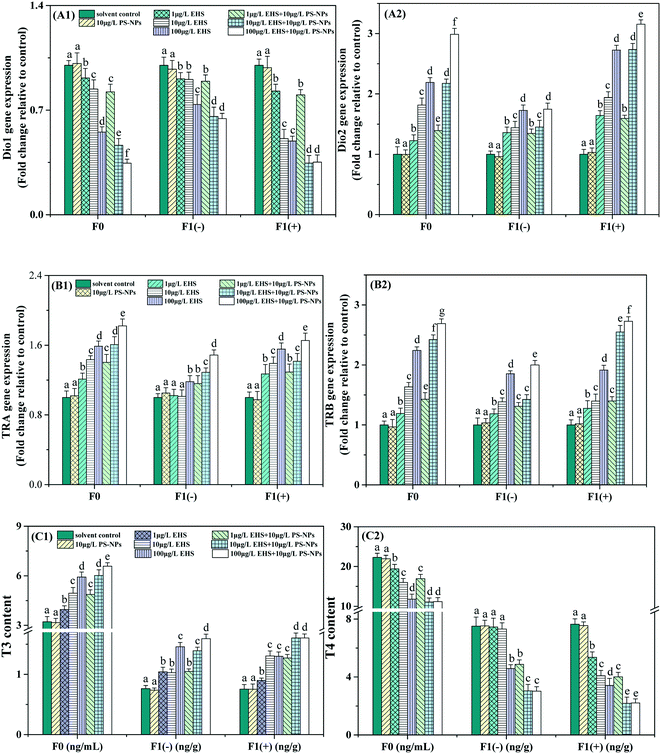 |
| Fig. 3 Dio1 and Dio2 (A1 and A2), TRA and TRB (B1 and B2) expression levels and T3 and T4 contents (C1 and C2) in serum of F0 after 28 days of exposure and homogenates of F1 at 5 dpf. Different letters express significant differences between different treatments of the same generation (P < 0.05). | |
THs play an important role in the differentiation, growth, development and metabolism physiology of early stage fish.56 Both low and excess concentrations of THs may cause delayed growth or severe damage to nerve development and function, and early fish larvae are more sensitive to toxic chemicals than adult fish.61 Therefore, thyroid destruction can be used to reveal the transgenerational toxicity of chemicals.61
Thyroid hormones mainly include T3 and T4.62 T3 is the biologically active form of T4 and T4 needs to be converted to T3 by the action of Dios before it exerts its physiological effects.63 There are three types of Dios, including Dio1, Dio2, and Dio3, among which Dio2 is the decisive enzyme followed by Dio1 and converts T4 into highly bioactive T3, which is then transported to the target tissue through blood, allowing availability of adequate local and systemic T3.64 The decrease of the T4 content and the increase of T3 (Fig. 3C) caused by single EHS in this study may be attributed to the upregulation of Dio2 expression (Fig. 3A2), although Dio1 expression (Fig. 3A1) was down-regulated. Studies have shown that Dio1 gene knockout does not affect developmental processes, while Dio2 gene knockout causes significant developmental delay, suggesting that Dio2 is a major contributor to TH activation during zebrafish embryo/larva thyroid development.65,66 In addition, the parental Dio2 expression levels of all the co-exposure groups were significantly higher than those of EHS alone. Correspondingly, T3 contents increased and T4 contents decreased, respectively, which might be attributed to the carrier effects of PS-NPs that promote the bioaccumulation of EHS in the parents as shown in Fig. 2A.
After THs enter the blood, they bind to the carrier protein in the serum and continue to bind to the specific DNA sequence of the TR that directly acts on the target gene and increases or decreases the expression of this gene, and then induces the cell to respond to THs.57,67 TRA and TRB are two main types of TRs in zebrafish, and their expression is mainly regulated by T3 binding at the transcriptional level.63 The up-regulated expression of TRA and TRB (Fig. 3B) in EHS alone exposure groups suggested that EHS could cause TR abnormalities, affect TH binding and activate the appropriate post-receptor response cascades. The changes of TRA and TRB match the change of T3 content, and this result is consistent with that reported by Shi et al.68 Thus, TRs, like T3, can also serve as a sensitive biomarker for zebrafish exposed to EHS and PS-NPs.
THs have been found in F1 eggs in many studies, which may have originated from the mother.69,70 Studies have shown that the endogenous THs of zebrafish begin to be produced at the time of hatching, and a large number of THs in eggs mainly come from the mother to maintain the TH balance of the offspring and the normal development of the embryo before the endogenous hormones are produced, so maternal thyroid dysfunction may be an important cause of thyroid system disruption in offspring.69,71 For example, Lee et al. found an abnormal thyroid system in offspring zebrafish whose parents were exposed to 0.05 mg L−1 EHMC for three months, and embodied the down-regulation of Dio2 and thyrotropin-releasing hormone gene expression.12 Yu et al. thought that the increase of T4 and T3 contents may be explained by maternal hyperthyroidism caused by pentabromodiphenyl ether exposure, which may also cause thyroid endocrine disorders in the offspring.65 Xu et al. also obtained similar results and the offspring zebrafish were even more sensitive than their parents.10 In this study, we found that the trends of all the thyroid-related indicators in both F1(+) and F1(−) were all consistent with their parents, among which the thyroid disturbance of F1(+) was the most serious that may be due to persistent exposure. The abnormal thyroid function in F1(−) may be due to the transfer of EHS from the parents to the offspring, or it may be explained by the abnormal thyroid system of parents leading to abnormal TH levels and various abnormal genetic indicators in the embryonic period, thus leading to abnormal growth and development of offspring, such as the decrease of hatching rate and the increase of malformation rate as described in section 3.2. In addition, more severe thyroid interference seemed to occur in F1(−) of co-exposure groups than EHS alone which may be caused by the biological carrier effect of PS-NPs, although the T3 content and Dio1 and Dio2 expression in co-exposure at the lowest concentrations did not change significantly compared to EHS alone, which could be interpreted as the increased bioaccumulation of EHS in offspring being promoted by PS-NPs (Fig. 2A) did not cause significant changes in the above indicators.
3.4 Antioxidant responses in F0 and F1
From Fig. 4 we can see that 10 μg L−1 PS-NPs alone significantly increased SOD and CAT activities in F0 but did not significantly change GR activity and GSH, ROS and MDA contents when compared with the controls. All the antioxidant indicators in F0 were significantly increased by different concentrations of EHS alone exposure. Among them, SOD activity showed a tendency to increase first and then decrease, while the others increased with the increase of EHS concentrations and exhibited obvious concentration dependence. For co-exposure, all the antioxidant indicators in F0 were also significantly higher than those in the controls except for the SOD activity at the highest concentrations. Among them, SOD activity decreased with the increase of EHS concentrations and even dropped below the control at the highest concentration of EHS and PS-NPs. CAT and GR activities were firstly increased and then decreased, while GSH, ROS and MDA contents were increased in a concentration-dependent manner.
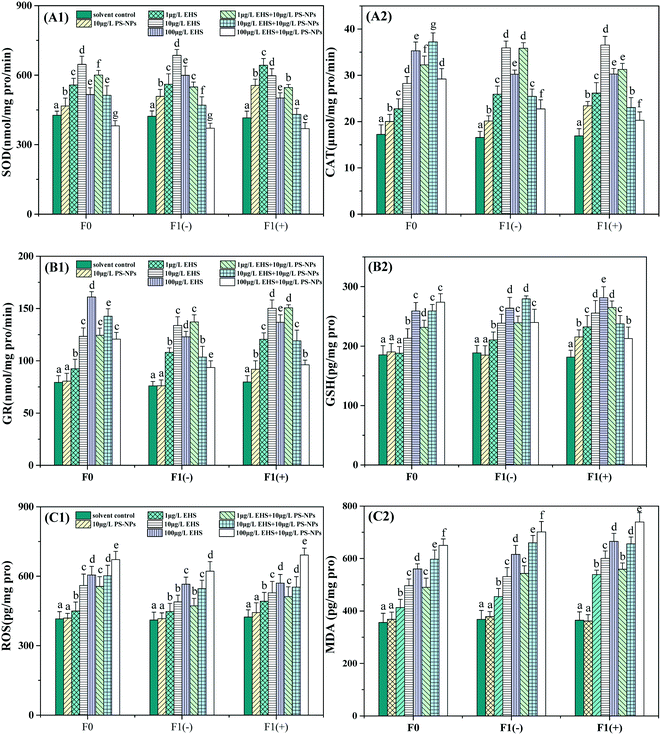 |
| Fig. 4 SOD (A1), CAT (A2) and GR (B1) activity, and GSH (B2), ROS (C1) and MDA (C2) contents in visceral mass of F0 after 28 days of exposure and homogenates of F1 at 5 dpf. Different letters express significant differences between different treatments of the same generation (P < 0.05). | |
Compared to the corresponding EHS alone, GSH, ROS and MDA contents were increased by the mixtures, although not all of them were significantly increased with regard to different concentrations. However, the activities of SOD were significantly increased only at the lowest concentration, while significantly reduced at two higher concentrations. CAT and GR activities were significantly increased at the two lower concentrations and decreased at the highest concentration.
Compared to the F1(−) of EHS alone exposure, the SOD activity of all co-exposure groups was reduced and MDA and ROS contents were increased although some were not significant, while the CAT and GR activities and GSH content were significantly increased at the lowest concentration co-exposure and decreased at the highest concentration co-exposure groups. In addition, compared to F1(−), the ROS and MDA contents of F1(+) of all test groups containing EHS were significantly increased and appeared to undergo stronger oxidative stress, among which the co-exposure was the most serious.
The production and elimination of ROS is usually in a state of dynamic equilibrium under normal physiological conditions, while environmental stress can destroy the balance.72 Increasing ROS levels would cause lipid peroxidation, apoptosis, and various physiological disorders (e.g. metabolic disorders, aging, cancer, and hypertension).73,74 MDA is one of the end products of lipid peroxidation caused by excessive accumulation of ROS, and its accumulation is the central feature and the final signal of oxidative damage. To protect against ROS, the body has developed an antioxidant defense system consisting of enzymes (antioxidant enzymes, SOD, CAT, GR, etc.) and non-enzymatic antioxidants.75
SOD and CAT are considered to be the key first line of defense against oxidative stress in the antioxidant system and play a vital role in combating ROS and protecting cells from oxidative stress.76 SOD can detoxify superoxide radicals into cytotoxic H2O2, while other enzymes such as CAT convert cytotoxic H2O2 into harmless water and oxygen.77 In the present study, the significantly increased SOD and CAT activities (Fig. 4A) and the invariance of ROS and MDA contents (Fig. 4C) in F0 parents exposed to 10 μg L−1 PS-NPs indicated that the NPs might activate the first line of defense to successfully resist mild oxidative stress, and this conclusion is consistent with the findings of Ding et al.33 The induction of SOD and CAT accompanied by the increase of ROS and MDA contents in F0 parents exposed to all the EHS alone indicated that EHS could induce oxidative stress and damage in zebrafish. However, as the EHS concentration increased, SOD activity significantly decreased, and this result is consistent with that reported previously by Shao et al.78 Studies have shown that the inactivation of antioxidant enzyme activity would occur when excess ROS are beyond the typical function of the antioxidant system.79 This is because ROS can oxidize the active site of proteins, resulting in loss of enzyme function and activity, even degrading the enzymes and losing protective ability against cellular superoxide toxicity.79 That is, there may be a threshold value that the enzyme activity is induced when the test concentration is lower than this threshold and is inhibited when the test concentration exceeds this threshold.80 In addition, the ROS and MDA contents of F0 parents in the co-exposure groups were all higher than those of the corresponding EHS alone exposure in which PS-NPs were absent. Considering the time and extent of SOD and CAT activities began to decline, we can speculate that PS-NPs may exacerbate oxidative damage induced by EHS, which may be attributed to PS-NPs promoting the bioaccumulation of EHS in F0 parents or PS-NPs with mild oxidative toxicity having a synergistic effect with EHS as discussed by Lu et al.81 Qu et al. also found that SOD activity induced by co-exposure of MPs and venlafaxine in the loach (Misgurnus anguillicaudatus) was significantly higher than that in venlafaxine alone exposure,82 which is consistent with the results of this study. The discussions of F1 generation were placed at the end of this chapter and all indicators were comprehensively analyzed.
GSH and GSH-related enzymes (such as GR) are the second line of defense of the antioxidant system.75 GSH can control ROS production by working with glutathione peroxidase to provide the reducing equivalent, while oxidative stress caused by exogenous pollutants can lead to GSH depletion.83 GR is an enzyme that maintains the cellular homeostasis of GSH and glutathione disulfide ratio or the redox balance of the cell.84 In this study, the absence of significant changes in GR activity and GSH and MDA contents, and the increase of SOD and CAT activities of F0 in 10 μg L−1 PS-NP alone exposure indicated that the first defense line was activated to successfully defend against oxidative stress, which is in line with the results of Ding et al.33 The increase of GR activity and GSH content of F0 in EHS alone exposure may be explained by the body's second line of defense having been activated, and the continuous increase in MDA content indicates that the body has undergone oxidative damage. Compared to EHS alone, the increase of GR activity in two lower concentrations and decrease in the highest concentration co-exposure groups are probably due to more severe oxidative damage occurring and the GR activity being suppressed and defenseless, and this is also corroborated by the increased ROS and MDA content in co-exposure groups. The above results suggest that PS-NPs may aggravate the oxidative damage caused by EHS for the same reason as changes in SOD and CAT activity.
For F1(−), the increase in ROS and MDA contents compared to the control indicated that parental exposure to EHS would damage the antioxidant defense system of the offspring although the offspring had recovered in clear water for five days. The MDA content of F1(−) was even higher than that of their parents although the amount of EHS transferred from parents to offspring is small which may be due to the more sensitive and weaker defenses of early juvenile fish against contaminants, and this result is also consistent with the results of Yu et al.65 and our previous study.18 Moreover, the SOD, CAT and GR activities of co-exposure groups continued to decrease or even lower than those of the control group, suggesting that more severe oxidative damage may have occurred in F1(−) of the co-exposed group, and this result corroborates with the further increase in ROS and MDA contents which also indicated that PS-NPs possibly synergized the oxidative toxicity of EHS to offspring. The further increase in the ROS and MDA contents of F1(+) compared with F1(−) proved that continued exposure may lead to further aggravation of the oxidative damage, and the results were also consistent with the concentration-dependent effect of EHS.
3.5 DNA damage in F0 zebrafish liver cells.
From Fig. 5, it can be found that there was no significant difference in the number of AP sites in the F0 liver tissues between PS-NP alone exposure and the controls, while the number of AP sites increased by 1.45, 1.99 and 3.43 times in 1, 10 and 100 μg L−1 EHS alone exposure groups and by 2.53, 3.48 and 4.33 times in mixtures, respectively. Fig. 6 shows the extent of apoptosis caused by DNA fragmentation damage. No apoptotic cells were found in the solvent control and PS-NP alone exposure groups, while apoptotic cells were present in EHS alone exposure groups and increased with the increase of EHS concentration, and the number of apoptotic cells increased further in co-exposure groups compared to EHS alone.
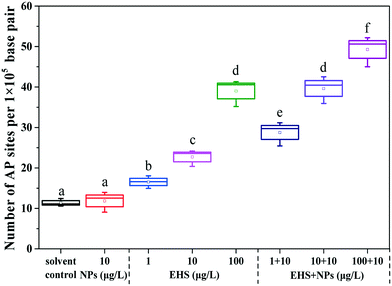 |
| Fig. 5 DNA damage in liver tissues of F0 after 28 days of exposure. The range of each box is from 25th (down) to 75th (up) percentile. The bars below and above the boxes refer to the minimum and maximum values, respectively. The horizontal lines and squares inside the boxes display the median and mean values in each individual treatment. Different letters express significant differences (P < 0.05). | |
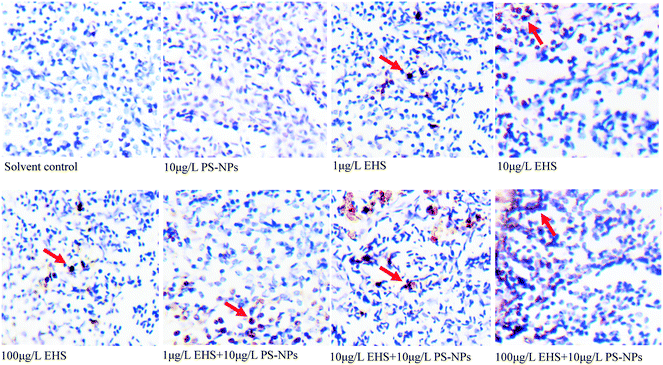 |
| Fig. 6 Cells in liver tissues of F0 after 28 days of exposure and the orange part represents the apoptotic cells. | |
AP sites are the main type of damage produced by oxidative damage and their number can represent the extent of DNA damage.46 The increase in the number of AP sites in all the test groups containing EHS indicates that DNA damage has occurred in these groups which also matches the results in Fig. 6. Studies have shown that DNA damage is mainly caused by ROS which accumulates when oxidative damage occurs.85 Excessive ROS can cause lipid peroxidation, cell membrane damage, mutations in DNA bases, DNA–protein crosslinking, DNA chain rupture, apoptosis or necrosis, and significant increase in MDA content and can eventually lead to DNA damage,86 even disease or genetic effect.85 A good correlation between the production of ROS and damage of embryo cells and DNA after 144 h post-fertilisation exposure to nano ZnO was found by Zhao et al., indicating that ROS induced by nano ZnO could attack DNA and lead to DNA chain breakage and apoptosis.85 Zhu et al. also showed that excessive ROS accumulation may lead to enhanced liver DNA damage in zebrafish.87 In agreement with the above study, the damage of DNA in all the test groups containing EHS in the present study may be caused by excessive ROS and lipid peroxidation induced by EHS. Moreover, the DNA damage degree of co-exposure groups was up to 4.3 times higher than that in EHS alone, which may be closely related to the aggravation of oxidative damage caused by co-exposure, and may also be due to PS-NPs synergizing the oxidative stress effects of EHS or the carrier effects of PS-NPs.
3.6 AOP analysis
Based on a range of key events, AOP provides a framework for predicting the association between direct molecular priming events and adverse outcomes at the biological level and can be used to assess the hazards of toxic substances.39,88 In this study, the destruction of thyroid hormone homeostasis and the accumulation of MDA and ROS in this study are MIEs. These MIEs can lead to an abnormal thyroid system and oxidative damage of F0 and F1, DNA damage and apoptosis of F0 and abnormal growth and development of F1, which are considered to be key events that may synergistically produce adverse outcomes of transgenerational toxic effects. Furthermore, the presence of MPs leads to more severe adverse outcomes than EHS exposure alone. Altogether, AOP analysis is a useful tool for conceptualizing interactions between potential mechanisms, which should be the focus of future research work on aquatic toxicity of pollutants.89 The proposed AOPs for EHS and PS-NP co-exposure are shown in Fig. 7.
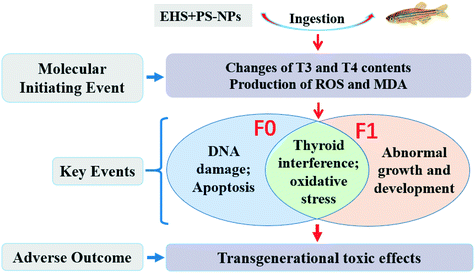 |
| Fig. 7 Proposed AOPs for EHS and PS-NP co-exposure. | |
4. Conclusions
Based on the above findings, PS-NPs can act as a carrier of EHS to promote the bioaccumulation of EHS in zebrafish and its transfer from parents to offspring. EHS can induce growth and developmental toxicity, and oxidative damage and interfere with the thyroid system in both parent and offspring zebrafish, and the transgenerational effects may be due to the EHS transmitted by the parents, or the changes of the physiological/biochemical indexes of their parents. In addition, both parents and offspring co-exposed to EHS showed more intense biochemical responses than EHS alone exposure, which possibly due to the PS-NP itself or its carrier effect promoting the bioaccumulation of EHS in the parents and its delivery to the offspring. Overall, this study highlights the influences of NPs on the bioavailability and transgenerational effects of emerging organic contaminants.
Author contributions
Ranran Zhou: Methodology, formal analysis, writing – original draft, investigation. Guanghua Lu: Conceptualization, writing – original draft, project administration, funding acquisition. Zhenhua Yan: Data curation, supervision. Runren Jiang: Formal analysis, investigation. Yu Sun: Validation, investigation. Peng Zhang: Investigation.
Conflicts of interest
The authors declare no competing financial interests.
Acknowledgements
This research was funded by the National Natural Science Foundation of China (No. 51879228, 51979080), the National Science Funds for Creative Research Groups of China (No. 51421006), and the Priority Academic Programme Development of Jiangsu Higher Education Institutions.
References
- F. Mao, Y. He and K. Y. H. Gin, Occurrence and fate of benzophenone-type UV filters in aquatic environments: a review, Environ. Sci.: Water Res. Technol., 2019, 5, 209–223 RSC.
- X. Peng, Z. Zhu, S. Xiong, Y. Fan and C. Tang, Tissue distribution, growth dilution, and species-specific bioaccumulation of organic ultraviolet absorbents in wildlife freshwater fish in the Pearl River catchment, China, Environ. Toxicol. Chem., 2020, 39, 343–351 CrossRef CAS.
- M. M. Tsui, J. C. Lam, T. Ng, P. O. Ang, M. B. Murphy and P. K. Lam, Occurrence, distribution, and fate of organic UV filters in coral communities, Environ. Sci. Technol., 2017, 51, 4182–4190 CrossRef CAS.
- P. C. Ku, T. Y. Liu, S. H. Lee, T. A. Kun and W. H. Wang, An environmentally friendly strategy for determining organic ultraviolet filters in seawater using liquid-phase microextraction with liquid chromatography–tandem mass spectrometry, Environ. Sci. Pollut. Res. Int., 2020, 27, 9818–9825 CrossRef CAS.
- Z. Yan, H. Yang, H. Dong, B. Ma, H. Sun, T. Pan, R. Jiang, R. Zhou, J. Shen and J. Liu, Occurrence and ecological risk assessment of organic micropollutants in the lower reaches of the Yangtze River, China: A case study of water diversion, Environ. Pollut., 2018, 239, 223–232 CrossRef CAS.
- S. Cunha, L. Trabalón, S. Jacobs, M. Castro, M. Fernandez-Tejedor, K. Granby, W. Verbeke, C. Kwadijk, F. Ferrari and J. Robbens, UV-filters and musk fragrances in seafood commercialized in Europe Union: Occurrence, risk and exposure assessment, Environ. Res., 2018, 161, 399–408 CrossRef CAS.
- C. Apel, J. Tang and R. Ebinghaus, Environmental occurrence and distribution of organic UV stabilizers and UV filters in the sediment of Chinese Bohai and Yellow Seas, Environ. Pollut., 2018, 235, 85–94 CrossRef CAS.
- M. Castro, J. Fernandes, A. Pena and S. Cunha, Occurrence, profile and spatial distribution of UV-filters and musk fragrances in mussels from Portuguese coastline, Mar. Environ. Res., 2018, 138, 110–118 CrossRef CAS.
- I. Tarazona, A. Chisvert and A. Salvador, Development of a gas chromatography-mass spectrometry method for the determination of ultraviolet filters in beach sand samples, Anal. Methods, 2014, 6, 7772–7780 RSC.
- C. Xu, L. Niu, J. Liu, X. Sun, C. Zhang, J. Ye and W. Liu, Maternal exposure to fipronil results in sulfone metabolite enrichment and transgenerational toxicity in zebrafish offspring: Indication for an overlooked risk in maternal transfer?, Environ. Pollut., 2019, 246, 876–884 CrossRef CAS.
- F. Cao, H. Li, F. Zhao, P. Wu, L. Qian, L. Huang, S. Pang, C. J. Martyniuk and L. Qiu, Parental exposure to azoxystrobin causes developmental effects and disrupts gene expression in F1 embryonic zebrafish (Danio rerio), Sci. Total Environ., 2019, 646, 595–605 CrossRef CAS.
- I. Lee, J. Lee, D. Jung, S. Kim and K. Choi, Two-generation exposure to 2-ethylhexyl 4-methoxycinnamate (EHMC) in Japanese medaka (Oryzias latipes) and its reproduction and endocrine related effects, Chemosphere, 2019, 228, 478–484 CrossRef CAS.
- R. Rodriguez-Gomez, A. Zafra-Gomez, N. Dorival-Garcia, O. Ballesteros and A. Navalon, Determination of benzophenone-UV filters in human milk samples using ultrasound-assisted extraction and clean-up with dispersive sorbents followed by UHPLC-MS/MS analysis, Talanta, 2015, 134, 657–664 CrossRef CAS.
- J. Valle-Sistac, D. Molins-Delgado, M. Díaz, L. Ibáñez, D. Barceló and M. S. Díaz-Cruz, Determination of parabens and benzophenone-type UV filters in human placenta. First description of the existence of benzyl paraben and benzophenone-4, Environ. Int., 2016, 88, 243–249 CrossRef CAS.
- A. Bergman, J. J. Heindel, S. Jobling, K. Kidd and T. R. Zoeller, State of the science of endocrine disrupting chemicals, World Health Organ., 2012, 2013 Search PubMed.
- M. Krause, H. Frederiksen, K. Sundberg, F. Jorgensen, L. Jensen, P. Norgaard, C. Jorgensen, P. Ertberg, A. Juul and K. Drzewiecki, Presence of benzophenones commonly used as UV filters and absorbers in paired maternal and fetal samples, Environ. Int., 2018, 110, 51–60 CrossRef CAS.
- M. Colicchia, L. Campagnolo, E. Baldini, S. Ulisse, H. Valensise and C. Moretti, Molecular basis of thyrotropin and thyroid hormone action during implantation and early development, Hum. Reprod. Update, 2014, 20, 884–904 CrossRef CAS.
- R. Zhou, G. Lu, Z. Yan, R. Jiang, J. Shen and X. Bao, Parental transfer of ethylhexyl methoxy cinnamate and induced biochemical responses in zebrafish, Aquat. Toxicol., 2019, 206, 24–32 CrossRef CAS.
- K. Fent, P. Y. Kunz and E. Gomez, UV filters in the aquatic environment induce hormonal effects and affect fertility and reproduction in fish, Chimia, 2008, 62, 368–375 CrossRef CAS.
- E. Gomez, M. Bachelot, C. Boillot, D. Munaron, S. Chiron, C. Casellas and H. Fenet, Bioconcentration of two pharmaceuticals (benzodiazepines) and two personal care products (UV filters) in marine mussels (Mytilus galloprovincialis) under controlled laboratory conditions, Environ. Sci. Pollut. Res. Int., 2012, 19, 2561–2569 CrossRef CAS.
- C. Li, R. Busquets and L. C. Campos, Assessment of microplastics in freshwater systems: A review, Sci. Total Environ., 2020, 707, 135578 CrossRef CAS.
- L. C. De Sa, M. Oliveira, F. Ribeiro, T. L. Rocha and M. N. Futter, Studies of the effects of microplastics on aquatic organisms: What do we know and where should we focus our efforts in the future?, Sci. Total Environ., 2018, 645, 1029–1039 CrossRef CAS.
- X. Xiong, C. Wu, J. Elser, Z. Mei and Y. Hao, Occurrence and fate of microplastic debris in middle and lower reaches of the Yangtze River– From inland to the sea, Sci. Total Environ., 2019, 659, 66–73 CrossRef CAS.
- E. L. Ng, E. H. Lwanga, S. M. Eldridge, P. Johnston, H. W. Hu, V. Geissen and D. Chen, An overview of microplastic and nanoplastic pollution in agroecosystems, Sci. Total Environ., 2018, 627, 1377–1388 CrossRef CAS.
- Y. Chae and Y. J. An, Effects of food presence on microplastic ingestion and egestion in Mytilus galloprovincialis, Chemosphere, 2020, 240, 124855 CrossRef CAS.
- A. A. Horton, L. K. Newbold, A. M. Palacio-Cortés, D. J. Spurgeon, M. G. Pereira, H. Carter, H. S. Gweon, M. G. Vijver, P. M. Bodegom and M. A. N. Silva, Accumulation of polybrominated diphenyl ethers and microbiome response in the great pond snail Lymnaea stagnalis with exposure to nylon (polyamide) microplastics, Ecotoxicol. Environ. Saf., 2020, 188, 109882 CrossRef CAS.
- Y. Zhang and G. G. Goss, Potentiation of polycyclic aromatic hydrocarbon uptake in zebrafish embryos by nanoplastics, Environ. Sci.: Nano, 2020, 7, 1730–1741 RSC.
- A. A. Koelmans, A. Bakir, G. A. Burton and C. R. Janssen, Microplastic as a vector for chemicals in the aquatic environment: critical review and model-supported reinterpretation of empirical studies, Environ. Sci. Technol., 2016, 50, 3315–3326 CrossRef CAS.
- O. S. Alimi, J. Farner Budarz, L. M. Hernandez and N. Tufenkji, Microplastics and nanoplastics in aquatic environments: aggregation, deposition, and enhanced contaminant transport, Environ. Sci. Technol., 2018, 52, 1704–1724 CrossRef CAS.
- Z. Duan, X. Duan, S. Zhao, X. Wang, J. Wang, Y. Liu, Y. Peng, Z. Gong and L. Wang, Barrier function of zebrafish embryonic chorions against microplastics and nanoplastics and its impact on embryo development, J. Hazard. Mater., 2020, 395, 122621 CrossRef CAS.
- A. A. Koelmans, E. Besseling and E. M. Foekema, Leaching of plastic additives to marine organisms, Environ. Pollut., 2014, 187, 49–54 CrossRef CAS.
- S. Zhang, J. Ding, R. M. Razanajatovo, H. Jiang, H. Zou and W. Zhu, Interactive effects of polystyrene microplastics and roxithromycin on bioaccumulation and biochemical status in the freshwater fish red tilapia (Oreochromis niloticus), Sci. Total Environ., 2019, 648, 1431–1439 CrossRef CAS.
- J. Ding, S. Zhang, R. M. Razanajatovo, H. Zou and W. Zhu, Accumulation, tissue distribution, and biochemical effects of polystyrene microplastics in the freshwater fish red tilapia (Oreochromis niloticus), Environ. Pollut., 2018, 238, 1–9 CrossRef CAS.
- S. Lambert and M. Wagner, Characterisation of nanoplastics during the degradation of polystyrene, Chemosphere, 2016, 145, 265–268 CrossRef CAS.
-
A. A. Koelmans, E. Besseling and W. J. Shim, Nanoplastics in the aquatic environment, Critical review, Marine anthropogenic litter, Springer, Cham, 2015, pp. 325–340 Search PubMed.
- C. B. Jeong, H. M. Kang, M. C. Lee, D. H. Kim, J. Han, D. S. Hwang, S. Souissi, S. J. Lee, K. H. Shin and H. G. Park, Adverse effects of microplastics and oxidative stress-induced MAPK/Nrf2 pathway-mediated defense mechanisms in the marine copepod Paracyclopina nana, Sci. Rep., 2017, 7, 41323 CrossRef CAS.
- M. Forte, G. Iachetta, M. Tussellino, R. Carotenuto, M. Prisco, M. D. Falco, V. Laforgia and S. Valiante, Polystyrene nanoparticles internalization in human gastric adenocarcinoma cells, Toxicol. In Vitro, 2016, 31, 126–136 CrossRef CAS.
-
OECD, Adverse outcome pathways, molecular screening and toxicogenomics, Organization for the Economic Co-Operation and Development, Paris, 2015 Search PubMed.
- L. D. Villeneuve, D. Crump, N. G. Reyero, M. Hecker, T. H. Hutchinson, C. A. LaLone, B. Landesmann, T. Lettieri, S. Munn, M. Nepelska, M. A. Ottinger, L. Vergauwen and M. Whelan, Adverse outcome pathway (AOP) development I: strategies and principles, Toxicol. Sci., 2014, 142, 312–320 CrossRef.
- E. B. Muller, S. Lin and R. M. Nisbet, Quantitative adverse outcome pathway analysis of hatching in zebrafish with cuo nanoparticles, Environ. Sci. Technol., 2015, 49, 11817–11824 CrossRef CAS.
- G. Steve and R. Paul, The role of chemistry in developing understanding of adverse outcome pathways and their application in risk assessment, Toxicol. Res., 2013, 2, 299–307 CrossRef.
- F. Li, F. Li, X. Hou, X. Luo, H. Tu, Y. Zou, C. Sun, M. Shi and H. Zheng, Comparison of six digestion methods on fluorescent intensity and morphology of the fluorescent polystyrene beads, Mar. Pollut. Bull., 2018, 131, 515–524 CrossRef CAS.
- M. M. Bradford, A rapid and sensitive method for the quantitation of microgram quantities of protein utilizing the principle of protein-dye binding, Anal. Biochem., 1976, 72, 248–254 CrossRef CAS.
- K. J. Livak and T. D. Schmittgen, Analysis of relative gene expression data using real-time quantitative PCR and the 2−ΔΔCT method, Methods, 2001, 25, 402–408 CrossRef CAS.
- X. Li, M. Zhang and W. Tang, Effects of melatonin on streptozotocin-induced retina neuronal apoptosis in high blood glucose rat, Neurochem. Res., 2013, 38, 669–676 CrossRef CAS.
- J. Nakamura, V. E. Walker, P. B. Upton, S. Y. Chiang, Y. W. Kow and J. A. Swenberg, Highly sensitive apurinic/apyrimidinic site assay can detect spontaneous and chemically induced depurination under physiological conditions, Cancer Res., 1998, 58, 222–225 CAS.
- C. Wu, K. Zhang, X. Huang and J. Liu, Sorption of pharmaceuticals and personal care products to polyethylene debris, Environ. Sci. Pollut. Res. Int., 2016, 23, 8819–8826 CrossRef CAS.
- H. Lee, W. J. Shim and J. H. Kwon, Sorption capacity of plastic debris for hydrophobic organic chemicals, Sci. Total Environ., 2014, 470, 1545–1552 CrossRef.
- A. Bakir, S. J. Rowland and R. C. Thompson, Enhanced desorption of persistent organic pollutants from microplastics under simulated physiological conditions, Environ. Pollut., 2014, 185, 16–23 CrossRef CAS.
- X. Han, E. N. Lei, M. H. Lam and R. Wu, A whole life cycle assessment on effects of waterborne PBDEs on gene expression profile along the brain-pituitary-gonad axis and in the liver of zebrafish, Mar. Pollut. Bull., 2011, 63, 160–165 CrossRef CAS.
- J. Chen, Tissue distributions and seasonal dynamics of the hepatotoxic microcystins-LR and -RR in two freshwater shrimps, Palaemon modestus and Macrobrachium nipponensis, from a large shallow, eutrophic lake of the subtropical China, Toxicon, 2005, 45, 615–625 CrossRef CAS.
- L. Zhao, M. Qu, G. Wong and D. Wang, Transgenerational toxicity of nanopolystyrene particles in the range of μg L−1 in the nematode Caenorhabditis elegans, Environ. Sci.: Nano, 2017, 4, 2356–2366 RSC.
- G. Rossi, J. Barnoud and L. Monticelli, Polystyrene nanoparticles perturb lipid membranes, J. Phys. Chem. Lett., 2013, 5, 241–246 CrossRef.
- J. A. Pitt, R. Trevisan, A. Massarsky, J. S. Kozal, E. D. Levin and R. T. Giulio, Maternal transfer of nanoplastics to offspring in zebrafish (Danio rerio): A case study with nanopolystyrene, Sci. Total Environ., 2018, 643, 324–334 CrossRef CAS.
- Z. Han, Y. Li, S. Zhang, N. Song, H. Xu, Y. Dang, C. Liu, J. P. Giesy and H. Yu, Prenatal transfer of decabromodiphenyl ether (BDE-209) results in disruption of the thyroid system and developmental toxicity in zebrafish offspring, Aquat. Toxicol., 2017, 190, 46–52 CrossRef CAS.
- L. Q. Yu, J. Deng, X. J. Shi, C. S. Liu, Y. Ke and B. S. Zhou, Exposure to DE-71 alters thyroid hormone levels and gene transcription in the hypothalamic-pituitary-thyroid axis of zebrafish larvae, Aquat. Toxicol., 2010, 97, 226–233 CrossRef CAS.
- Y. W. Liu and W. K. Chan, Thyroid hormones are important for embryonic to larval transitory phase in zebrafish, Differentiation, 2002, 70, 36–45 CrossRef CAS.
- Q. Wang, N. L. S. Lai, X. Wang, Y. Guo, P. K. S. Lam, J. C. W. Lam and B. Zhou, Bioconcentration and transfer of the organophorous flame retardant 1, 3-dichloro-2-propyl phosphate causes thyroid endocrine disruption and developmental neurotoxicity in zebrafish larvae, Environ. Sci. Technol., 2015, 49, 5123–5132 CrossRef CAS.
- E. Davarpanah and L. Guilhermino, Single and combined effects of microplastics and copper on the population growth of the marine microalgae Tetraselmis chuii, Estuarine, Coastal Shelf Sci., 2015, 167, 269–275 CrossRef CAS.
- A. Pacheco, A. Martins and L. Guilhermino, Toxicological interactions induced by chronic exposure to gold nanoparticles and microplastics mixtures in Daphnia magna, Sci. Total Environ., 2018, 628, 474–483 CrossRef.
- P. P. Jia, Y. B. Ma, C. J. Lu, Z. Mirza, W. Zhang, Y. F. Jia, W. G. Li and D. S. Pei, The effects of disturbance on Hypothalamus-Pituitary-Thyroid (HPT) axis in zebrafish larvae after exposure to DEHP, PLoS One, 2016, 11, e0155762 CrossRef.
- W. K. Chan and K. M. Chan, Disruption of the hypothalamic-pituitary-thyroid axis in zebrafish embryo–larvae following waterborne exposure to BDE-47, TBBPA and BPA, Aquat. Toxicol., 2012, 108, 106–111 CrossRef CAS.
- S. Liu, J. Chang, Y. Zhao and G. Zhu, Changes of thyroid hormone levels and related gene expression in zebrafish on early life stage exposure to triadimefon, Environ. Toxicol. Pharmacol., 2011, 32, 472–477 CrossRef CAS.
- A. Orozco and R. C. Valverde, Thyroid hormone deiodination in fish, Thyroid, 2005, 15, 799–813 CrossRef CAS.
- L. Yu, J. C. Lam, Y. Guo, R. S. Wu, P. K. Lam and B. Zhou, Parental transfer of polybrominated diphenyl ethers (PBDEs) and thyroid endocrine disruption in zebrafish, Environ. Sci. Technol., 2011, 45, 10652–10659 CrossRef CAS.
- C. N. Walpita, A. D. Crawford, E. D. Janssens, S. Van and V. M. Darras, Type 2 iodothyronine deiodinase is essential for thyroid hormone-dependent embryonic development and pigmentation in zebrafish, Endocrinology, 2009, 150, 530–539 CrossRef CAS.
- J. Eales and S. Brown, Measurement and regulation of thyroidal status in teleost fish, Rev. Fish. Biol. Fisher., 1993, 3, 299–347 CrossRef.
- X. Shi, C. Liu, G. Wu and B. Zhou, Waterborne exposure to PFOS causes disruption of the hypothalamus-pituitary-thyroid axis in zebrafish larvae, Chemosphere, 2009, 77, 1010–1018 CrossRef CAS.
- D. M. Power, L. Llewellyn, M. Faustino, M. A. Nowell, B. T. Björnsson, I. E. Einarsdóttir, A. V. Canario and G. E. Sweeney, Thyroid hormones in growth and development of fish, Comp. Biochem. Physiol., Part C: Toxicol. Pharmacol., 2001, 130, 447–459 CAS.
- M. Tagawa, M. Tanaka, S. Matsumoto and T. Hirano, Thyroid hormones in eggs of various freshwater, marine and diadromous teleosts and their changes during egg development, Fish Physiol. Biochem., 1990, 8, 515–520 CrossRef CAS.
- W. Thomas, L. Klaus, M. Marina, F. Jack, B. Michael, S. W. Wilson and K. B. Rohr, Pax2.1 is required for the development of thyroid follicles in zebrafish, Development, 2002, 129, 3751–3760 Search PubMed.
- K. W. Samarakoon, S. H. Cha, J. H. Lee and Y. J. Jeon, The growth, innate immunity and protection against H2O2-induced oxidative damage of a chitosan-coated diet in the olive flounder Paralichthys olivaceus, Fish. Aquat. Sci., 2013, 16, 149–158 CAS.
- S. H. Yan, J. H. Wang, L. S. Zhu and A. M. Chen, Wang J, Thiamethoxam induces oxidative stress and antioxidant response in zebrafish (Danio Rerio) livers, Environ. Toxicol., 2016, 31, 2006–2015 CrossRef CAS.
- M. Oliveira, V. Maria, I. Ahmad, A. Serafim, M. Bebianno, M. Pacheco and M. Santos, Contamination assessment of a coastal lagoon (Ria de Aveiro, Portugal) using defence and damage biochemical indicators in gill of Liza aurata-an integrated biomarker approach, Environ. Pollut., 2009, 157, 959–967 CrossRef CAS.
- C. Li, L. Qin, R. Qu, P. Sun and Z. Wang, Responses of antioxidant defense system to polyfluorinated dibenzo-p-dioxins (PFDDs) exposure in liver of freshwater fish Carassius auratus, Ecotoxicol. Environ. Saf., 2016, 126, 170–176 CrossRef CAS.
- Q. L. Chen, Y. L. Sun, Z. H. Liu and Y. W. Li, Sex-dependent effects of subacute mercuric chloride exposure on histology, antioxidant status and immune-related gene expression in the liver of adult zebrafish (Danio rerio), Chemosphere, 2017, 188, 1–9 CrossRef CAS.
- J. L. Zheng, S. S. Yuan, C. W. Wu and Z. M. Lv, Acute exposure to waterborne cadmium induced oxidative stress and immunotoxicity in the brain, ovary and liver of zebrafish (Danio rerio), Aquat. Toxicol., 2016, 180, 36–44 CrossRef CAS.
- B. Shao, L. Zhu, M. Dong, J. Wang, H. Xie, Q. Zhang, Z. Du and S. Zhu, DNA damage and oxidative stress induced by endosulfan exposure in zebrafish (Danio rerio), Ecotoxicology, 2012, 21, 1533–1540 CrossRef CAS.
- C. N. Zhang, J. L. Zhang, H. T. Ren, B. H. Zhou and Q. J. Wu, Sun P, Effect of tributyltin on antioxidant ability and immune responses of zebrafish (Danio rerio), Ecotoxicol. Environ. Saf., 2017, 138, 1–8 CrossRef CAS.
- S. Wu, G. Ji, J. Liu, S. Zhang, Y. Gong and L. Shi, TBBPA induces developmental toxicity, oxidative stress, and apoptosis in embryos and zebrafish larvae (Danio rerio), Environ. Toxicol., 2016, 31, 1241–1249 CrossRef CAS.
- K. Lu, R. Qiao, H. An and Y. Zhang, Influence of microplastics on the accumulation and chronic toxic effects of cadmium in zebrafish (Danio rerio), Chemosphere, 2018, 202, 514–520 CrossRef CAS.
- H. Qu, R. Ma, B. Wang, J. Yang, L. Duan and G. Yu, Enantiospecific toxicity, distribution and bioaccumulation of chiral antidepressant venlafaxine and its metabolite in loach (Misgurnus anguillicaudatus) co-exposed to microplastic and the drugs, J. Hazard. Mater., 2019, 370, 203–211 CrossRef CAS.
- M. E. Nunes, T. E. Müller, C. Murussi, A. M. Do, J. L. Gomes, A. T. Marins, J. Leitemperger, C. C. Rodrigues, T. L. Fiuza and M. D. Costa, Oxidative effects of the acute exposure to a pesticide mixture of cypermethrin and chlorpyrifos on carp and zebrafish-a comparative study, Comp. Biochem. Physiol., Part C: Toxicol. Pharmacol., 2018, 206, 48–53 Search PubMed.
- S. Sarkar, S. Mukherjee, A. Chattopadhyay and S. Bhattacharya, Low dose of arsenic trioxide triggers oxidative stress in zebrafish brain: expression of antioxidant genes, Ecotoxicol. Environ. Saf., 2014, 107, 1–8 CrossRef CAS.
- X. Zhao, S. Wang, Y. Wu, H. You and L. Lv, Acute ZnO nanoparticles exposure induces developmental toxicity, oxidative stress and DNA damage in embryo-larval zebrafish, Aquat. Toxicol., 2013, 136, 49–59 CrossRef.
- S. Yan, J. Wang, L. Zhu, A. Chen and J. Wang, Toxic effects of nitenpyram on antioxidant enzyme system and DNA in zebrafish (Danio rerio) livers, Ecotoxicol. Environ. Saf., 2015, 122, 54–60 CrossRef CAS.
- L. Zhu, X. Dong, H. Xie, J. Wang, J. Wang, J. Su and C. Yu, DNA damage and effects on glutathione-S-transferase activity induced by atrazine exposure in zebrafish (Danio rerio), Environ. Toxicol., 2011, 26, 480–488 CrossRef CAS.
- Y. B. Ma, C. J. Lu, M. Junaid, P. P. Jia, L. Yang, J. H. Zhang and D. S. Pei, Potential adverse outcome pathway (AOP) of silver nanoparticles mediated reproductive toxicity in zebrafish, Chemosphere, 2018, 207, 320–328 CrossRef CAS.
- E. R. Garman, The mechanisms of nickel toxicity in aquatic environments: An adverse outcome pathway analysis, Environ. Toxicol. Chem., 2017, 36, 1128–1137 CrossRef.
Footnote |
† Electronic supplementary information (ESI) available. See DOI: 10.1039/d0en00952k |
|
This journal is © The Royal Society of Chemistry 2021 |