Insights into the factors influencing mercury concentrations in tropical reservoir sediments†
Received
19th April 2021
, Accepted 17th August 2021
First published on 8th September 2021
Abstract
Thousands of dams are currently under construction or planned worldwide to meet the growing need for electricity. The creation of reservoirs could, however, lead to conditions that promote the accumulation of mercury (Hg) in surface sediments and the subsequent production of methylmercury (MeHg). Once produced, MeHg can bioaccumulate to harmful levels in organisms. It is unclear to what extent variations in physical features and biogeochemical factors of the reservoir impact Hg accumulation. The objective of this study was to identify key drivers of the accumulation of total Hg (THg) in tropical reservoir sediments. The concentration of THg in all analyzed depth intervals of 22 sediment cores from the five contrasting reservoirs investigated ranged from 16 to 310 ng g−1 (n = 212, in the different sediment cores, the maximum depth varied from 18 to 96 cm). Our study suggests reservoir size to be an important parameter determining the concentration of THg accumulating in tropical reservoir sediments, with THg ranging up to 50 ng g−1 in reservoirs with an area exceeding 400 km2 and from 100 to 200 ng g−1 in reservoirs with an area less than 80 km2. In addition to the reservoir size, the role of land use, nutrient loading, biome and sediment properties (e.g., organic carbon content) was tested as potential drivers of THg levels. The principal component analysis conducted suggested THg to be related to the properties of the watershed (high degree of forest cover and low degree of agricultural land use), size and age of the reservoir, water residence time and the levels of nutrients in the reservoir. A direct correlation between THg and tested variables was, however, only observed with the area of the reservoir.
Environmental significance
The creation of hydroelectric reservoirs is well known to alter the biogeochemical cycle of mercury (Hg) and to potentially enhance the accumulation of Hg within the reservoirs. Although many of the currently constructed and planned reservoirs are located in South America, few studies have focused on the accumulation of Hg in tropical reservoirs. In this study we show the size of the reservoir to be a critical driver of the concentrations of total Hg in tropical reservoir sediments. In addition to the reservoir size, our study suggests that factors such as the nutrients levels in the reservoirs and the degree of forest cover around the reservoir could play a role in the levels of total Hg accumulating in the sediments.
|
1. Introduction
Hydroelectricity is the oldest renewable energy source and today accounts for around 80% of the renewable energy produced worldwide.1–3 With the global need for energy growing, extensive hydroelectricity expansion is currently underway.4 Construction of reservoirs and dams is, however, associated with substantial environmental costs, such as biodiversity loss,5,6 fish migration,5 increased emissions of greenhouse gases,7,8 and the potential for increased contaminant exposure, including exposure to mercury (Hg).9–11
The accumulation of Hg in aquatic systems poses a threat to biota and to human health, particularly when it is transformed to methylmercury (MeHg), a highly toxic form of Hg that bioaccumulates and biomagnifies in aquatic food webs.12,13 Several studies have demonstrated elevated concentrations of Hg in fish from constructed reservoirs in comparison to the concentrations found in unperturbed rivers and natural lakes.14 The effects observed, however, vary greatly between systems and with time. In recently impounded reservoirs, flooding of the landscape typically results in a greater flux of Hg and organic matter (OM) to the reservoir water column.15,16 In combination with decreased flow rates, increased inputs of OM lead to a greater accumulation of material, including OM and Hg, in reservoir sediments. Increased deposition of OM tends to result in oxygen depletion towards bottom waters and conditions favorable for the microbial methylation of Hg to MeHg.14,17,18 During the operation of the reservoir, water level fluctuations may continue to promote Hg methylation due to changing redox conditions.16,18,19 Such redox shifts result in, e.g., the oxidation of sulfur, which enhances the activity of sulfate-reducing bacteria, including Hg methylators.20,21
Although many of the planned and newly constructed reservoirs are located in Brazil and other parts of South America,4 there is a lack of studies on the accumulation of Hg in tropical reservoirs. Thus, the cycling of Hg and reservoir features explaining differences in the accumulation between systems are poorly understood. Here, we have studied sediment cores from five Brazilian reservoirs with contrasting features in order to identify and discuss potential key drivers for the THg levels in tropical reservoir surface sediments. Using generated data from these five reservoirs and previously published data from other tropical reservoirs, THg in the surface sediments of the different reservoirs was then tested as a function of physical reservoir features (e.g., reservoir size, trophic status and catchment land use and biome) and ancillary parameters, such as: total organic carbon content (TOC), total nitrogen (TN), the TOC/TN ratio and the δ13C and δ15N isotopic signatures.
2. Material and methods
2.1 Study area and sample collection
In total, 22 sediment cores were collected from the five reservoirs, Curuá-Una (CUN, n = 4), Monte Serrat, Bonfante and Santa Fé (MBS, n = 3), Chapéu D'Uvas (CDU, n = 5), Furnas (FNS, n = 3) and Funil (FUN, n = 7; Fig. 1 and Table S1†). The MBS reservoirs consist of a cascade system with three dams located in the Paraibuna river.22 The physical and hydrological features of the reservoirs are presented in Table 1. Details of the sampling have been described elsewhere.8,22 Briefly, the sediment cores were collected during the dry season in 2015 (July, September and October) and 2017 (July and October) at accumulation bottoms in transects (for CUN, CDU, FNS and FUN) from the inflow of the main river to the dam. Sediment cores were obtained using a gravity corer equipped with a hammer (6 cm internal diameter, UWITEC, Mondsee, Austria) and then sliced in intervals of three or six cm (information of the depth intervals used in this study are presented in Table S1†).23 The resulting samples (n = 212) were dried at 40 °C, homogenized using a mortar and pestle, sieved through 72 μm, and placed in airtight plastic bags until analysis. This drying procedure has previously been shown to not alter the THg concentrations, but to be problematic for the determination of MeHg.24
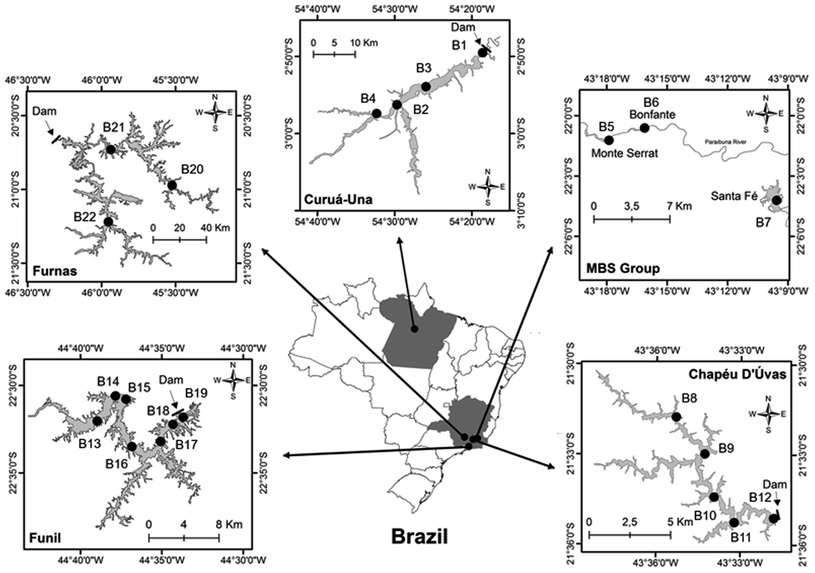 |
| Fig. 1 Location of the reservoirs Curuá-Una (CUN), Monte Serrat, Bonfante and Santa Fé (MBS), Chapéu D'Uvas (CDU), Furnas (FNS), and Funil (FUN). The gray areas indicate the state of Pará (north Brazil), and the state of Minas de Gerais and Rio de Janeiro (southeast Brazil). The cores were labelled as B and numbered from 1 to 22 in each reservoir. | |
Table 1 Description of the reservoirs including their location, physical and hydrological features (area, water residence time, maximum depth and annual precipitation), reservoir age and use, nutrient status (trophic state) and information of the surrounding area (type of surrounding, biome of the region and anthropogenic influences)
Features |
CDU22,25,26 |
CUN8,22,27 |
FUN22,28,29 |
FNS30–32 |
MBS22,33 |
Location (state in Brazil) |
Minas Gerais |
Pará |
Rio de Janeiro |
Minas Gerais |
Minas Gerais bordering Rio de Janeiro |
Surrounding area |
Urban |
Forest |
Urban |
Urban |
Urban |
Main river |
Paraibuna |
Curuá Una |
Paraíba do Sul |
Grande |
Paraibuna |
Year of damming |
1994 |
1977 |
1969 |
1963 |
2008 and 2009 |
Reservoir age (time between damming and sampling) |
21 |
40 |
46 |
52 |
8 and 7 |
Mean area (km2) |
12 |
72 |
35 |
1342 |
0.3, 0.2 and 2 |
Water residence time (days) |
716 |
30 |
20 |
511 |
Unknown |
Maximum depth (m) |
41 |
19.5 |
60 |
127 |
15, 10 and 25 |
Annual precipitation (mm per year) |
1443 |
1750 |
1337 |
1126 |
1600 |
Biome |
Atlantic forest |
Amazon |
Atlantic forest |
Savanna |
Atlantic forest |
Trophic state |
Oligotrophic |
Oligotrophic |
Eutrophic |
Mesotrophic, and eutrophic |
Mesotrophic |
Activities and anthropogenic influence |
Domestic effluents, deforestation |
Artisanal gold mining, vegetation burning, other mining, deforestation |
Industrial, agriculture and domestic effluents, deforestation, fish farming |
Agricultural (coffee, potatoes, corn), sewage discharge, fish farming |
Domestic, industrial effluents (textile, tannery) |
Reservoir use(s) |
Water supply |
Electricity |
Electricity, aquaculture, water supply |
Electricity, irrigation, industry supply |
Electricity |
2.2 Sample analysis
2.2.1 Determination of THg concentration.
The concentration of THg in sediments was analyzed through thermal decomposition followed by pre-concentration of Hg on a gold trap and cold vapor and Hg detection by atomic absorption spectrophotometry (CV-AAS) using a Milestone Direct Mercury Analyzer (DMA-80).34 The concentration of THg was determined for all the sediment depth intervals collected (n = 212, Table S1†), as well as for a subset of samples where the fine-grained fractions (<72 μm) were extracted (n = 43). For the quality control, an in-house sediment reference material with a previously determined THg concentration of 210 ± 10 ng g−1 was used. The observed mean and standard deviation (SD) concentration of this in-house reference sediment material was 213 ± 8.2 ng g−1 (relative standard deviation (RSD) of 3.8%, relative error of 1.6%, n = 21). In a separate study, in which the THg concentration of the in-house reference sediment material measured was 212 ± 6.4 ng g−1, the accuracy of the analysis was also validated with a commercially available reference sediment material (NIST2702, measured THg: 432 ± 10 ng g−1, certified THg: 447.4 ± 6.9 ng g−1). Randomly selected samples (n = 12) were analyzed in triplicate (RSD ranged from 1.2 to 3.7%). Blanks were measured (n = 30) and limit of detection (3 × SD) was calculated to 0.2 ng. In comparison, the amount of THg detected in samples ranged from 1.1 to 36 ng.
2.2.2 Ancillary parameters.
Surface sediment samples (<12 cm) were analyzed for TOC, TN and for their δ13C and δ15N isotopic signatures (Table S2†). In addition, TOC/TN ratios were calculated. TOC and TN content and their isotopic composition (δ13C and δ15N) were analyzed at the UC Davis Stable Isotope Facility using an elemental analyzer interfaced to a continuous flow isotope ratio mass spectrometer. Approximately 10 to 15 mg of prepared sample material were weighed in silver capsules, then 50 μl Milli-Q water and 100 μl of HCl 12 M were added. The samples were left overnight in a desiccator to remove the carbonates and then dried in an oven at 60 °C before being sent out for analysis. In addition, loss on ignition (LOI) was carried out on sediments from the CUN reservoir to assess the organic matter content in the deeper sediment layers by combusting the samples at 550 °C for 4 h.35
2.3 Statistical analysis
All statistical analyses and plots were done using JMP Pro 15 and Origin 2020, respectively. The distribution of the data was tested using the Anders–Darling test (with normal distribution rejected when p < 0.05). When testing the entire dataset of THg (n = 212) normal distribution was rejected. Depth-weighted concentration of HgT, TOC:TN ratio, δ13C and δ15N (n = 22) was deemed to be normally distributed while TOC and TN were deemed to have a lognormal distribution. One-way ANOVA tests were carried out to test for statistically significant differences of HgT, TOC:TN ratio, δ13C, δ15N, TOC and TN between the five reservoirs (using depth-weighted data and log transformed depth-weighted data for TOC and TN). Pairwise post-hoc tests were then done using the Tukey–Kramer HSD test. All further statistical data analyses were conducted using non-parametric methods (no log transformation of the data was done for these tests). Correlation between variables was tested using the Spearman ranked correlation (ρ) test (Tables S2 and S4†). All reported concentrations of THg and the ancillary parameters are presented as median ± IQR (interquartile range).
A principle component analysis (PCA) was performed by combining the chemical and physical data of the five reservoirs in this study (Table 1) with those of the five reservoirs (Tucuruí, Samuel, Manso, Itaipú, and Ribeirão das Lajes, Table S5†) included in the review by Pestana et al.36 to identify drivers between reservoirs and variables and to explore their correlations and influence on THg concentrations. The number of variables included in the analysis was limited to 9 (n − 1, n being the number of reservoirs). Mean concentrations of THg and content of TOC were calculated in each reservoir. The trophic state (nutrient levels) was converted to a trophic state index variable ranging from 1 to 5, where 1 is considered to be oligotrophic, 3 is mesotrophic and 5 is eutrophic. Visual inspection of satellite images was performed to identify major land use patterns around each reservoir. From this inspection, the density of agriculture and forest was assessed and scored from 0 (minor land use), 0.5 (ca. 50% of the land use) to 1 (dominant land use). Tropical status and the density of agriculture and forest were treated as continuous variables in the PCA. The PCA was performed using data from 10 reservoirs and the following 9 variables: THg, TOC, age and area of reservoir, maximum depth, trophic state index, water residence time, agriculture cover, and forest cover. A separate PCA was performed that also included biome as a variable (Fig. S4†). To avoid scaling effects, the data was standardized by subtracting the mean and dividing by the standard deviation for each value of each feature (Z-score). To maximize the loadings of the variables, a varimax rotation was applied, and the selection of the number of components was based on eigenvalues <1. Two-dimensional charts, i.e., biplots, were constructed (Fig. 4 and S4†) and the relationship between variables was evaluated. Correlation between variables in this data set was tested using the Spearman ranked correlation (ρ) test (Table S6†). To test the potential of THg concentration to overfit the model, another PCA was performed excluding THg concentrations.
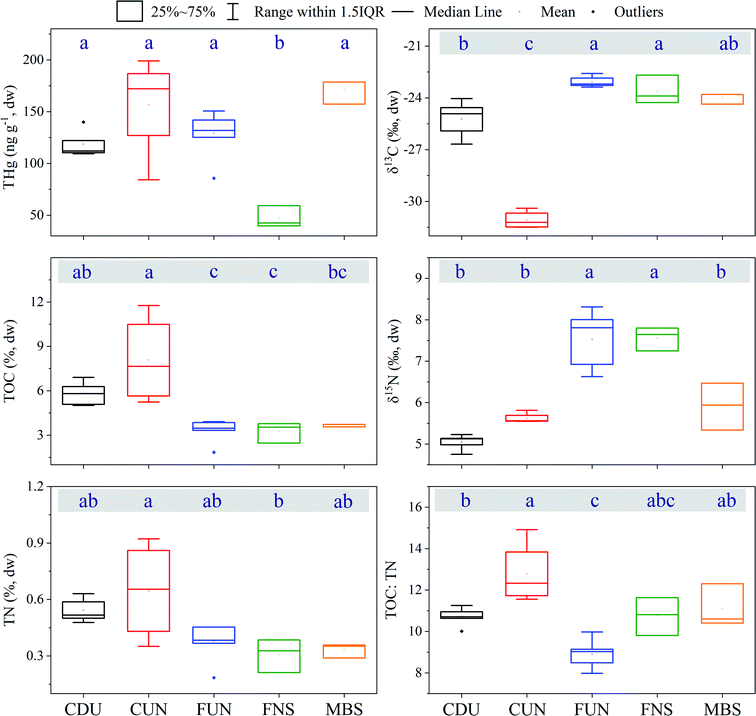 |
| Fig. 2 Boxplots of depth-weighted concentrations in surface sediments (<12 cm) of THg, content of total organic carbon (TOC) and total nitrogen (TN), isotopic compositions of δ15N and δ13C, and TOC:TN ratios in the reservoirs Curuá-Una (CUN), Monte Serrat, Bonfante and Santa Fé (MBS), Chapéu D'Uvas (CDU), Furnas (FNS), and Funil (FUN). All concentrations or isotopic compositions are presented as per dry weight (dw) of the sediments. Letters represent the results of pairwise comparisons using Tukey–Kramer HSD and indicate significant differences in concentrations and content among the reservoirs. Reservoirs with different letters are significantly different at the 0.05 significance threshold. | |
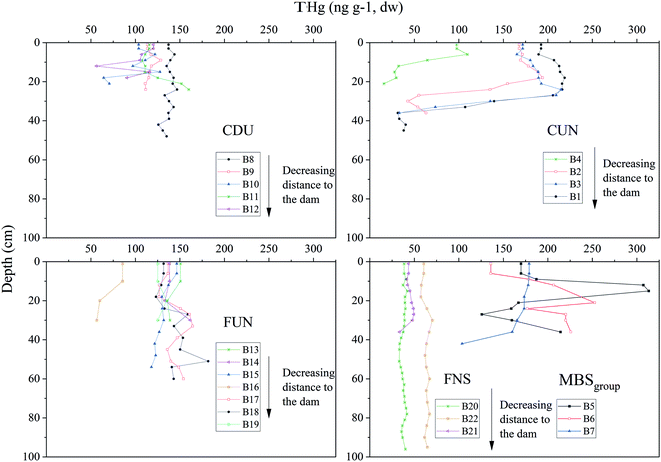 |
| Fig. 3 Vertical profiles of THg concentrations (dry weight, dw) in sediment cores from the reservoirs: Curuá-Una (CUN), Monte Serrat, Bonfante and Santa Fé (MBS), Chapéu D'Uvas (CDU), Furnas (FNS), and Funil (FUN). | |
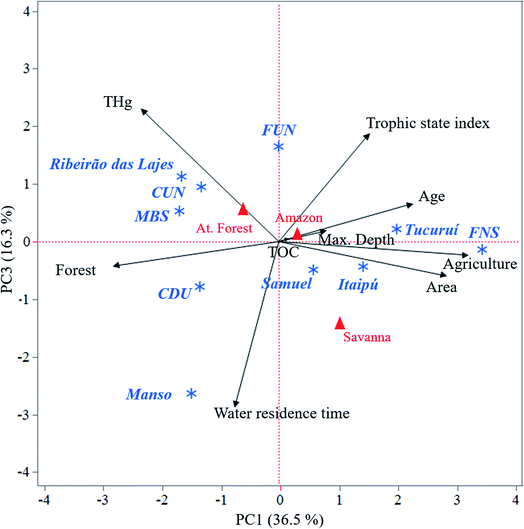 |
| Fig. 4 Principal component biplot showing PC scores of the reservoirs (blue asterisks), type of biome (red triangles), and loading of the variables (black arrows) for component 1 (PC1) and component 3 (PC3). Max. depth: maximum depth; age: reservoir age; area: reservoir area; agriculture: cover of agriculture as land use; forest: cover of forest as land use; TOC: total organic carbon; water residence time; THg; At. forest: Atlantic forest biome; Amazon: Amazon biome; Savanna: Savanna biome; reservoirs: FNS – Furnas, FUN – Funil, CUN – Curuá Uná, CDU – Chapeú D'Uvas, and MBS – Monte Serrat, Bonfante, and Santa Fé. Information from the reservoirs Tucuruí, Itaipú, Samuel, Manso and Ribeirão das Lajes were collected from Pestana et al.36 and other earlier studies (Table S5†). | |
3. Results
3.1 Ancillary parameters
The content of TOC and TN in the analyzed surface sediments ranged from 1.84 to 11.8% (4.9 ± 3.1%, n = 49), and from 0.18 to 0.92% (0.45 ± 0.23%, n = 49), respectively (Tables S2 and S3† and Fig. 2). When comparing depth-weighted TOC and TN, differences were observed between the reservoirs (TOC: CUN > FUN, FNS and MBS, CDU > FUN and FNS, TN: CUN > FUN). Calculated TOC/TN ratios ranged from 8.0 to 15.1 (11 ± 1.6 n = 49). The isotopic composition of δ13C and δ15N in the analyzed depth sections ranged from −31.5 to −22.6‰ and from 4.8 to 8.3‰, respectively, with a more depleted signature of δ13C found in CUN (−31.2 ± 0.8‰, n = 4, for CUN, in comparison to −23.2 ± 0.4‰ to −24.9 ± 1.3‰ in the other reservoirs). For the δ15N signature, more enriched signals were found in FUN (7.8 ± 1.1‰, n = 7) and FNS (7.6 ± 0.5‰, n = 3) in comparison to the other three reservoirs (CDU: 5.1 ± 0.2‰, n = 5, CUN: 5.6 ± 0.1‰, n = 4, MBS: 5.9 ± 1.1‰, n = 3). There are significant correlations among the proxies δ13C, δ15N, TOC, TN and the TOC/TN ratios (Table S4†).
3.2 Distribution of THg in reservoir sediments
The concentrations of THg in sediments (Fig. 2) ranged from 40 to 199 ng g−1 (126 ± 44, n = 22) in the upper 12 cm of the sediment cores, and from 16 to 310 ng g−1 (130 ± 96 ng g−1, n = 212) in all analyzed depth intervals (in the different sediment cores, the maximum depth varied from 18 to 96 cm). In most of the cores, the concentration of THg remained fairly constant with depth (relative percentage range of <50%) compared to the variability observed between cores (relative percentage range of 120%, Fig. 3 and S1†). In some of the cores (from MBS and CDU), however, concentrations of THg peaked at sediment depths of 10 to 18 cm. For the cores collected at CUN, lower concentrations of THg were also observed in the deeper layers of the cores (>24 cm). For the top 12 cm, lower concentrations of THg were observed in FNS compared to the other reservoirs (Fig. 2). For a subset of the samples, where the fine-grained (<72 μm) fraction of the sediments was also analyzed, a close to 1
:
1 relationship was observed between the THg concentrations in bulk and the fine-grained fraction (R2 = 0.97, n = 43, Fig. S2†). The concentration of THg increased with increasing concentrations of TOC and TN (including the top 12 cm of the sediment, Table S4†). There was no clear trend (i.e. change in concentration) when visually inspecting the depth-weighted concentration of THg (for the upper 20 cm of the sediments) within each reservoir as a function of the distance from the constructed dams (Fig. S3†).
3.3. Grouping according to PCA
The PCA resulted in three significant components (eigenvalues > 1) accounting for 76.3% of the total variance of the data set. The first component (PC1, physical features, Fig. 4) explained 36.5% of the variance and was positively influenced by the degree of agricultural cover and reservoir area and age and negatively influenced by THg and degree of forest cover. The second component (PC2) explained 23.5% of the variance and was positively influenced by maximum water depth and water residence time, negatively influenced by TOC but was not influenced by THg (Fig. S4†). The third component (PC3, biological productivity, Fig. 4) explained 16.3% of the variance, and was positively influenced by THg and negatively influenced by water residence time and trophic index. When excluding THg concentrations in the PCA, the number of significant components, the variance explained, and the variables accounting for each component did not change. Furthermore, the eigenvalues were comparable when running the PCA with and without THg. These results show that THg can be considered an independent variable.
4. Discussion
4.1 THg concentrations
The concentrations of THg observed in the five reservoirs are in the same range as the THg concentrations previously reported from tropic reservoirs36 and other reservoirs worldwide.14 The lack of information on the local THg background levels from these environments hinders us in evaluating whether or not these sediments' THg concentrations are influenced by local point sources. Based on the observed concentration ranges, however, we assume these THg pools to, first and foremost, reflect THg originating from natural and secondary anthropogenic sources rather than local anthropogenic point sources such as small-scale gold mining. This assumption is supported by the fact that the observed THg ranges in the reservoirs are orders of magnitude lower than many of the observations made elsewhere (THg concentrations ranging up to 10
000–100
000 ng g−1) at sites known to be heavily impacted by mining activities.14 Furthermore, no clear THg hotspots were observed within the reservoirs when exploring the spatial distribution of THg as a function of the distance from constructed dams. We cannot, however, rule out the fact that local point sources may still have contributed to observed THg concentrations. Mining activities have, for instance, been reported from the Tapajós National Forest, where the CUN reservoir is located.37–40
Bulk concentrations of TOC have been shown in many systems to explain the variability of THg concentrations observed in sediments.41 In our study, we also observed a positive correlation between TOC and THg in the surface sediments among the collected cores (top 12 cm, n = 49, Spearman ranked correlation test: ρ = 0.306, p-value = 0.033, Table S4†). TOC was, however, not a variable that was related to THg in the PCA analysis of the extended dataset (PCA analysis including mean THg and TOC from 10 reservoirs).
The sediment analyzed here is assumed to consist of material accumulated after the reservoirs were constructed. This assumes that the visually observed transition between the mineral and the organic-rich layer in each core also represents the transition between material accumulated before and after the flooding, respectively.22 At CUN, lower concentrations of THg were found in the lower parts of the cores (Fig. 3), likely due to lower OM content in these sections as indicated by the lower LOI550 values observed (Table S7†). If these more minerogenic layers represent material accumulated before the reservoir impoundment, these results would also suggest a greater accumulation of THg in the sediment as a result of the flooding. Higher concentrations of THg in sediment layers accumulated after flooding have also been shown previously in, e.g., the Urrá reservoir, Sinú River, Colombia.42,43
4.2 Factors influencing THg concentrations
Several processes, such as dilution and reduction of THg exported to the reservoirs, may alter the final concentration of THg in reservoir surface sediments. These processes may, in turn, be influenced by various reservoir features such as watershed land use, reservoir age and surface area. Here, we evaluated reservoir size, trophic state index and catchment land use and biome as potential drivers of THg in the surface sediments of 10 tropical reservoirs (data from this study and data compiled by Pestana et al.36).
Terrestrial runoff is the primary source of THg in most freshwater systems.14 The loading of THg to downstream systems (and reservoirs) may, however, depend on the properties of the catchment. Foliar uptake is recognized to be of importance for the flux of Hg from the atmosphere to the watershed, as well as for the export of Hg to downstream systems.44,45 In our PCA analysis, the degree of forest cover surrounding the reservoirs was also related to THg for PC1 (Fig. 4). Furthermore, the amounts of Hg exported to downstream systems may depend on the type of vegetation present, as vegetation type may lead to large differences in the amount of THg accumulated, retained in, and export from, the watershed.46,47 The reservoirs included in this study are located in regions with three different biomes: Savanna, Atlantic forest and Amazon. The amount of THg exported from the watershed is typically coupled with the amount of OM exported.13,48 Regions with the savanna biome are less productive and have less OM-rich soils49 compared to the Amazon and Atlantic forest biomes.50 These regions are thus less likely to accumulate high amounts of Hg and also less likely to export high amounts of Hg to downstream systems. From the PCA that also included biomes (Fig. S4†), however, none of the three components suggested that the type of biome was related to THg. Furthermore, the PCA did not suggest that TOC accumulating in the sediments was related to THg. These results suggest that features other than the biome control THg in the reservoir surface sediments. It should however be noted that the biome only describes the vegetation in the region, and not the actual coverage of forests around the reservoir investigated.
In the PCA, PC1 was positively influenced by the degree of agricultural cover and negatively influenced by the THg. Similarity, THg positively influenced PC3 while trophic state index (with a higher index for eutrophic systems) negatively influenced the same PC. These observations suggest low THg to be related with a high degree of agricultural land use and more eutrophic conditions within the reservoir. Earlier studies have suggested that catchments with substantial agricultural cover result in lower concentrations of THg in the runoff compared to catchments dominated by urban and forested areas.51–53 The excess of nutrients from areas with intensive agricultural land use can also result in lower THg concentrations in downstream systems due to the increased production of biomass within the aquatic system54,55 and the subsequent dilution of Hg.56,57 Among the reservoirs included in this analysis, no correlation was found between the agricultural land use and the nutrient status in the reservoirs (p = 0.575, Table S6†). The agricultural land use, however, correlated positively with the reservoir area (ρ = 0.675, p = 0.032). The fact that THg correlated with the area of the reservoir (ρ = −0.721, p = 0.019), suggests that the results of the PCA that low THg was related with a high degree of agricultural land use could be a spurious relationship, rather than to demonstrate a true causality.
For the set of reservoirs sampled in this study, we analyzed ancillary parameters related to land use and reservoir productivity (TOC/TN ratios and δ15N). The TOC/TN ratios observed in all reservoirs, except for FUN, were greater than 10 and suggest significant contributions of allochthonous organic carbon in most of the surface sediments.58 The variation in TOC/TN ratios is strongly linked to plant–microbial–soil OM transformations (litter decomposition and build-up of soil OM),59 and to the contribution of different OM pools (from vegetation, leaf litter, roots, and soil microbes). In the same way as these OM pools differ in TOC and TN content, THg concentrations vary among different environmental compartments.60–62 In the studied reservoirs, however, lower TOC/TN ratios were not consistent with lower THg concentrations (Fig. 2), suggesting either TOC/TN ratios do not reflect differences in the dilution of terrestrially derived material between sites, that dilution of the terrestrial material does not play a major role, or that differences in TOC/TN ratios do not reflect differences in THg loading from different OM sources.
In comparison to CDU, MBS and CUN, the catchments of FUN and FNS (where low THg concentrations were observed) have a high percentage of agricultural land cover (Table 1).8,31,33,63 For both reservoir systems, enriched δ15N signals were observed, indicating these sediments are influenced by the input of agricultural and/or wastewater runoff.64,65 As mentioned above, high agricultural land use can result in lower Hg loading to downstream systems and dilution of the Hg within the reservoir due to eutrophication. Lower concentrations of THg were, however, only observed in FNS and not in FUN. As can be seen in the Fig. 4, these two sites were also not close to each other in the PC1–PC3 biplot. As discussed further below, other features of the reservoirs were likely more important in controlling the accumulation of Hg that the agricultural land use. It would however, still be of interest to further explore the correlation between these proxies (δ15N and TOC/TN) and THg in future studies as the PCA indicated that nutrient loadings could play a role in THg concentrations in the reservoir sediments.
The age of the reservoir was another variable influencing THg concentrations in sediments for PC1. A correlation between the reservoir age and THg in sediments was also suggested by Pestana et al.36 and was observed in a study comparing reservoirs of different ages in China.66 It should, however, be noted that our data fit poorly with the modelled relationship between sediment THg and reservoir age put forth in Pestana et al.36 Furthermore, Khopkar suggests catchment features (e.g., hydrology, land use, organic matter input) to be more important for THg in surface sediments than the reservoir age.67 We also note that most studies examining the short- and long-term effects of the Hg biogeochemical cycle in reservoirs focus on the timing of enhanced methylation of Hg68,69 or peaks of Hg concentrations in fish (which is driven by Hg methylation and the subsequent bioaccumulation and biomagnification of MeHg).70,71 The same processes are, however, unlikely to drive the concentration of THg in surface sediments. Age-related shifts in THg concentrations in surface sediments are thus not necessarily expected.
Among the five reservoirs investigated in this study, we observed higher concentrations of THg in the four smaller reservoirs in comparison to the larger FNS reservoir. This observation is in line with the meta-analysis from Pestana et al.36 which included data from four smaller and one larger reservoir (Fig. 5 and Table S5†). The relationship between THg concentration and the area of the reservoir is also represented in PC1 (Fig. 4), which is, in addition, influenced by land use (represented here as agriculture/forest cover) and reservoir age. Together, the data currently available from reservoirs in South America (this study and the data synthesized by Pestana et al.36) suggest sediment concentrations of THg of up to 50 ng g−1 in reservoirs with an area exceeding 400 km2 and concentrations ranging from 100 to 200 ng g−1 in reservoirs with an area less than 80 km2 (Fig. 5). There are several processes that may contribute to lower THg concentrations in the sediments of the larger reservoirs. First of all, the distribution of terrestrial material over greater areas in the largest reservoirs can result in a higher fraction of the THg derived from the terrestrial compartment being reduced through, e.g., photochemical pathways72 and subsequently evading out of the aquatic system as elemental Hg (Hg0). Secondly, the distribution of THg over larger areas can result in a greater dilution of the terrestrial material with autochthonous carbon. Given the similar direction of both size and agricultural landcover in the biplot, we cannot separate the contribution of the two. A significant direct correlation was, however, only observed between THg and reservoir size (Table S6†) and not between THg and agricultural cover, suggesting reservoir size to the be primary reservoir feature influencing THg in surface sediments.
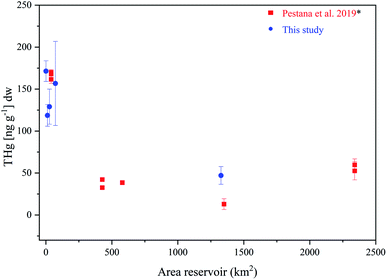 |
| Fig. 5 Relationship between THg concentration (ng per g dry weight) and the reservoir area of tropic reservoirs in surface sediments in this study (blue circle) and from other reservoirs in South America (red square), n = 9 from *Pestana et al.36 | |
Conclusions
Given the extensive hydroelectricity expansion currently underway in South America,4 understanding drivers of Hg accumulation across systems is needed in order to mitigate the risks of Hg upon the construction of the reservoirs. Here, we explored the potential role of physical reservoir features (e.g., reservoir size, nutrient levels, and catchment land use and biome) and ancillary parameters, such as: TOC, TN, TOC/TN, and the δ13C and δ15N isotopic signatures for the accumulated concentrations of THg in tropical reservoirs. Out of the tested variables, the size of the reservoirs was the feature that best predicted THg concentrations, with greater concentrations of THg found in the smaller reservoirs (area < 100 km2). Although the concentrations of Hg accumulating in the reservoir sediments not necessarily reflects the amounts of Hg accumulating in the fish,73 these results indicate higher concentrations of Hg to accumulate in the abiotic compartment of the smaller reservoirs. The risks associated with the Hg in these reservoirs are thus likely to be greater. We further note that higher concentrations of Hg also have been previously noted elsewhere in the biota from smaller freshwater systems in comparison to larger reservoirs.73
In addition to the size of the reservoir, the PCA suggested that the type of watershed (high degree of forest cover and low degree of agricultural land use), age of the reservoir, water residence time and the levels of nutrients in the reservoir may influence THg concentrations in surface sediments in tropical reservoirs. Although we in this study cannot tease the influence of these parameters apart, or eliminate the possibility that some of these relationships are spurious, the results presented points to potential drivers that warrants further investigation. Our data also shows that both in-reservoir and watershed drivers need to be considered. In addition to the forest coverage and other properties of the natural environment that influence the cycling of Hg, perturbations of the watershed may alter the capacity of the landscape to retain the Hg deposited.14 Forestry, for instance, increases both the re-emission of Hg back to the atmosphere as well as the export of Hg to downstream systems. Our study suggest that the smaller reservoirs may be more vulnerable for such perturbation as these systems already accumulate higher concentrations of Hg in the abiotic compartment.
Conflicts of interest
There are no conflicts to declare.
Acknowledgements
We are grateful for the study area map provided by José Reinaldo Paranaiba. This study was financed in part by the Coordenação de Aperfeiçoamento de Pessoal de Nível Superior—Brasil (CAPES)—Finance Code 001. The fieldwork was funded partially by the European Research Council under the European Union's Seventh Framework Programme (FP7/2007–2013)/ERC grant agreement n° 336642.
References
-
P. Breeze, in Power Generation Technologies, ed. P. Breeze, Newnes, Third edn, 2019, pp. 173–201 Search PubMed.
- M. A. P. Mahmud, N. Huda, S. H. Farjana and C. Lang, IOP Conf. Ser.: Mater. Sci. Eng., 2018, 351, 012–018 CrossRef.
-
W. Rex, V. Foster, K. Lyon, J. Bucknall and R. Liden, Supporting Hydropower: an Overview of the World Bank Group's Engagement, 2014 Search PubMed.
- C. Zarfl, A. E. Lumsdon, J. Berlekamp, L. Tydecks and K. Tockner, A global boom in hydropower dam construction, Aquat. Sci., 2015, 77, 161–170 CrossRef.
- M. Dorber, K. R. Mattson, O. T. Sandlund, R. May and F. Verones, Quantifying net water consumption of Norwegian hydropower reservoirs and related aquatic biodiversity impacts in Life Cycle Assessment, Environ. Impact Assess. Rev., 2019, 76, 36–46 CrossRef.
- C. J. R. Alho, Environmental effects of hydropower reservoirs on wild mammals and freshwater turtles in Amazonia: a review, Oecologia Aust., 2011, 15, 593–604 CrossRef.
- R. M. Almeida, J. R. Paranaíba, Í. Barbosa, S. Sobek, S. Kosten, A. Linkhorst, R. Mendonça, G. Quadra, F. Roland and N. Barros, Carbon dioxide emission from drawdown areas of a Brazilian reservoir is linked to surrounding land cover, Aquat. Sci., 2019, 81, 68 CrossRef.
- G. R. Quadra, S. Sobek, J. R. Paranaíba, A. Isidorova, F. Roland, R. do Vale and R. Mendonça, High organic carbon burial but high potential for methane ebullition in the sediments of an Amazonian hydroelectric reservoir, Biogeosciences, 2020, 17, 1495–1505 CrossRef CAS.
- V. Hatje, R. L. B. Andrade, R. M. Jesus, P. Masqué, A. C. R. Albergaria-Barbosa, J. B. de Andrade and A. C. S. S. Santos, Historical records of mercury deposition in dated sediment cores reveal the impacts of the legacy and present-day human activities in Todos os Santos Bay, Northeast Brazil, Mar. Pollut. Bull., 2019, 145, 396–406 CrossRef CAS PubMed.
- P. C. Van Metre and B. J. Mahler, Contaminant Trends in Reservoir Sediment Cores as Records of Influent Stream Quality, Environ. Sci. Technol., 2004, 38, 2978–2986 CrossRef CAS PubMed.
- L. Chassiot, P. Francus, A. De Coninck, P. Lajeunesse, D. Cloutier and T. Labarre, Spatial and temporal patterns of metallic pollution in Québec City, Canada: Sources and hazard assessment from reservoir sediment records, Sci. Total Environ., 2019, 673, 136–147 CrossRef CAS PubMed.
- J. Dolbec, D. Mergler, C.-J. Sousa Passos, S. Sousa de Morais and J. Lebel, Methylmercury exposure affects motor performance of a riverine population of the Tapajós river, Brazilian Amazon, Int. Arch. Occup. Environ. Health, 2000, 73, 195–203 CrossRef CAS PubMed.
-
W. F. Fitzgerald and C. H. Lamborg, in Treatise on Geochemistry, ed. H. D. Holland and K. K. Turekian, Pergamon, Oxford, 2007, pp. 1–47 Search PubMed.
- H. Hsu-Kim, C. S. Eckley, D. Achá, X. Feng, C. C. Gilmour, S. Jonsson and C. P. J. Mitchell, Challenges and opportunities for managing aquatic mercury pollution in altered landscapes, Ambio, 2018, 47, 141–169 CrossRef PubMed.
-
R. A. Bodaly, K. G. Beaty, L. H. Hendzel, A. R. Majewski, M. J. Paterson, K. R. Rolfhus, A. F. Penn, V. L. S. Louis, B. D. Hall and C. J. Matthews, Peer Reviewed: Experimenting with Hydroelectric Reservoirs, ACS Publications, 2004 Search PubMed.
- C. S. Eckley, T. P. Luxton, J. L. McKernan, J. Goetz and J. Goulet, Influence of reservoir water level fluctuations on sediment methylmercury concentrations downstream of the historical Black Butte mercury mine, OR, Appl. Geochem., 2015, 61, 284–293 CrossRef CAS.
- A. G. Bravo, S. Bouchet, J. Tolu, E. Björn, A. Mateos-Rivera and S. Bertilsson, Molecular composition of organic matter controls methylmercury formation in boreal lakes, Nat. Commun., 2017, 8, 1–9 CrossRef PubMed.
- S. M. Ullrich, T. W. Tanton and S. A. Abdrashitova, Mercury in the Aquatic Environment: A Review of Factors Affecting Methylation, Crit. Rev. Environ. Sci. Technol., 2001, 31, 241–293 CrossRef CAS.
-
M. Marcotte, R. Schetagne, N. Thérien, C. Langlois and A. Tremblay, Mercury in the Biogeochemical Cycle: Natural Environments and Hydroelectric Reservoirs of Northern Québec (Canada), Springer Science & Business Media, 2012 Search PubMed.
- C. S. Eckley, T. P. Luxton, J. Goetz and J. McKernan, Water-level fluctuations influence sediment porewater chemistry and methylmercury production in a flood-control reservoir, Environ. Pollut., 2017, 222, 32–41 CrossRef CAS PubMed.
- B. A. Poulin, J. N. Ryan, K. L. Nagy, A. Stubbins, T. Dittmar, W. Orem, D. P. Krabbenhoft and G. R. Aiken, Spatial Dependence of Reduced Sulfur in Everglades Dissolved Organic Matter Controlled by Sulfate Enrichment, Environ. Sci. Technol., 2017, 51, 3630–3639 CrossRef CAS PubMed.
- G. R. Quadra, A. Lino, A. Sobek, O. Malm, N. Barros, Y. Guida, J. Thomaz, R. Mendonça, S. Cardoso, C. Estrada, F. Rust and F. Roland, Environmental Risk of Metal Contamination in Sediments of Tropical Reservoirs, Bull. Environ. Contam. Toxicol., 2019, 103, 292–301 CrossRef CAS PubMed.
- R. Mendonça, S. Kosten, S. Sobek, J. J. Cole, A. C. Bastos, A. L. Albuquerque, S. J. Cardoso and F. Roland, Carbon Sequestration in a Large Hydroelectric Reservoir: An Integrative Seismic Approach, Ecosystems, 2014, 17, 430–441 CrossRef.
- H. Kodamatani, C. Maeda, S. J. Balogh, Y. H. Nollet, R. Kanzaki and T. Tomiyasu, The influence of sample drying and storage conditions on methylmercury determination in soils and sediments, Chemosphere, 2017, 173, 380–386 CrossRef CAS PubMed.
- J. R. Paranaíba, N. Barros, R. Mendonça, A. Linkhorst, A. Isidorova, F. Roland, R. M. Almeida and S. Sobek, Spatially Resolved Measurements of CO2 and CH4 Concentration and Gas-Exchange Velocity Highly Influence Carbon-Emission Estimates of Reservoirs, Environ. Sci. Technol., 2018, 52, 607–615 CrossRef PubMed.
-
M. A. de Olivera, Limnologia da Paisagem com uso de Regressao Geograficamente Ponderada: estudo da qualidade da água na represa de Chapeú DÚvas, MG (in Portuguese), Universidad Federal de Juiz de Fora, 2018 Search PubMed.
- P. M. Fearnside, Do Hydroelectric Dams Mitigate Global Warming? The Case of Brazil's CuruÁ-una Dam, Mitig. Adapt. Strategies Glob. Change, 2005, 10, 675–691 CrossRef.
- M. C. S. Soares, M. I. de A. Rocha, M. M. Marinho, S. M. F. O. Azevedo, C. W. C. Branco and V. L. M. Huszar, Changes in species composition during annual cyanobacterial dominance in a tropical reservoir: physical factors, nutrients and grazing effects, Aquat. Microb. Ecol., 2009, 57, 137–149 CrossRef.
- R. N. de S. Lima, C. B. de M. Ribeiro, C. C. F. Barbosa, O. C. Rotunno Filho, R. N. de S. Lima, C. B. de M. Ribeiro, C. C. F. Barbosa and O. C. Rotunno Filho, Estudo da poluição pontual e difusa na bacia de contribuição do reservatório da usina hidrelétrica de Funil utilizando modelagem espacialmente distribuída em Sistema de Informação Geográfica, Eng. Sanit. Ambiental, 2016, 21, 139–150 CrossRef.
- V. De Souza Dias, M. Pereira da Luz, G. M. Medero, D. Tarley Ferreira Nascimento, W. Nunes de Oliveira and L. Rodrigues de Oliveira Merelles, Historical Streamflow Series Analysis Applied to Furnas HPP Reservoir Watershed Using the SWAT Model, Water, 2018, 10, 458 CrossRef.
- R. C. Chaves, C. C. Figueredo, I. G. Boëchat, J. T. M. de Oliveira and B. Gücker, Fluorescence indices of dissolved organic matter as early warning signals of fish farming impacts in a large tropical reservoir, Ecol. Indic., 2020, 115, 106389 CrossRef CAS.
- R. M. Santos, N. F. Negreiros, L. C. Silva, O. Rocha and M. J. Santos-Wisniewski, Biomass and production of Cladocera in Furnas Reservoir, Minas Gerais, Brazil, Braz. J. Microbiol., 2010, 70, 879–887 CAS.
- M. C. S. Soares, V. L. M. Huszar and F. Roland, Phytoplankton dynamics in two tropical rivers with different degrees of human impact (southeast Brazil), River Res. Appl., 2007, 23, 698–714 CrossRef.
-
O. US EPA, EPA Method 7473 (SW-846), https://www.epa.gov/homeland-security-research/epa-method-7473-sw-846-mercury-solids-and-solutions-thermal-decomposition, accessed 3 April 2018.
- O. Heiri, A. F. Lotter and G. Lemcke, Loss on ignition as a method for estimating organic and carbonate content in sediments: reproducibility and comparability of results, J. Paleolimnol., 2001, 25, 101–110 CrossRef.
- I. A. Pestana, L. S. Azevedo, W. R. Bastos and C. M. Magalhães de Souza, The impact of hydroelectric dams on mercury dynamics in South America: a review, Chemosphere, 2019, 219, 546–556 CrossRef CAS PubMed.
- I. Aula, H. Braunschweiler and I. Malin, The watershed flux of mercury examined with indicators in the Tucuruí reservoir in Pará, Brazil, Sci. Total Environ., 1995, 175, 97–107 CrossRef CAS.
- P. J. Lechler, J. R. Miller, L. D. Lacerda, D. Vinson, J.-C. Bonzongo, W. B. Lyons and J. J. Warwick, Elevated mercury concentrations in soils, sediments, water, and fish of the Madeira River basin, Brazilian Amazon: a function of natural enrichments?, Sci. Total Environ., 2000, 260, 87–96 CrossRef CAS PubMed.
- M. Roulet, M. Lucotte, A. Saint-Aubin, S. Tran, I. Rhéault, N. Farella, E. De Jesus Da silva, J. Dezencourt, C.-J. Sousa Passos, G. Santos Soares, J.-R. D. Guimarães, D. Mergler and M. Amorim, The geochemistry of mercury in central Amazonian soils developed on the Alter-do-Chão formation of the lower Tapajós River Valley, Pará state, Brazil1The present investigation is part of an ongoing study, the CARUSO project (IDRC-UFPa-UQAM), initiated to determine the sources, fate, and health effects of MeHg in the Lower Tapajós area.1, Sci. Total Environ., 1998, 223, 1–24 CrossRef CAS PubMed.
- M. Roulet and M. Lucotte, Geochemistry of mercury in pristine and flooded ferralitic soils of a tropical rain forest in French Guiana, South America, Water, Air, Soil Pollut., 1995, 80, 1079–1088 CrossRef CAS.
- T. Stoichev, D. Amouroux, J. C. Wasserman, D. Point, A. De Diego, G. Bareille and O. F. X. Donard, Dynamics of mercury species in surface sediments of a macrotidal estuarine–coastal system (Adour River, Bay of Biscay), Estuarine, Coastal Shelf Sci., 2004, 59, 511–521 CrossRef CAS.
- J. J. Feria, J. L. Marrugo and H. González, Heavy metals in Sinú river, department of Córdoba, Colombia, South America, Rev. Fac. Ing., Univ. Antioquia, 2010, 35–44 CAS.
- J. Marrugo-Negrete, A. Navarro-Frómeta and J. Ruiz-Guzmán, Total mercury concentrations in fish from Urrá reservoir (Sinú river, Colombia). Six years of monitoring, Rev. MVZ Cordoba, 2015, 20, 4754–4765 CrossRef.
- G. S. da Silva, M. C. Bisinoti, P. S. Fadini, G. Magarelli, W. F. Jardim and A. H. Fostier, Major aspects of the mercury cycle in the Negro River Basin, Amazon, J. Braz. Chem. Soc., 2009, 20, 1127–1134 CrossRef.
- D. C. Teixeira, L. D. Lacerda and E. V. Silva-Filho, Mercury sequestration by rainforests: the influence of microclimate and different successional stages, Chemosphere, 2017, 168, 1186–1193 CrossRef CAS PubMed.
- J. Rydberg, J. Karlsson, R. Nyman, I. Wanhatalo, K. Näthe and R. Bindler, Importance of vegetation type for mercury sequestration in the northern Swedish mire, Rödmossamyran, Geochim. Cosmochim. Acta, 2010, 74, 7116–7126 CrossRef CAS.
- M. Schütze, G. Tserendorj, M. Pérez-Rodríguez, M. Rösch and H. Biester, Prediction of Holocene Mercury Accumulation Trends by Combining Palynological and Geochemical Records of Lake Sediments (Black Forest, Germany), Geosciences, 2018, 8, 358 CrossRef.
- C. L. Babiarz, J. M. Benoit, M. M. Shafer, A. W. Andren, J. P. Hurley and D. A. Webb, Seasonal influences on partitioning and transport of total and methylmercury in rivers from contrasting watersheds, Biogeochemistry, 1998, 41, 237–257 CrossRef CAS.
- X. Chen, L. B. Hutley and D. Eamus, Soil organic carbon content at a range of north Australian tropical savannas with contrasting site histories, Plant Soil, 2005, 268, 161–171 CrossRef CAS.
- T. Dittmar, C. E. De Rezende, M. Manecki, J. Niggemann, A. R. C. Ovalle, A. Stubbins and M. C. Bernardes, Continuous flux of dissolved black carbon from a vanished tropical forest biome, Nat. Geosci., 2012, 5, 618–622 CrossRef CAS.
- S. J. Balogh, Y. H. Nollet and H. J. Offerman, A comparison of total mercury and methylmercury export from various Minnesota watersheds, Sci. Total Environ., 2005, 340, 261–270 CrossRef CAS PubMed.
- J. Xia, J. Wang, L. Zhang, C. W. N. Anderson, X. Wang, H. Zhang, Z. Dai and X. Feng, Screening of native low mercury accumulation crops in a mercury-polluted mining region: agricultural planning to manage mercury risk in farming communities, J. Cleaner Prod., 2020, 262, 121324 CrossRef CAS.
- Z. Zhao, D. Wang, Y. Wang, Z. Mu and J. Zhu, Wet deposition flux and runoff output flux of mercury in a typical small agricultural watershed in Three Gorges Reservoir areas, Environ. Sci. Pollut. Res., 2015, 22, 5538–5551 CrossRef CAS PubMed.
- C. D. Matthaei, J. J. Piggott and C. R. Townsend, Multiple stressors in agricultural streams: interactions among sediment addition, nutrient enrichment and water abstraction, J. Appl. Ecol., 2010, 47, 639–649 CrossRef.
- C. R. Townsend, S. S. Uhlmann and C. D. Matthaei, Individual and combined responses of stream ecosystems to multiple stressors, J. Appl. Ecol., 2008, 45, 1810–1819 CrossRef.
- R. Karimi, C. Y. Chen, P. C. Pickhardt, N. S. Fisher and C. L. Folt, Stoichiometric controls of mercury dilution by growth, Proc. Natl. Acad. Sci. U. S. A., 2007, 104, 7477–7482 CrossRef CAS PubMed.
- P. C. Pickhardt, C. L. Folt, C. Y. Chen, B. Klaue and J. D. Blum, Algal blooms reduce the uptake of toxic methylmercury in freshwater food webs, Proc. Natl. Acad. Sci. U. S. A., 2002, 99, 4419–4423 CrossRef CAS PubMed.
- P. A. Meyers, Applications of organic geochemistry to paleolimnological reconstructions: a summary of examples from the Laurentian Great Lakes, Org. Geochem., 2003, 34, 261–289 CrossRef CAS.
- S. Zechmeister-Boltenstern, K. M. Keiblinger, M. Mooshammer, J. Peñuelas, A. Richter, J. Sardans and W. Wanek, The application of ecological stoichiometry to plant–microbial–soil organic matter transformations, Ecol. Monogr., 2015, 85, 133–155 CrossRef.
- J. R. D. Guimarães, M. Meili, L. D. Hylander, E. de C. e Silva, M. Roulet, J. B. N. Mauro and R. A. de Lemos, Mercury net methylation in five tropical flood plain regions of Brazil: high in the root zone of floating macrophyte mats but low in surface sediments and flooded soils, Sci. Total Environ., 2000, 261, 99–107 CrossRef.
- M. Roulet, M. Lucotte, J. R. D. Guimarães and I. Rheault, Methylmercury in water, seston, and epiphyton of an Amazonian river and its floodplain, Tapajós River, Brazil, Sci. Total Environ., 2000, 261, 43–59 CrossRef CAS PubMed.
- G. Liu, Y. Cai, T. Philippi, P. Kalla, D. Scheidt, J. Richards, L. Scinto and C. Appleby, Distribution of total and methylmercury in different ecosystem compartments in the Everglades: implications for mercury bioaccumulation, Environ. Pollut., 2008, 153, 257–265 CrossRef CAS PubMed.
- C. D. Domingues, L. H. S. da Silva, L. M. Rangel, L. de Magalhães, A. de Melo Rocha, L. M. Lobão, R. Paiva, F. Roland and H. Sarmento, Microbial food-web drivers in tropical reservoirs, Microb. Ecol., 2017, 73, 505–520 CrossRef PubMed.
- C. Savage, P. R. Leavitt and R. Elmgren, Distribution and retention of effluent nitrogen in surface sediments of a coastal bay, Limnol. Oceanogr., 2004, 49, 1503–1511 CrossRef CAS.
- J. L. Teranes and S. M. Bernasconi, The record of nitrate utilization and productivity limitation provided by δ15N values in lake organic matter—A study of sediment trap and core sediments from Baldeggersee, Switzerland, Limnol. Oceanogr., 2000, 45, 801–813 CrossRef CAS.
-
X. Feng, B. Meng, H. Yan, X. Fu, H. Yao and L. Shang, in Biogeochemical Cycle of Mercury in Reservoir Systems in Wujiang River Basin, Southwest China, ed. X. Feng, B. Meng, H. Yan, X. Fu, H. Yao and L. Shang, Springer, Singapore, 2018, pp. 303–338 Search PubMed.
-
S. M. Khopkar, Environmental Pollution Monitoring and Control, New Age International, 2007 Search PubMed.
- J. E. Gray and M. E. Hines, Biogeochemical mercury methylation influenced by reservoir eutrophication, Salmon Falls Creek Reservoir, Idaho, USA, Chem. Geol., 2009, 258, 157–167 CrossRef CAS.
- Y. Wang, D. Yin, Y. Xiang, Q. Xu, C. Zhang, Q. Xie and D. Wang, A Review of Studies on the Biogeochemical Behaviors of Mercury in the Three Gorges Reservoir, China, Bull. Environ. Contam. Toxicol., 2019, 102, 686–694 CrossRef CAS PubMed.
- D. Kasper, B. R. Forsberg, J. H. F. Amaral, R. P. Leitão, S. S. Py-Daniel, W. R. Bastos and O. Malm, Reservoir Stratification Affects Methylmercury Levels in River Water, Plankton, and Fish Downstream from Balbina Hydroelectric Dam, Amazonas, Brazil, Environ. Sci. Technol., 2014, 48, 1032–1040 CrossRef CAS PubMed.
- D. Kasper, E. F. A. Palermo, C. W. C. Branco and O. Malm, Evidence of elevated mercury levels in carnivorous and omnivorous fishes downstream from an Amazon reservoir, Hydrobiologia, 2012, 694, 87–98 CrossRef CAS.
- J. D. Lalonde, M. Amyot, A. M. L. Kraepiel and F. M. M. Morel, Photooxidation of Hg(0) in Artificial and Natural Waters, Environ. Sci. Technol., 2001, 35, 1367–1372 CrossRef CAS PubMed.
- R. A. Bodaly, J. W. M. Rudd, R. J. P. Fudge and C. A. Kelly, Mercury concentrations in fish related to size of remote Canadian Shield lakes, Can. J. Fish. Aquat. Sci., 1993, 50, 980–987 CrossRef CAS.
Footnote |
† Electronic supplementary information (ESI) available. See DOI: 10.1039/d1em00156f |
|
This journal is © The Royal Society of Chemistry 2021 |