Polystyrene micro- and nanoplastics affect locomotion and daily activity of Drosophila melanogaster†
Received
10th September 2020
, Accepted 7th December 2020
First published on 6th January 2021
Abstract
Microplastics and nanoplastics are emerging contaminants in aquatic and terrestrial environments. Due to the challenges in detecting these small plastics in complex soil matrices, data about their abundance in terrestrial environments are scarce and our knowledge of their impacts on terrestrial organisms is severely lacking. Here, we introduced a well-established terrestrial model organism – the fruit fly (Drosophila melanogaster) – to study the chronic adverse effects of model micro- and nanoplastics. The toxicity of model dialyzed polystyrene spheres (1 μm and 20 nm) was assessed via dietary exposures to a wide range of concentrations (0.01 to 100 ppm). In a first experiment, flies were exposed from larval to adult stage for 13 days. Uptake of both particle sizes in the gastrointestinal tract of larvae was observed, with depuration times of 1 and 24 h for 20 nm and 1 μm particles, respectively. In adults, only 1 μm particles accumulated to detectable levels and nano computed tomography imaging revealed intestinal damage. Both micro- and nanoplastics significantly affected locomotion while mortality, development, and fertility were not significantly affected. A second 8 day experiment focused on the daily behavior of adults exposed to micro- and nanoplastics. We observed no effect on circadian rhythms but an increase in daily activity after micro- and nanoplastic exposure at 50 ppm, likely in response to a reduction in nutrient absorption due to the change in diet or intestinal damage. Overall, dietary exposures to clean spherical polystyrene micro- and nanoplastics caused low toxicity but significant sublethal effects in the fruit fly. This study establishes a baseline understanding of the impacts of model micro- and nanoplastic exposure to the fruit fly and motivates the need for further work focusing on naturally weathered plastic debris.
Environmental significance
With the emergence and ubiquity of micro- and nanoplastic pollution, there is concern about its impact on the environment. High microplastic concentrations (67 500 ppm) have been detected in soils and, with the increasing use of plastic mulch and biosolids, agricultural lands are expected to accumulate these particles quickly, presenting potential health implications for all organisms including humans. However, toxicity data for terrestrial organisms is lacking and nanoplastics have been largely ignored. To fill this knowledge gap, we assessed the toxicity of model micro- and nanoplastics using an environmentally relevant terrestrial model, Drosophila melanogaster. Observed effects on locomotor ability, behavior, intestinal tissue and stress gene expression motivate the need for follow-up studies using naturally weathered plastic debris.
|
1. Introduction
As a highly versatile material, plastic has found uses in a seemingly endless list of applications from construction, electronics, clothing, food packaging, and even cosmetics. As a result, demand is extremely high and yearly global production reached 360 million metric tonnes in 2018.1 Of this, 70% ends up as waste with the majority (79%) going to landfills or directly into the environment.2 The billions of metric tonnes of plastic pollution that has accumulated worldwide have developed into a problem that goes beyond the visible debris. Microplastics and nanoplastics are generally defined as plastic pieces with sizes between ∼100 nm–5 mm and <∼100 nm,3 respectively, that can be introduced to the environment from weathering of bulk plastic or the release of primary plastic particles developed for commercial and industrial use.4,5 These small fragments compose the vast majority of plastic pollution and their size allows them to be carried to even the most remote locations globally via ocean currents and winds.6 Inevitably, a wide variety of species, including humans, has been exposed to these fragments.4 Nanoplastics can pose additional risks as they have been shown to pass through biological barriers and accumulate in tissues and organs, triggering physiological distress, diminished reproductive fitness and early mortality.7 These nanoparticles can also act as carriers for other contaminants that can enact toxic effects of their own.8,9
Although the toxicity of micro- and nanoplastics has been extensively studied in aquatic organisms with various effects on growth, development, behavior, reproduction, and mortality being observed across a wide range of aquatic species,10 studies in terrestrial systems are far less abundant despite soils being one of the largest sinks for plastics.11 Plastics enter soils through aerial deposition,12 littering, and the application of plastic films and sewage sludge to agricultural lands.8,11,13,14 Sludges retain up to 99% of microplastics that pass through wastewater treatment plants (WWTPs), with 15
385 particles per kg having been measured in samples from WWTPs in Ireland.8,15 Sludge has been estimated to add up to 300 thousand tonnes of microplastics to North American farmlands per year.13 Farmlands that use modern practices (e.g., plastic films and sludge) have reported higher microplastics concentrations (up to 42
960 particles per kg)16 than those that do not (0.34 particles per kg).17 In “hot spot” sites, concentrations can be extremely high with the soils outside an industrial site reporting concentrations as high as 67
500 ppm,18 and 55.5 ppm in the Swiss floodplains soils.19 Our understanding of the potential impacts of microplastics in soils is lacking, as studies in terrestrial organisms are scarce, representing only 10% of 45 peer-reviewed articles on microplastics in a recent review.20 Few terrestrial models have been investigated, with earthworms predominating, and nanoplastics have been largely ignored.20,21 Therefore, there is an urgent need to close this knowledge gap to gain a better understanding of the impacts of plastic pollution in terrestrial ecosystems.22
Drosophila is a diverse genus that is found in abundance worldwide in various environments, ranging from tropical to temperate climates.23 In terrestrial ecosystems, it plays important roles as predator, prey, pollinator, and decomposer.23–25Drosophila lives and breeds in many different decaying vegetal tissues, with a strong preference for rotting fruits,23 and thus environmental exposure is likely to occur. Fruits can contain plastics through the potential uptake of micro- and nanoplastics in plants,26 the aerial deposition of airborne microplastics on their surface,12 and, once fallen on the ground, from interactions with contaminated soils. Animals also interact with soil directly as larvae can roam into the soil as they seek a dry place to pupate. Within this group, Drosophila melanogaster, known as the fruit fly, is a well-studied and highly tractable genetic model for understanding the molecular mechanisms of embryogenesis, signaling pathways, and various human diseases.27 More recently, it has been growing in popularity as a model to assess the toxicity of nanomaterials28–31 and insect toxicology32 as it has many advantages including a short life-cycle (10–12 days), low cost, easy handling, a completely sequenced genome, and simpler homologues of the nervous33 and digestive systems.34 As one of first lines of defense during oral exposure, the digestive system will often suffer toxic effects from nanomaterials and Drosophila gut has proven a useful gut model for nanotoxicity.35 The first study using Drosophila as a model for microplastic toxicity was published earlier this year.36 In this study, flies were exposed to 200 μg mL−1 of 0.1 or 1 μm polystyrene particles for 7 days, alone or in combination with cadmium. Plastics alone caused no change in survival or epigenetics but decreased climbing ability and caused significant gut damage. Combined exposure with Cd aggravated the impact on these endpoints, suggesting additive or synergistic effects with metals like Cd. As only a single, high exposure concentration was tested, the effects of lower and more environmentally relevant concentrations, important chronic impacts on fly reproduction, as well as daily behaviors remain unknown.
The objective of this study was to assess the chronic effects of micro- and nanoplastics exposure in the fruit fly D. melanogaster by measuring various lethal and sublethal endpoints including mortality, development, locomotion, gene expression, daily activity rhythms, and fertility. This is one of the first studies to comprehensively assess the chronic dose-dependent impacts of both micro- and nanoplastics in the fruit fly. The toxicological data of this laboratory exposure experiment is a prerequisite for understanding and predicting the potential ecological impacts in terrestrial environments.
2. Materials & methods
2.1 Drosophila strains
The Oregon R strain of D. melanogaster was used for all experiments. Flies were cultured on Nutri-Fly Bloomington Formulation (Diamed) in a Panasonic MLR-352H-PA Versatile Environmental Test Chamber operating at 25 °C, 60% humidity and a 12/12 h day/night cycle. Egg collection and aging, and exposure experiments were also conducted under these conditions.
2.2 Polystyrene particles
Polystyrene particles have been found in soils.17–19 In addition to its environmental importance, the commercial availability of polystyrene particles in a wide range of sizes, surface functionalities, fluorescent labelling and stable concentrations, make it the most widely adopted model plastic particle in toxicological studies.37 To investigate the concentration-dependent effects, visualize the uptake in situ, avoid the use of surfactants, and compare to previous studies, carboxylated fluorescently labeled polystyrene particles in both micro- and nano size range were also chosen for this study. Commercially prepared 1 μm red fluorescently labeled carboxylated polystyrene (580 nm excitation, 605 nm emission, catalog N° F8821) and 20 nm green-yellow polystyrene particles (505 nm excitation, 515 nm emission, catalog N° F8787) were purchased from ThermoFisher. To remove the confounding toxic effects of preservatives, surfactants as well as any free dye within the stock suspensions, particles were dialyzed for 7 days according to a method described by Xu et al.38 This dialysis protocol was shown to remove excess fluorescent dye from these commercial particles, reducing the signal from leaked dye to negligible amounts.38 Even in harsh simulated gastric conditions, no detectable amount of fluorescent dye leached from similar polystyrene microspheres.39 It should be noted that the fluorescent dye was not quantified and only used to confirm particle uptake in the present study. Fluorescent imaging of particles in suspension and in Ward's Instant Drosophila Media shows that both particles generally maintained their original size and dispersion (Fig. S1 and S2†), except at high concentration (100 ppm) where some aggregates of nanoplastics formed when mixed into food media, with the largest aggregates being approximately 1 μm in diameter.
2.3 Developmental toxicity assay
2.3.1 Exposure conditions and workflow.
Flies were exposed to plastic particles as described in Rand et al.40 and the workflow is outlined in Fig. 1. Briefly, populations of ∼300–400 adult flies were housed in embryo collection cages on FlyStuff Grape Agar Premix (Diamed) with yeast paste (1 g per mL of Milli-Q water) to collect fresh eggs over an 8 h period. The eggs were aged for 24 h to 1st instar larvae stage and then exposed to a series of concentrations (0, 0.1, 1, 10, 50, 100 ppm) of micro- or nanoplastics and observed over 13 days. The 100 ppm concentration was equivalent to 2.27 × 1013 particles per mL of food and 1.8 × 108 particles per mL of food for the 20 nm and 1 μm polystyrene particles, respectively, and falls within the concentration range of plastics in “hot spot” sites. Particle suspensions were prepared by diluting a 5000 or 5 ppm stock of dialyzed particles in Milli-Q water to desired exposure concentration. Then, 10 mL of the particle suspension was added to 2.1 g of Ward's Instant Drosophila Medium (VWR) in a 25 mm wide glass tube. The pH of the medium ranged from 4.9–5.0, which is comparable to that of fruit fly's natural foods such as pears and bananas (pH 5.2).41 Fifty larvae were exposed in each vial, and 9 replicate vials were prepared for each condition. Of these, three vials were exposed for 3 days at which point 10 larvae from each vial were used in a larval crawling assay to assess locomotion, another 10 were observed by fluorescence microscopy (Olympus SZX16 stereomicroscope; settings in ESI†) to verify particle uptake, and 15 were placed on clean food for a 24 h depuration, being fluorescently imaged at 1, 4, 8, 12, and 24 h. The remaining vials were exposed for 13 days, with the number of pupae, adults, and mortality recorded daily, and the length of pupal casing measured on day 6 (Fig. S3†). On the final day, adult locomotion was assessed with an adult climbing assay before flies were anesthetized with carbon dioxide, counted, and sorted by sex. Nine mating pairs from each condition were randomly selected for a fertility assay. Thirty adult males and 30 females were preserved in 70% ethanol for imaging and body measurements (thorax length, Fig. S3†). Three females from control and 100 ppm conditions were prepared for nano-computed tomography X-ray scans. Six groups of 5 individuals per condition were placed in RNAlater™ Stabilization Solution (Invitrogen) to preserve for gene expression analysis (details in ESI†). The remaining flies were cleared according to the ScaleS protocol developed by Hama et al.42 for 10 days to reduce autofluorescence prior to fluorescence imaging with a stereomicroscope.
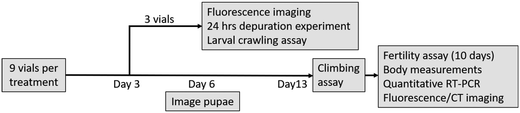 |
| Fig. 1 A workflow of the chronic toxicity assays. Each treatment had 9 vials with 50 individuals each. On day 3, 3 vials from each treatment were sacrificed to confirm particle uptake (by fluorescence imaging) and for depuration tests, as well as larval crawling assays. The remaining 6 vials were exposed until the emergence of adults (13 days). Their locomotion was assessed with climbing assay before being anesthetized and randomly sorted into fertility assay, euthanized for body measurements, preserved for quantitative real-time PCR expression analysis, or processed for fluorescence or X-ray CT imaging. | |
2.3.2 Larval crawling assay.
Ten randomly selected larvae were placed in a glass-bottom culture dish (14 mm diameter, Matsunami Glass, VWR) filled with 100 μL diluted yeast paste (0.1 g mL−1 of Milli-Q water). The light source from the microscope was below the glass-bottom culture dish. The animals were allowed to adapt to the lighting environment for 3 min before being recorded for 60 s and the number of peristaltic contractions per min noted. As Drosophila larvae demonstrate social behavior,43 locomotion was assessed individually and also in groups of ten.
2.3.3 Adult climbing assay.
The locomotor performance of Drosophila adults was measured according to the method described by Pappus et al.44 with minor modifications. Briefly, all emerged adult flies from each vial were transferred, without anesthesia, to a 100 mL glass graduated cylinder with a marking at 10 cm height and sealed in with a cotton plug. Flies were gently tapped down to the bottom of the vial and then allowed to ascend for 10 s. The proportion of flies that passed the 10 cm mark, as well as those that remained at the bottom of the vial, was recorded. This experiment was repeated three times for each vial and the mean proportion of flies that passed the 10 cm mark or remained at the bottom was calculated.
2.3.4 Fertility assay.
Nine mating pairs that had been previously exposed for 13 days to micro- or nanoplastics were placed in vials with food free of polystyrene particles for 10 days, being transferred to new vials every 48 h, and the number of progeny was recorded. Fertility was assessed on clean food to minimize the influence of oviposition preferences commonly observed in Drosophila, as females are seen to avoid laying eggs in unfavorable media.45
2.3.5 Gene expression.
Stress-response genes (HSP70, CAT, SOD2) and genotoxic stress response gene (P53) were selected. A detailed description of the RT-PCR method can be found in ESI.† Briefly, 15 males and 15 females from each condition were stored in groups of 5 in RNAlater (Thermo Fisher) at −4 °C, giving a total of 6 biological replicates per condition. Total RNA was isolated using Purelink RNA Mini Kit (Thermo Fisher); the amount of RNA in each sample was determined using a spectrophotometer and the quality was analyzed using agarose gel electrophoresis. First-strand cDNA was obtained using High-Capacity cDNA Reverse Transcription Kit (Thermo Fisher). Real-time quantitative PCR (RT-PCR) was performed in triplicate for each sample with a 7900HT Fast Real-Time PCR System (Applied Biosystems) following manufacturer's instructions and using SYBR Green-based detection of PCR products. Melting curves were examined after amplification to exclude the presence of unspecific products. Relative expression was calculated through the 2−ΔΔCt method and normalized to the transcript levels for alpha-tubulin.46
2.3.6 Nano-CT scan.
Nano-computed tomography (CT) scans using Zeiss Xradia 520 Versa (Carl Zeiss Canada Limited) were performed on three randomly selected female fruit flies from control and 100 ppm treatments of the two particles for qualitative assessment of the integrity of the intestinal walls. This technique offers the advantage of preserving soft tissues close to their original state and can distinguish differences in organ size and tissue organization. The flies were fixed in FAE fixative (6
:
10
:
1 ratio of 40% formaldehyde, ethanol, and glacial acetic acid) for 24 h, decapitated with a razor, and then stained in 1% phosphotungstic acid solution in water for 2 weeks, changing to fresh reagent every 2–4 days. Flies were then placed in solutions of increasing ethanol concentration (25, 50, 70%) for 20 min increments before being mounted in 70% ethanol in a 20–200 μL pipette tip. All the scans were acquired at 1–3 μm resolution with 4× objective lens with 2 × 2 camera binning over a 360 degree-rotation. A total of 2401 and 201–501 projections were taken in high and low resolution scans respectively, at 60 kVp and 82 μA. High resolution scans of each specimen were taken with LE1 filter with 2.7 s exposure, yielding 3 h scans in total whereas one of the 100 ppm with 20 nm plastic specimens was taken with LE2 filter with 3.5 s exposure, resulting in 3.5 h scan. In low resolution scans, the same filters were used with 0.8–1.2 s exposure, yielding 20–40 min scans. One control was scanned at low resolution with an LE2 filter and with additional projections (total 2401) yielding a 1.5 h scan time. The resulting images were qualitatively analyzed and 3D models were reconstructed with Dragonfly image analysis software (Object Research Systems Inc.). 3D models were generated based on a combination of the low and high resolution scan data.
2.4 Measuring daily activity and circadian rhythms
The daily activity and circadian rhythm of adult flies were assessed using a DAM2 Drosophila Activity Monitor (TriKinetics) following a protocol previously described by Chiu et al.47 with some modifications. Two-day old adult males were loaded individually into glass tubes with food containing 0, 10, 50, and 100 ppm microplastics or nanoplastics (16 flies per treatment). Monitors were placed in an incubator at 25 °C, 60% humidity for 8 days. For the first 4 days, flies were subjected to 12 h/12 h light/dark cycle (LD) and then a 24 h dark cycle (DD) for the remaining 4 days. An activity event was defined as a break in the infrared beam that crosses the center of the activity tube and the number of events was recorded over 1 min intervals. Data were analyzed using Shiny-R software48 to extract daily activity accounts, sleep events, bout lengths, and circadian rhythms. The first day of both cycles was a period of acclimatization and excluded from the final statistical analysis along with data from dead flies. Further details can be found in ESI.†
2.5 Statistical analyses
All statistical analyses were performed using R studio Software V3.3.149 with agricolae and MASS packages.50 Homoscedasticity was checked using Bartlett test and normality was checked on ANOVA residuals, with log-transformation when normality assumption was not met with raw data. Group comparisons were performed using one or two-way ANOVAs to assess the effect of concentration and, in the case of fertility, time. Considering the large difference in particle numbers of micro- and nano-sized particles at an equal mass concentration,51 no comparison was made between particle sizes. Statistical significances were obtained using TukeyHSD test. Statistical significance was set to p < 0.05. Data in bar graphs and box plots are presented as mean ± standard error (SE) and mean ± standard deviation (SD), respectively.
3. Results and discussion
3.1 Developmental exposure assay
3.1.1 Uptake and effects of micro- and nanoplastics in the gut.
After 3 days of exposure to 1 μm or 20 nm particles, 3rd instar larvae were rinsed with de-ionized water and imaged with a fluorescence stereomicroscope. The images in Fig. 2A show the accumulation of both types of particles in the gastrointestinal tract (GIT) of larvae. In contrast, only microplastics accumulated to levels that exceeded the background fluorescence in the GIT of adults in Fig. 2B. Nanoplastic fluorescence was likely masked by the green background autofluorescence of adult bodies that could not be completely eliminated by the clearing protocol. Although the extent of fluorescence was not quantitatively analyzed, it can be noted that the intensity of the fluorescence signal generally increased with exposure concentration (Fig. S4†).
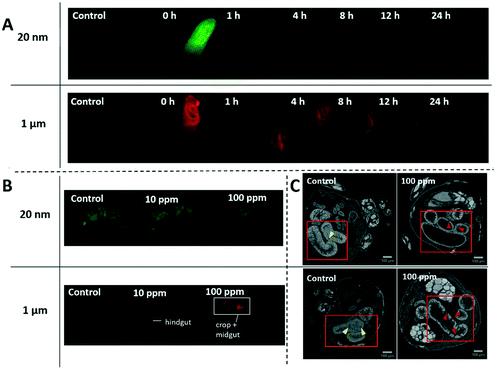 |
| Fig. 2
D. melanogaster larvae and adults exposed to various concentrations of 20 nm green-yellow or 1 μm red fluorescent polystyrene in food. A) Larvae were exposed to 100 ppm concentration for 3 days and then underwent a 24 h depuration period. Nano- and microplastics mainly localize in the gastrointestinal tract of the larvae. Nanoplastics were largely excreted from the gastrointestinal tract after 1 h while it took the full 24 h for the microplastics to clear. B) Adults that emerged after 13 days of exposure were cleared according to the ScaleS protocol42 for 10 days to reduce autofluorescence prior to fluorescence imaging with stereomicroscope. There is an accumulation of microplastics in the gastrointestinal tract that increases with exposure concentration. In contrast, there is no observable accumulation of nanoplastics. C) CT scans of gastrointestinal tracts are indicated in red squares. Normal secretory enteroendocrine cells are indicated by yellow arrows and signs of deterioration such as deep crypts or missing epithelia, are indicated by red arrows. See ESI† for larger images and 3D reconstructions. | |
Sequestration of micro- and nanoplastics in the GIT has been reported in many species including zebrafish,52 daphnia,53 fathead minnow,53 and krill54 as animals intake free-floating particles in the environment with their food. On the other hand, depuration is more varied depending on species and particle size. In general, microplastic depuration occurs relatively fast, usually from hours to days. Daphnia and fathead minnows cleared 6 μm polystyrene spheres 72–96 h after exposure to 2 × 10−3 ppm,53 and krill exposed to 20
000 ppm of 27–32 μm polyethylene spheres cleared them in 24 h.54 Nanoplastics tend to require longer depuration periods and a small portion often remains in the body. In scallops, 88% of ingested 24 nm polystyrene nanoparticles were excreted from the intestine after 3 days of depuration, but particles that had migrated to gills, hepatopancreas, gonad, and kidney remained.55 In the 24 h depuration period of larvae in our experiment we observed the opposite trend for depuration times, with 1 μm microparticles requiring 24 h to clear compared to 1 h for 20 nm nanoparticles. After the nanoplastic depuration, there appeared to be some residual green fluorescence throughout the body of larvae suggesting some migration to other tissues as was seen in previous studies. More sensitive techniques such as fluorescence spectrophotometry of homogenized tissue56 or histological analysis would be necessary to confirm this. The difference likely stems from the dispersion of particles in solid instead of liquid media, as well as the relative size of the particles to the animal gut. Microplastics are more comparable to the diameter of the gut (∼160 μm),57 which makes them more likely to form intestinal obstructions. Obstructions could lead to greater accumulation of microplastics, which in turn would require longer depuration times as we observed. Quantification of microplastics and nanoplastics within the GIT would be needed to confirm this hypothesis.
Intestinal blockages can also cause histopathological damages in the intestinal walls with such damage occurring from large (250–1000 μm),58 intermediate (15 μm),59 and nano-sized (100 nm)36 polystyrene spheres in different species including Drosophila. Although the extent of the damage in the latter study was quantified using dye, it was not visualized, leaving the nature of the tissue injury unknown. Here, a qualitative assessment of CT scans of female adults (Fig. 2C) indicated tissue disorganization and damage after exposure to 100 ppm of either particle size. Within the gut tube, the treatment flies appear to have little or no intact peritrophic matrix. These flies also appeared to have fewer or shrunken secretory enteroendocrine cells compared to controls. The enterocytes of control flies tightly filled the intestinal walls, whereas in plastic-treated flies, they were generally thinner and separated from each other, forming crypts that extended from the lumen to the basement membrane or, for 1 μm treated flies, gaps in the epithelia. However, there was a burst in the epithelia in one of the 3 controls, which suggests that larger gaps could have occurred due to processing. The 3D models constructed from the data show gross anatomical differences in the GIT (Fig. 3). The midgut distal to the middle midgut (copper cell region) is lengthened in treatment flies leading to extra coils in the posterior midgut region. In controls, the anterior midgut anteroventrally covers the major loop of the posterior midgut for the exception of one replicate control (Fig. S12†). In all treatment individuals imaged, the hypertrophied posterior midgut extends anterior to the anterior midgut coil. Additionally, the descending portion of the hindgut leading to the ampulla is generally shorter in all treatment individuals. These length and coiling differences are expected to manifest during larval development and offer an insight into further research using larval life stages. In Drosophila, the gut is not only central to digestion and nutrient absorption, but has complex self-regulatory functions via neuronal and peptide signalling.60 Damage to enteroendocrine cells has been shown to disrupt intestinal homeostasis and shorten adult life spans.61 Immunohistochemistry in follow-up studies will be required to fully characterize these histological differences.
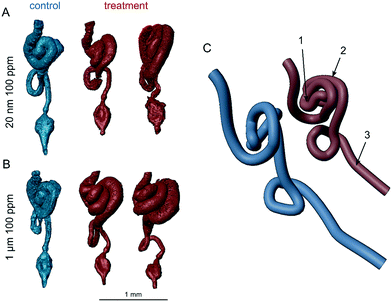 |
| Fig. 3 Reconstructed adult gastrointestinal tracts of D. melanogaster exposed to 100 ppm concentrations of A) 20 nm or B) 1 μm polystyrene particles. GITs are represented to the same scale from the anterior midgut to the ampulla and shown in ventral view with anterior toward the top. C) A right posteroventrolateral view of a simplified pipe representation of the GIT of the control and left treatment individual in (A). Labels: 1 – middle midgut (copper cell region), 2 – hypertrophied posterior midgut, and 3 – shortened descending hindgut. | |
3.1.2 No effect on development and mortality.
The number of pupae, adults, and dead adults in each vial was recorded daily, and body measurements (length of the pupal case and adult thorax length, Fig. S3†) were taken to evaluate the development of fruit flies (Table S2†). There was no significant delay in life-stage transitions or changes in pupae or thorax lengths in all treatments. While the previously discussed anatomical changes in the GIT are likely due to a development change, they are not considered here as it is unknown whether it is a primary developmental change that is irreversible, or a secondary change that is plastic and reversible. The eclosion rate, defined as the transition from pupae to adult fly, was also unaffected with rates of 89% or more in all treatments. The mortality of flies was less than 10% for all treatments, which is contrary to studies that observed increased mortality and/or decrease in body weight in other models.62,63 However, these studies used unwashed commercial particle suspensions that often contain toxic preservatives and surfactants like sodium azide.64 When these chemicals are removed, as they were via dialysis in our study, mortality is significantly reduced in plastic exposures.64 Similarly, low mortality for polystyrene spheres has been observed in Drosophila,36 mice,65 and different species of bacteria and algae66,67 at similar or greater exposure concentrations of comparable polystyrene particles. Therefore, the developmental toxicity of clean spherical polystyrene micro- and nanoparticles in fruit flies is low as they are quickly moved through the digestive tract with minimal migration to other tissues, and in consequence, cause little to no lethal damages within this 13 day time frame.
3.1.3 Gene expression.
The expression of stress-response genes (HSP70, CAT, SOD2) and genotoxic stress response gene (P53) of males and females was measured since reactive oxygen species (ROS) generation and/or genotoxicity have been observed with exposure to metallic68,69 and silica35 nanoparticles. In our experiment, there was no significant difference in the expression of CAT, SOD2, and P53 in all treatment groups (Fig. S5†). However, there was a significant upregulation of HSP70 in flies exposed to microplastics (F = 2.88, P = 0.033) with a 4.5-fold increase compared to controls (Fig. 4B). HSP70 is a highly conserved gene that is induced in Drosophila by various physical, physiological, and chemical stressors. Its upregulation has been observed in response to gold nanoparticles70 and cadmium,71 and is correlated with reduced lifespan.72 The upregulation of HSP70 might stem from potential dietary restriction, as the ingested plastics lack nutritional value and can also cause obstructions or physical damages. Given the longer depuration times observed for 1 μm spheres, the latter likely occurred to a greater extent for microplastics, hence consistently triggering the stress response and yielding a significant result.
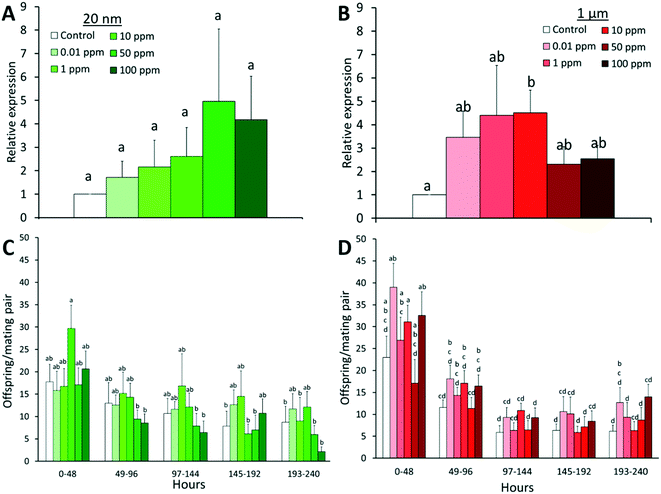 |
| Fig. 4 Average gene expression of HSP70 (A and B) and fertility (C and D) of D. melanogaster post nano- or microplastic exposure with standard error. After exposure, mating pairs of flies were transferred to clean food for 240 h (or 10 days), with flies being transferred to new vials every 48 h (n = 9). In cases where flies were lost during transfer, n was reduced in subsequent days. The relative abundance of HSP70 was determined from total RNA extracts of groups of 5 individuals per replicate (n = 6), analyzed in three technical replicates. Tubulin served as the reference gene. Letters correspond to significant differences according to one or two-way ANOVA with time and/or concentration as factors. Combinations (time × concentration) with the same letter are not considered significantly different by Tukey-HSD test. | |
3.1.4 Effects on fertility.
Fertility was measured by placing mating pairs on clean food for 10 days and counting the number of offspring (Fig. 4C and D). Although any specific treatment group was not significantly different from the control within the same time frame, in general, there was a greater number of offspring in 1 μm treated groups than controls at all time points (F = 4.75, P < 0.001). However, this increase appears to have no dependence on concentration which, in addition to the high variability in offspring produced, suggests that these results fall within a normal range. Time was also a significant factor in offspring numbers in both particle exposures (F = 8.44 and 41.12 for 20 nm and 1 μm exposures, respectively, P < 0.001), with fewer offspring being produced in each subsequent day. This can be attributed to the natural decline of fertility with age in Drosophila as there is both a decrease in stem cell division and an increase in cell death in developing eggs in older females.73 However, the rate of decline can vary from species to species, thus further studies on the aging and fertility of specific strains are required to distinguish the contribution of factors (i.e. aging, exposure) to the decline of fertility over time.73
3.1.5 Larval locomotion.
Larval locomotion was defined as the average number of peristaltic contractions per minute. Locomotor and behavioral measurements are highly sensitive endpoints to toxicants.74 The locomotor ability of flies was first assessed at the larval stage during the developmental toxicity assay. Nanoplastics showed no significant effect in individual tests, however, it impacted locomotion at concentrations ≥10 ppm when larvae were tested in a group (F = 14.89, P < 0.001) (Fig. 5B). D. melanogaster larvae demonstrate social behavior and tend to spontaneously form social foraging groups43 and can communicate via pheromone signaling.75,76 These social interactions affect individual behavior such as burrowing43 and localization.76 Therefore, it is likely that behavioral response is more sensitive to plastic exposure in a group; however, the potential mechanisms remain unknown. The non-monotonic response to nanoplastics we observed could result from competing effects from stress, nutrition and, potentially, neurotoxicity from translocated particles. Food scarcity can induce one of the two strategies depending on individual polymorphism: roving, when larvae actively forage for better feeding sites; or sitting, where energy is conserved by minimizing movement.77,78 Nanoplastics have been observed to be neurotoxic in fish79 and C. elegans62 and adsorb to sensorial antenna and appendages of shrimp80 and water fleas,38 all of which were associated with irregular motility in organisms. Depending on the nature of the damage, neurotoxicity can also alter behavior in either direction.81,82 At 100 ppm concentration of nanoplastics, factors that increase crawling overcame those that suppress the movement. In contrast, microplastics reduced the locomotion of larvae at concentrations ≥10 ppm (in groups: F = 15.6, P < 0.001) (Fig. 5B). Given the lack of microplastic fluorescence outside the GIT, neurotoxicity is unlikely the major cause. Instead, occlusions within the intestine could also impede movement, as the same peristaltic movements are responsible for locomotion of the larvae and transport of food through the digestive tract.83
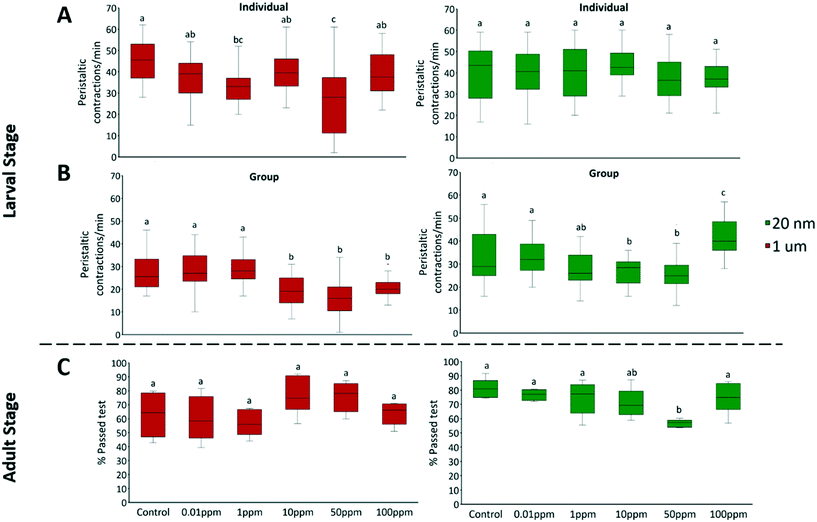 |
| Fig. 5 The locomotion of A) individual 3rd instar larvae, B) groups of ten 3rd instar larvae, C) adult D. melanogaster, was assessed via larval crawling and adult climbing assays after exposure to 20 nm green fluorescent or 1 μm red fluorescent polystyrene spheres for 3 days (larvae) or 13 days (adults). Bars represent standard deviation. Letters correspond to significant differences according to one-way ANOVA with concentration as the factor. Different letters indicate statistically significant differences by Tukey-HSD test; n = 10 in larval crawling assay; and n = 6 in adult climbing assay. | |
3.1.6 Adult locomotion.
The locomotion of adult flies was assessed with a climbing assay. Adult locomotion was defined as the proportion of adults that passed 10 cm height after a 10 s period. There was no difference in the proportion of flies that remained at the bottom in all conditions (Fig. S6†). Nanoplastics produced a similar but weaker response in adults as they did in larvae, while the effects of microplastics were absent in adults (Fig. 5C). The disappearance of movement inhibition from exposure to 1 μm plastics in adulthood could be due to the larger size of adult digestive tracts and the decoupling of whole-body movement and digestion. In contrast, 50 ppm of 20 nm polystyrene significantly inhibited the climbing activity (F = 4.49, P = 0.005). This contrasts with the results of Zhang et al.36 who saw similar climbing inhibition from 1 μm and 100 nm polystyrene particles. However, this was at 200 μg mL−1, twice the highest concentration in our study, which could explain the greater effect they observed. Like in larvae, the inhibited locomotion of adults could be attributed to the attachment of nanoplastics to mechanosensory organs such as bristles and/or neurotoxic effects. However, effects are dampened as any injury from particle exposure halts during the wandering phase of development, a period where larvae stop feeding and purge their gut prior to pupation. This may leave neurons a chance to regenerate and recover some locomotor ability.84
3.2 Daily activity and circadian rhythms
Adult male flies were exposed to 10, 50, and 100 ppm micro- and nanoplastics, and their daily activity was recorded. There were no significant effects on circadian rhythm, but daily activity counts significantly increased at 50 ppm of micro- (F = 5.11, P = 0.003) and nanoplastics (F = 4.13, P = 0.012) (Fig. 6A and C, respectively). Total sleep was unaffected by nanoplastic exposure but decreased with 50 ppm of microplastics (F = 9.84, P < 0.001) (Fig. 6B). The average sleep bout length was unaffected in all treatments (Fig. S7†), indicating that the number of sleep events was what diminished. The average activity bouts length increased at 50 ppm of micro- (F = 5.71, P = 0.002) and nanoplastics (F = 3.23, P = 0.029) (Fig. S7†). Overall, flies exposed to plastics were more active than controls, with higher activity counts, longer bouts of activity, and reduced total sleep at 50 ppm (Fig. 6A, B, and C). One possible reason is the effects of dietary restriction, that is defined as a decrease in nutrient intake without malnutrition, which has been shown to increase daily locomotor activity in flies.85
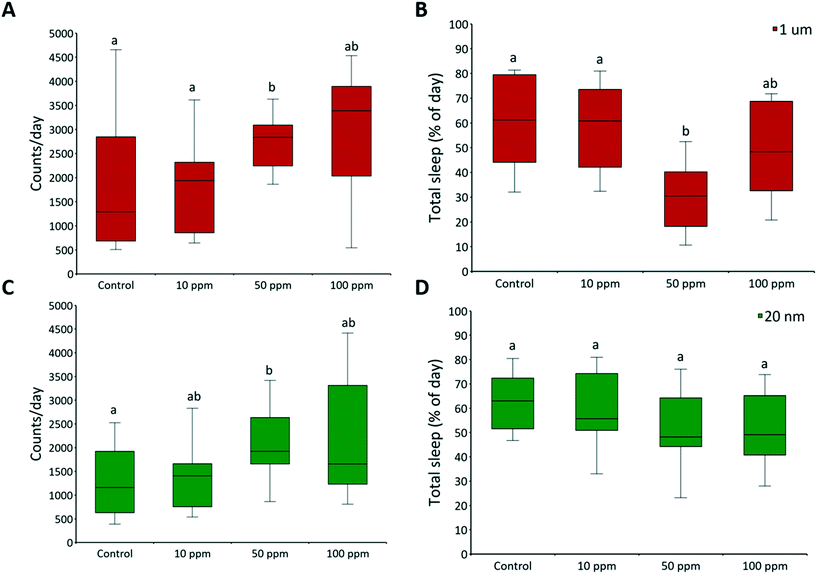 |
| Fig. 6 Daily activity and sleep. The daily activity of two-day-old adult male fruit flies was recorded by a Drosophila activity monitor 2 with activity counts being recorded in 1 min time intervals. The raw data were processed with Shiny-R software48 to extract individual (A and C) daily activity counts, and (B and D) total sleep per day (≥5 min of inactivity). Letters correspond to significant differences according to one-way ANOVA with concentration as a factor; n = 16 for each concentration. There is a range of sample size as flies that died during the experiment were excluded from the analysis. Mortality was ≤12% in all controls. | |
4. Conclusions
We provided the first comprehensive data on the chronic effects of micro- and nanoplastics on D. melanogaster following exposures to a wide range of plastic concentrations. Overall, our results showed that clean spherical model polystyrene particles caused little to no toxicity at environmentally relevant concentrations up to 100 ppm in the fruit fly. In the absence of other toxicants, these particles had limited effect on severe endpoints (i.e. mortality, development, and fertility), which suggests that the population of fruit flies would not be significantly impacted directly by exposure to up to 100 ppm of polystyrene micro- and nanospheres over one generation. However, there were several sub-lethal changes including upregulation of HSP70 expression, intestinal damage, and significant changes in locomotion and daily activity after chronic exposures. Together, these may reduce the lifespan of individuals in the actual environment. For example, the reduced locomotion of larvae and adults may increase the likelihood of predation. Microplastics also induced upregulation of HSP70 that is also associated with reduced lifespan,72 and the intestinal damages could reduce nutrient absorption. Our results motivate the need for follow-up mechanistic studies using naturally weathered plastic particles to understand effects on sublethal endpoints in intestinal and neuronal tissues using biochemical and histochemical assays, as well as investigating the impact on the whole life-cycle of the organism.
Conflicts of interest
There are no conflicts to declare.
Acknowledgements
The authors acknowledge the financial support of the Canada Research Chairs Program, the Canada Foundation for Innovation, the Natural Sciences and Engineering Research Council of Canada (NSERC) through its Discovery, Strategic Project, Canada Graduate Scholarships (CGS) and the Collaborative Research and Training Experience (CREATE) programs, the Canada Council for the Arts Killam Research Fellowship, le Regroupement des Écotoxicologues du Québec, the Eugenie Ulmer-Lamothe fund at McGill, and the SURE program at McGill University. The authors thank Paul Lasko at McGill University for providing D. melanogaster and Eric Déziel and Marie-Christine Groleau at INRS-Institut Armand-Frappier for advice on Drosophila rearing and handling. We also thank Brian Nguyen for his helpful comments. This research was performed using infrastructure of the Integrated Quantitative Biology Initiative, Canada Foundation for Innovation project 33122.
References
-
PlasticsEurope, Plastics - the Facts 2019: An analysis of European plastics productions, demand and waste data, PlasticsEurope, Belgium, Europe, 2019 Search PubMed.
- C. J. Rhodes, Plastic Pollution and Potential Solutions, Sci. Prog., 2018, 101, 207–260 CrossRef.
- M. Miernicki, T. Hofmann, I. Eisenberger, F. von der Kammer and A. Praetorius, Legal and practical challenges in classifying nanomaterials according to regulatory definitions, Nat. Nanotechnol., 2019, 14, 208–216 CrossRef CAS.
- S. Karbalaei, P. Hanachi, T. R. Walker and M. Cole, Occurrence, sources, human health impacts and mitigation of microplastic pollution, Environ. Sci. Pollut. Res., 2018, 25, 36046–36063 CrossRef CAS.
-
A. L. Andrady, Plastics and Environmental Sustainability, John Wiley & Sons, Inc, Hoboken, NJ, 2015 Search PubMed.
-
W. J. Shim, S. H. Hong and S. Eo, Marine Microplastics: Abundance, Distribution, and Composition, Elsevier Inc., 2018 Search PubMed.
- K. Mattsson, L.-A. Hansson and T. Cedervall, Nano-plastics in the aquatic environment, Environ. Sci.: Processes Impacts, 2015, 17, 1712–1721 RSC.
- O. S. Alimi, J. Farner Budarz, L. M. Hernandez and N. Tufenkji, Microplastics and Nanoplastics in Aquatic Environments: Aggregation, Deposition, and Enhanced Contaminant Transport, Environ. Sci. Technol., 2018, 52, 1704–1724 CrossRef CAS.
-
F. Wang, F. Wang and E. Y. Zeng, in Microplastic Contamination in Aquatic Environments: An emerging Matter of Environmental Urgency, Elsevier, 2018, pp. 225–247 Search PubMed.
- Y. Chae and Y. J. An, Effects of micro- and nanoplastics on aquatic ecosystems: Current research trends and perspectives, Mar. Pollut. Bull., 2017, 124, 624–632 CrossRef CAS.
-
L. Mai, L.-J. Bao, C. S. Wong and E. Y. Zeng, in Microplastic Contamination in Aquatic Environments, Elsevier, 2018, pp. 365–378 Search PubMed.
- J. Brahney, M. Hallerud, E. Heim, M. Hahnenberger and S. Sukumaran, Plastic rain in protected areas of the United States, Science, 2020, 368, 1257–1260 CrossRef CAS.
- E.-L. Ng, E. Huerta Lwanga, S. M. Eldridge, P. Johnston, H.-W. Hu, V. Geissen and D. Chen, An overview of microplastic and nanoplastic pollution in agroecosystems, Sci. Total Environ., 2018, 627, 1377–1388 CrossRef CAS.
- D. Briassoulis, M. Hiskakis, G. Scarascia, P. Picuno, C. Delgado and C. Dejean, Labeling scheme for agricultural plastic wastes in Europe: Labeling scheme for APW in Europe, Qual. Assur. Saf. Crops Foods, 2010, 2, 93–104 CrossRef.
- A. M. Mahon, B. O'Connell, M. G. Healy, I. O'Connor, R. Officer, R. Nash and L. Morrison, Microplastics in Sewage Sludge: Effects of Treatment, Environ. Sci. Technol., 2017, 51, 810–818 CrossRef CAS.
- G. S. Zhang and Y. F. Liu, The distribution of microplastics in soil aggregate fractions in southwestern China, Sci. Total Environ., 2018, 642, 12–20 CrossRef CAS.
- S. Piehl, A. Leibner, M. G. J. Löder, R. Dris, C. Bogner and C. Laforsch, Identification and quantification of macro- and microplastics on an agricultural farmland, Sci. Rep., 2018, 8, 17950 CrossRef CAS.
- S. Fuller and A. Gautam, A Procedure for Measuring Microplastics using Pressurized Fluid Extraction, Environ. Sci. Technol., 2016, 50, 5774–5780 CrossRef CAS.
- M. Scheurer and M. Bigalke, Microplastics in Swiss Floodplain Soils, Environ. Sci. Technol., 2018, 52, 3591–3598 CrossRef CAS.
- B. Nguyen, D. Claveau-Mallet, L. M. Hernandez, E. G. Xu, J. M. Farner and N. Tufenkji, Separation and Analysis of Microplastics and Nanoplastics in Complex Environmental Samples, Acc. Chem. Res., 2019, 52, 858–866 CrossRef CAS.
- Y. Chae and Y.-J. An, Current research trends on plastic pollution and ecological impacts on the soil ecosystem: A review, Environ. Pollut., 2018, 240, 387–395 CrossRef CAS.
- M. C. Rillig and A. Lehmann, Microplastic in terrestrial ecosystems, Science, 2020, 368, 1430–1431 CrossRef CAS.
- J. H. Skevington and P. T. Dang, Exploring the biodiversity of flies (Diptera), Biodiversity, 2002, 3(4), 3–27 CrossRef.
-
E. Galante and M. Marcos-Garcia, Encyclopedia of Entomology, ed. J. L. Capinera, 2008, pp. 1158–1169 Search PubMed.
- J. Frouz, Use of soil dwelling Diptera (Insecta, Diptera) as bioindicators: a review of ecological requirements and response to disturbance, Agric., Ecosyst. Environ., 1999, 74, 167–186 CrossRef.
- M. C. Rillig, Plastic and plants, Nat. Sustain., 2020, 3, 887–888 CrossRef.
- U. B. Pandey and C. D. Nichols, Human Disease Models in Drosophila melanogaster and the Role of the Fly in Therapeutic Drug Discovery, Pharmacol. Rev., 2011, 63, 411–436 CrossRef CAS.
- M. Alaraby, B. Annangi, R. Marcos and A. Hernández,
Drosophila melanogaster as a suitable in vivo model to determine potential side effects of nanomaterials: A review, J. Toxicol. Environ. Health, Part B, 2016, 19, 65–104 CAS.
-
N. Agrawal and P. Shah, Toxicology of nanoparticles: Insights from drosophila, Springer, Singapore, 2020 Search PubMed.
- A. S. Anand, U. Gahlot, D. N. Prasad and E. Kohli, Aluminum oxide nanoparticles mediated toxicity, loss of appendages in progeny of Drosophila melanogaster on chronic exposure, Nanotoxicology, 2019, 13, 977–989 CrossRef CAS.
- M. Alaraby, E. Demir, J. Domenech, A. Velázquez, A. Hernández and R. Marcos, In vivo evaluation of the toxic and genotoxic effects of exposure to cobalt nanoparticles using Drosophila melanogaster, Environ. Sci.: Nano, 2020, 7, 610–622 RSC.
- J. G. Scott and N. Buchon,
Drosophila melanogaster as a powerful tool for studying insect toxicology, Pestic. Biochem. Physiol., 2019, 161, 95–103 CrossRef CAS.
- L. McGurk, A. Berson and N. M. Bonini,
Drosophila as an In Vivo Model for Human Neurodegenerative Disease, Genetics, 2015, 201, 377–402 CrossRef CAS.
- B. Lemaitre and I. Miguel-Aliaga, The Digestive Tract of Drosophila melanogaster, Annu. Rev. Genet., 2013, 47, 377–404 CrossRef CAS.
- A. Pandey, S. Chandra, L. K. S. Chauhan, G. Narayan and D. K. Chowdhuri, Cellular internalization and stress response of ingested amorphous silica nanoparticles in the midgut of Drosophila melanogaster, Biochim. Biophys. Acta, Gen. Subj., 2013, 1830, 2256–2266 CrossRef CAS.
- Y. Zhang, M. B. Wolosker, Y. Zhao, H. Ren and B. Lemos, Exposure to microplastics cause gut damage, locomotor dysfunction, epigenetic silencing, and aggravate cadmium (Cd) toxicity in Drosophila, Sci. Total Environ., 2020, 744, 140979 CrossRef CAS.
- Y. Chae and Y.-J. An, Effects of micro- and nanoplastics on aquatic ecosystems: Current research trends and perspectives, Mar. Pollut. Bull., 2017, 124, 624–632 CrossRef CAS.
- E. G. Xu, R. S. Cheong, L. Liu, L. M. Hernandez, A. Azimzada, S. Bayen and N. Tufenkji, Primary and Secondary Plastic Particles Exhibit Limited Acute Toxicity but Chronic Effects on Daphnia magna, Environ. Sci. Technol., 2020, 54(11), 6859–6868 CrossRef CAS.
- A. P. Walczak, P. J. M. Hendriksen, R. A. Woutersen, M. van der Zande, A. K. Undas, R. Helsdingen, H. H. J. van den Berg, I. M. C. M. Rietjens and H. Bouwmeester, Bioavailability and biodistribution of differently charged polystyrene nanoparticles upon oral exposure in rats, J. Nanopart. Res., 2015, 17, 231 CrossRef.
-
M. D. Rand, S. L. Montgomery, L. Prince and D. Vorojeikina, in Current Protocols Toxicology, ed. L. G. Costa, J. C. Davila, D.
A. Lawrence and D. J. Reed, John Wiley & Sons, Inc., Hoboken, NJ, USA, 2014, pp. 1.12.1–1.12.20 Search PubMed.
- W. Seifriz, Breakdown of fruit and vegetables tissues due to an elecric current, Plant Physiol., 1936, 11, 195–200 CrossRef CAS.
- H. Hama, H. Hioki, K. Namiki, T. Hoshida, H. Kurokawa, F. Ishidate, T. Kaneko, T. Akagi, T. Saito, T. Saido and A. Miyawaki, ScaleS: an optical clearing palette for biological imaging, Nat. Neurosci., 2015, 18, 1518–1529 CrossRef CAS.
- Z. Durisko, R. Kemp, R. Mubasher and R. Dukas, Dynamics of Social Behavior in Fruit Fly Larvae, PLoS One, 2014, 9, e95495 CrossRef.
- S. A. Pappus, B. Ekka, S. Sahu, D. Sabat, P. Dash and M. Mishra, A toxicity assessment of hydroxyapatite nanoparticles on development and behaviour of Drosophila melanogaster, J. Nanopart. Res., 2017, 19, 136 CrossRef.
- B. Moreteau, S. R'Kha and J. R. David, Genetics of a nonoptimal behavior: Oviposition preference of Drosophila mauritiana for a toxic resource, Behav. Genet., 1994, 24, 433–441 CrossRef CAS.
- F. Ponton, M.-P. Chapuis, M. Pernice, G. A. Sword and S. J. Simpson, Evaluation of potential reference genes for reverse transcription-qPCR studies of physiological responses in Drosophila melanogaster, J. Insect Physiol., 2011, 57, 840–850 CrossRef CAS.
- J. C. Chiu, K. H. Low, D. H. Pike, E. Yildirim and I. Edery, Assaying Locomotor Activity to Study Circadian Rhythms and Sleep Parameters in Drosophila, J. Visualized Exp., 2010, 43, e2157 Search PubMed.
- K. Cichewicz and J. Hirsh, ShinyR-DAM: a program analyzing Drosophila activity, sleep and circadian rhythms, Commun. Biol., 2018, 1, 25 CrossRef.
- R Core Team, R: A language and environment for statistical computing, R Foundation for Statistical Computing, Vienna, Austria, 2020 Search PubMed.
-
W. Venables and B. Ripley, Modern Applied Statistics with S, Springer, New York, NY, 4th edn, 2002 Search PubMed.
- G. OberdÃrster, Safety assessment for nanotechnology and nanomedicine: concepts of nanotoxicology: Symposium: Nanotoxicological concepts, J. Intern. Med., 2010, 267, 89–105 CrossRef.
- Y. Lu, Y. Zhang, Y. Deng, W. Jiang, Y. Zhao, J. Geng, L. Ding and H. Ren, Uptake and Accumulation of Polystyrene Microplastics in Zebrafish (Danio rerio) and Toxic Effects in Liver, Environ. Sci. Technol., 2016, 50, 4054–4060 CrossRef CAS.
- A. Elizalde-Velázquez, A. M. Carcano, J. Crago, M. J. Green, S. A. Shah and J. E. Cañas-Carrell, Translocation, trophic transfer, accumulation and depuration of polystyrene microplastics in Daphnia magna and Pimephales promelas, Environ. Pollut., 2020, 259, 113937 CrossRef.
- A. Dawson, W. Huston, S. Kawaguchi, C. King, R. Cropp, S. Wild, P. Eisenmann, K. Townsend and S. Bengtson Nash, Uptake and Depuration Kinetics Influence Microplastic Bioaccumulation and Toxicity in Antarctic Krill (Euphausia superba), Environ. Sci. Technol., 2018, 52, 3195–3201 CrossRef CAS.
- M. Al-Sid-Cheikh, S. J. Rowland, K. Stevenson, C. Rouleau, T. B. Henry and R. C. Thompson, Uptake, Whole-Body Distribution, and Depuration of Nanoplastics by the Scallop Pecten maximus at Environmentally Realistic Concentrations, Environ. Sci. Technol., 2018, 52, 14480–14486 CrossRef CAS.
- Y. Deng, Y. Zhang, B. Lemos and H. Ren, Tissue accumulation of microplastics in mice and biomarker responses suggest widespread health risks of exposure, Sci. Rep., 2017, 7, 46687 CrossRef.
- N. Buchon, D. Osman, F. P. A. David, H. Yu Fang, J.-P. Boquete, B. Deplancke and B. Lemaitre, Morphological and Molecular Characterization of Adult Midgut Compartmentalization in Drosophila, Cell Rep., 2013, 3, 1725–1738 CrossRef CAS.
- A. Rodriguez-Seijo, J. Lourenço, T. A. P. Rocha-Santos, J. da Costa, A. C. Duarte, H. Vala and R. Pereira, Histopathological and molecular effects of microplastics in Eisenia andrei Bouché, Environ. Pollut., 2017, 220, 495–503 CrossRef CAS.
- R. Qiao, Y. Deng, S. Zhang, M. B. Wolosker, Q. Zhu, H. Ren and Y. Zhang, Accumulation of different shapes of microplastics initiates intestinal injury and gut microbiota dysbiosis in the gut of zebrafish, Chemosphere, 2019, 236, 124334 CrossRef CAS.
- I. Miguel-Aliaga, H. Jasper and B. Lemaitre, Anatomy and Physiology of the Digestive Tract of Drosophila melanogaster, Genetics, 2018, 210, 357–396 CrossRef CAS.
- A. Amcheslavsky, W. Song, Q. Li, Y. Nie, I. Bragatto, D. Ferrandon, N. Perrimon and Y. T. Ip, Enteroendocrine Cells Support Intestinal Stem-Cell-Mediated Homeostasis in Drosophila, Cell Rep., 2014, 9, 32–39 CrossRef CAS.
- L. Lei, M. Liu, Y. Song, S. Lu, J. Hu, C. Cao, B. Xie, H. Shi and D. He, Polystyrene (nano)microplastics cause size-dependent neurotoxicity, oxidative damage and other adverse effects in Caenorhabditis elegans, Environ. Sci.: Nano, 2018, 5, 2009–2020 RSC.
- B.-K. Zhu, Y.-M. Fang, D. Zhu, P. Christie, X. Ke and Y.-G. Zhu, Exposure to nanoplastics disturbs the gut microbiome in the soil oligochaete Enchytraeus crypticus, Environ. Pollut., 2018, 239, 408–415 CrossRef CAS.
- O. Pikuda, E. G. Xu, D. Berk and N. Tufenkji, Toxicity Assessments of Micro- and Nanoplastics Can Be Confounded by Preservatives in Commercial Formulations, Environ. Sci. Technol. Lett., 2019, 6, 21–25 CrossRef CAS.
- M. Rafiee, L. Dargahi, A. Eslami, E. Beirami, M. Jahangiri-rad, S. Sabour and F. Amereh, Neurobehavioral assessment of rats exposed to pristine polystyrene nanoplastics upon oral exposure, Chemosphere, 2018, 193, 745–753 CrossRef CAS.
- M. Heinlaan, K. Kasemets, V. Aruoja, I. Blinova, O. Bondarenko, A. Lukjanova, A. Khosrovyan, I. Kurvet, M. Pullerits, M. Sihtmäe, G. Vasiliev, H. Vija and A. Kahru, Hazard evaluation of polystyrene nanoplastic with nine bioassays did not show particle-specific acute toxicity, Sci. Total Environ., 2020, 707, 136073 CrossRef CAS.
- M. Okshevsky, E. Gautier, J. M. Farner, L. Schreiber and N. Tufenkji, Biofilm formation by marine bacteria is impacted by concentration and surface functionalization of polystyrene nanoparticles in a species-specific manner, Environ. Microbiol. Rep., 2020, 12, 203–213 CrossRef CAS.
- G. Vecchio, A. Galeone, V. Brunetti, G. Maiorano, L. Rizzello, S. Sabella, R. Cingolani and P. P. Pompa, Mutagenic effects of gold nanoparticles induce aberrant phenotypes in Drosophila melanogaster, Nanomedicine, 2012, 8, 1–7 CrossRef CAS.
- M. Ahamed, R. Posgai, T. J. Gorey, M. Nielsen, S. M. Hussain and J. J. Rowe, Silver nanoparticles induced heat shock protein 70, oxidative stress and apoptosis in Drosophila melanogaster, Toxicol. Appl. Pharmacol., 2010, 242, 263–269 CrossRef CAS.
- G. Vecchio, A. Galeone, V. Brunetti, G. Maiorano, S. Sabella, R. Cingolani and P. P. Pompa, Concentration-Dependent, Size-Independent Toxicity of Citrate Capped AuNPs in Drosophila melanogaster, PLoS One, 2012, 7, e29980 CrossRef CAS.
- X. Hu, W. Fu, X. Yang, Y. Mu, W. Gu and M. Zhang, Effects of cadmium on fecundity and defence ability of Drosophila melanogaster, Ecotoxicol. Environ. Saf., 2019, 171, 871–877 CrossRef CAS.
- J. Yang and J. Tower, Expression of hsp22 and hsp70 Transgenes Is Partially Predictive of Drosophila Survival Under Normal and Stress Conditions, J. Gerontol., Ser. A, 2009, 64, 828–838 CrossRef.
- P. B. Miller, O. T. Obrik-Uloho, M. H. Phan, C. L. Medrano, J. S. Renier, J. L. Thayer, G. Wiessner and M. C. Bloch Qazi, The song of the old mother: Reproductive senescence in female drosophila, Fly, 2014, 8, 127–139 CrossRef.
- T. D. Algarve, C. E. Assmann, T. Aigaki and I. B. M. da Cruz, Parental and preimaginal exposure to methylmercury disrupts locomotor activity and circadian rhythm of adult Drosophila melanogaster, Drug Chem. Toxicol., 2020, 43, 255–265 CrossRef CAS.
- J. D. Mast, C. M. De Moraes, H. T. Alborn, L. D. Lavis and D. L. Stern, Evolved differences in larval social behavior mediated by novel pheromones, eLife, 2014, 3, e04205 CrossRef.
- Z. Durisko and R. Dukas, Attraction to and learning from social cues in fruitfly larvae, Proc. R. Soc. B, 2013, 280, 20131398 CrossRef.
- M. B. Sokolowski, H. S. Pereira and K. Hughes, Evolution of foraging behavior in Drosophila by density-dependent selection, Proc. Natl. Acad. Sci. U. S. A., 1997, 94, 7373–7377 CrossRef CAS.
- R. K. Vijendravarma, S. Narasimha and T. J. Kawecki, Evolution of foraging behaviour in response to chronic malnutrition in Drosophila melanogaster, Proc. R. Soc. B, 2012, 279, 3540–3546 CrossRef CAS.
- K. Mattsson, E. V. Johnson, A. Malmendal, S. Linse, L.-A. Hansson and T. Cedervall, Brain damage and behavioural disorders in fish induced by plastic nanoparticles delivered through the food chain, Sci. Rep., 2017, 7, 11452 CrossRef.
- E. Bergami, E. Bocci, M. L. Vannuccini, M. Monopoli, A. Salvati, K. A. Dawson and I. Corsi, Nano-sized polystyrene affects feeding, behavior and physiology of brine shrimp Artemia franciscana larvae, Ecotoxicol. Environ. Saf., 2016, 123, 18–25 CrossRef CAS.
- S. D. Mhatre, V. Satyasi, M. Killen, B. E. Paddock, R. D. Moir, A. Saunders and D. Marenda, Synaptic abnormalities in a Drosophila model of Alzheimer's disease, Dis. Models Mech., 2014, 7, 373–385 CrossRef.
- A. B. Pizzo, C. S. Karam, Y. Zhang, H. Yano, R. J. Freyberg, D. S. Karam, Z. Freyberg, A. Yamamoto, B. D. McCabe and J. A. Javitch, The membrane raft protein Flotillin-1 is essential in dopamine neurons for amphetamine-induced behavior in Drosophila, Mol. Psychiatry, 2013, 18, 824–833 CrossRef CAS.
- D. R. LaJeunesse, B. Johnson, J. S. Presnell, K. Catignas and G. Zapotoczny, Peristalsis in the junction region of the Drosophila larval midgut is modulated by DH31 expressing enteroendocrine cells, BMC Physiol., 2010, 10, 14 CrossRef.
- L. DeVault, T. Li, S. Izabel, K. L. Thompson-Peer, L. Y. Jan and Y. N. Jan, Dendrite regeneration of adult Drosophila sensory neurons diminishes with aging and is inhibited by epidermal-derived matrix metalloproteinase 2, Genes Dev., 2018, 32, 402–414 CrossRef CAS.
- S. Ghimire and M. S. Kim, Enhanced Locomotor Activity Is Required to Exert Dietary Restriction-Dependent Increase of Stress Resistance in Drosophila, Oxid. Med. Cell. Longevity, 2015, 2015, 1–8 CrossRef.
Footnote |
† Electronic supplementary information (ESI) available: Includes a pdf document with additional details on materials & methods, additional data, and full size CT images. See DOI:10.1039/d0en00942c |
|
This journal is © The Royal Society of Chemistry 2021 |