Microaerobic conditions in anaerobic sludge promote changes in bacterial composition favouring biodegradation of polymeric siloxanes†
Received
6th April 2021
, Accepted 17th July 2021
First published on 20th July 2021
Abstract
Volatile organic silicon compounds (VOSiC) are harmful pollutants to the biota and ecological dynamics as well as biogas-based energy conversion systems. However, there is a lack of understanding regarding the source of VOSiCs in biogas, especially arising from the biochemical conversion of siloxane polymers such as polydimethylsiloxanes (PDMS). The biodegradation of PDMS was evaluated under anaerobic/microaerobic conditions (PO2 = 0, 1, 3, 5%), using wastewater treatment plant (WWTP) sludge as an inoculum and PDMS as a co-substrate (0, 50, 100, 500 ppm). On average, strictly anaerobic treatments produced significantly less methane than the 3 and 5% microaerated ones, which show the highest PMDS biodegradation at 50 ppm. Thauera sp. and Rhodococcus sp. related phylotypes were identified as the most abundant bacterial groups in microaerated treatments, and siloxane-related molecules were identified as remnants of PDMS catabolism. Our study demonstrates that microaeration promotes changes to the native bacterial community which favour the biological degradation of PDMS. This confirms that the presence of VOSiC (e.g., D4–D6) in biogas is not only due to its direct input in wastewaters, but also to the PDMS microbial catabolism. Microaerobic conditions enhance both PDMS and (subsequent) VOSiC degradation in the liquid phase, increasing the concentrations of D4 and D5 in biogas, and the production of less toxic siloxane-based derivatives in the liquid phase. This study suggests that microaeration of the anaerobic sludge can significantly decrease the concentration of PDMSs in the WWTP effluent. However, for WWTPs to become effective barriers for the emission of these ecotoxic contaminants to the environment, such a strategy needs to be coupled with an efficient biodegradation of VOSiCs from the biogas.
Environmental significance
Siloxanes are considered emergent contaminants due to their recalcitrant nature, bioaccumulation potential, and harmful effects on human health. For wastewater treatment plants (WWTPs), siloxanes result in millions of dollars in annual losses due to costly physicochemical processes used to remove volatile siloxanes from the anaerobic digestion (AD) biogas prior its use as energy source. This work shows that microaerobic conditions induce changes in the native AD microbial community, allowing degradation of siloxane polymers and methane production from its metabolic products. These results open a new area of research on the treatment and control of siloxanes and other, previously thought recalcitrant compounds. Microaeration may be a viable and cost-effective alternative to remove siloxanes, mitigating its effects in WWTPs and the environment.
|
1. Introduction
Anaerobic digestion (AD) is probably the most environmentally sustainable and cost-effective technology to manage sewage sludge in wastewater treatment plants (WWTP). It allows efficient organic matter stabilisation and energy production, which can be used on-site for the internal operations of the facility. AD takes place in the absence of oxygen, where organic matter is converted into biogas – a gas composed of methane, carbon dioxide, and water vapour. Depending on the characteristics of the influent waste stream, AD biogas can also contain trace concentrations of pollutants, notably hydrogen sulphide (H2S) and volatile organic silicon compounds (VOSiC). Octamethylcyclotetrasiloxane (D4) and decamethylcyclopentasiloxane (D5) cyclic siloxanes are the most common VOSiCs present in biogas,1,2 causing severe, usually irreversible damage to the internal components of the energy generation systems which convert biogas into secondary forms of energy.3,4 Specifically, silicate deposits,5 formed inside combustion engines,6 decrease the thermal conductivity and lubrication of components,7 clog the pistons and lines,6 reduce compression efficiency,5 and cause overheating issues.7 For WWTPs, this results in millions of dollars in annual losses due to the need to invest in filters and technologies to remove VOSiCs from biogas. Siloxanes can increase operating costs up to €0.02 per m3 of biogas treated and add an extra €44
000 of annual expenses.5
PDMS, and particularly VOSiCs, were especially used in the ‘90s to replace or clean chlorofluorocarbons (CFC),8,9 hydrochlorofluorocarbons (HCFC),9,10 and parachlorobenzotrifluoride (PCBTF),9,10 due to – what appeared at the time – their “environmentally friendly” characteristics.8,11 Despite that the siloxane industrial use could be dated to 1940,12 they still are a critical component of personal care products. Siloxanes begin their journey to the WWTP after being rinsed down the drain by the consumer. Once there, siloxanes (as emergent contaminants) can be released to the atmosphere by different physicochemical and biological processes that transform non-volatile to volatile siloxanes.13,14 Also, siloxane-related compounds have been linked to a variety of health impacts on different animal models and possibly also in human populations.12,15,16 For example, exposure to D4 is classified as human hazard class reproductive toxicity 2 by the European Chemicals Agency17 after several investigations and reports on animal models. D5 also can cause health issues to the nervous system, cancer, and hormonal disorders according was reported by the US Office of Environmental Health Hazard Assessment (OEHHA).18 The widespread use of siloxanes in many industries (e.g., cosmetic, automotive, medical, and food processing) has led to an estimated VOSiC emission between 1.4–4.2 g per year per capita in the UK and 0.4–85 g per year per capita in the US, solely from personal care products.19 VOSiCs have been recently classified as substances of very high concern (SVHC) for the environment by the Registration, Evaluation, Authorisation and Restriction of Chemicals (REACH) organisation,17,20 as well as by countries such as the US,21 Japan,22 Canada,23,24 and the UK.25 These countries have framed D4 and D5 siloxanes as substances with high production volume, persistence, bioaccumulation, and ecological concern.
Through the WWTP processes, VOSiCs could be desorbed and volatilised by the aeration process (Fig. 1, steps 5 and 6) or remain adsorbed onto the bio-sludge (Fig. 1, steps 3, 4, 7, 8). Usually, siloxanes, and specially PDMS, remain attached to the organic matter (OM) due to their high affinity with the solid matrix.1,26 In the AD stage, the increased temperature of the process weakens the physical forces that maintain the siloxane molecules attached to the OM, releasing them into the biogas (Fig. 1, steps 9 and 10).1,26 Currently, adsorption using granular activated carbon (GAC) is the most common process used by WWTPs to remove siloxanes.27 However, critical disadvantages have been reported, mainly related to its inherent unspecific adsorption characteristics and the number of competitive adsorption reactions among the pollutants present in biogas streams.28,29 Moreover, physicochemical processes, such as GAC adsorption, are costly, and only separate pollutants, concentrating them onto a different phase, thus not solving the environmental problem. Other technologies, namely membrane separation, chemical absorption (with strong acid or alkali solutions), catalysis, and deep chilling, have severe drawbacks, which make these processes not affordable for most WWTPs.13,14,30
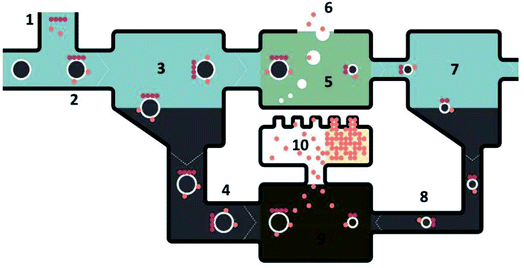 |
| Fig. 1 Possible pathway of siloxanes in a typical WWTP process. (1) PDMS and non-volatile siloxanes (red dots), VOSiC (orange dots) are released into wastewater streams, (2) PDMS and VOSiC attach to the organic matter, OM (brown circles), (3) a fraction of the OM settles with siloxanes in the primary sludge, (4) primary sludge to AD, (5) OM to the aerobic treatment where PDMS can be partially cleaved and VOSiC molecules released, or retained on OM, (6) VOSiCs released to the atmosphere by the aeration process, (7) OM, along with siloxanes, settle in the secondary sludge, (8) secondary sludge to AD, (9) PDMS are cleaved to VOSiCs, which along with existing VOSiC are released to the biogas stream, (10) SiO2 molecules depositing on combustion engine components. | |
On the other hand, biologically based methods certainly constitute the most sustainable and economical alternatives to use. However, studies on the biological degradation of siloxanes are scarce, mainly focused on VOSiCs, and particularly under anaerobic environments.19 Precisely, the high dissociation energy of the Si–O and Si–C bonds31 making the biodegradation of the siloxane bonds difficult to achieve,2 especially in siloxane polymers and absence of higher energy electron acceptors in the anaerobic digestion trophic web. Siloxane biodegradation studies have been focused mostly on D4 or D5 removal; however, no information on the microbial groups or the biochemical interactions involved in siloxanes degradation has been provided. These efforts have been focused on using oxygen as a final electron acceptor mainly by filamentous fungi and aerobic bacteria (i.e., Pseudomonas sp.).32–34 Aerobic conditions seem to be suitable for a more heterogeneous and diverse group of bacteria,35 which may produce the required enzymatic machinery to hydrolyse siloxanes.33,36 However, the aerobic regime has drawbacks such as the increased biomass yield, and deficient syntrophic microbial relationships due to the ecological competitions for space and resources.35 In addition, a strict aerobic environment may be insufficient to ensure complete degradation of organic matter in general,37 recalcitrant compounds38 or siloxanes. Alternatively, the controlled addition of small amounts of oxygen to the anaerobic environment (i.e., microaeration) can promote the required microbial changes and fulfil the thermodynamic requirements to allow for the catabolism of more recalcitrant compounds in general.39–41 A microaerobic environment may help overcome the low energy production, low microbial diversity, and weak biofilm formation issues of strictly anaerobic environments,41–43 while increasing hydrolysis rates and produce more diverse enzymes.38,44 Consequently, microaeration arises as a useful strategy to extract the positive aspects of both aerobic and anaerobic conditions without their drawbacks. This strategy could lead to the biodegradation of complex polymers such as PDMS.
As VOSiCs are present in the personal care products reaching the WWTP influent stream,14 it has been assumed that only this input is the responsible for the damage to combustion engines. Therefore, most of studies mainly refer to D4 and D5 (ref. 19, 45 and 46) without taking into account the potential contribution of siloxane polymers to biogas contamination. It is well known that PDMS can be abiotically degraded in soils by clay acidic minerals with Lewis acid sites (Al3+ or Fe3+) under low moisture conditions.2,47 However, it is not known if PDMS can be abiotically cleaved under the conditions of the AD sludge. It is also unclear if PDMS can be biologically degraded under aerobic32 or anaerobic conditions,48–50 considering that previous studies have reported partial to no biodegradability of PDMS under either condition. Yet, if PDMS could indeed be microbially degraded within the anaerobic sludge, it would likely constitute an additional source of VOSiCs within the AD reactor itself. And, if microaeration enhances PDMS biodegradation, it will result in even further VOSiCs released to the biogas. Based on these premises, three important potential impacts should be considered: first, microaeration may increase concentrations of VOSiCs in biogas and, unless strategies to remove them from the biogas phase are in place, their impact on the energy generation systems of WWTPs may continue; second: the ability of microorganisms to degrade siloxane polymers and use them as carbon source for methane production, may supress or decrease the release of VOSiCs to the environment from post-digested sludge when used as soil amendment; third, the overall biodegradability enhancement of sludge-attached siloxanes (i.e., VOSiCs and PDMS) through microaeration will protect the environment and possibly reduce the release of VOSiCs to the biogas, particularly if residence times are long enough.
Based on above discussion, the aim of this study is to shed some light in following questions: are polymeric siloxanes biodegradable? If so, will biochemical transformations of PDMS within the anaerobic digester produce additional VOSiCs in the biogas? Can microaeration (in contrast to strict anaerobiosis) improve the biodegradability of PDMS and the overall conversion of organic matter to methane during anaerobic digestion? Can microaeration drive changes in the native microbial community of anaerobic digesters? Do trace amounts of oxygen affect methane production and effluent products? To answer these questions, we evaluated the biodegradability of siloxane polymers under either anaerobic or microaerobic conditions, assessing the production of D4 and D5 in biogas and the presence of PDMS catabolic by-products in the liquid phase. Performance of anaerobic digestion was evaluated under increasing oxygen partial pressures and PDMS concentrations, using the biochemical methane potential (BMP) assay. Finally, microbial ecology changes were characterised for each experimental condition.
2. Materials and methods
Inoculum and substrate
Microbial inoculum was obtained from the effluent of an active anaerobic continuously stirred tank reactor (CSTR), treating secondary sludge from “La Farfana” municipal WWTP (Santiago, Chile). The inoculum was degassed by incubation for 24 h at 37 °C before the experimental set up, to ensure that the endogenous microbiota degraded the residual organic matter still present in the sludge. After degassing, the inoculum was characterised in terms of chemical oxygen demand (COD = 5.65 mg O2 per g), total solids (TS = 28.9 g L−1), volatile solids (VS = 15.2 g L−1) and pH (6.5) to then be added to each BMP bottle at an inoculum-to-substrate (I/S) ratio of 2.51−53
In order to promote and sustain initial microbial growth, reduce the lag phase, and favour enzymatic diversity within the BMP, 200 ppm of organic substrate (OS) were added to each bottle. The OS used was based on the equal mixture (COD basis) of glucose, sodium acetate, sodium casein, cellulose (technical grade-Merck), and coconut oil (industrial grade). Selected micronutrients, considered critical for the AD microbiome,54,55 were also added to each BMP bottle (Table S1 – ESI†).
Biochemical methane potential (BMP) assay
The BMP protocol was based on Labatut et al.,56 using 250 mL Schott bottles as reaction units with 50 mL of effective sample volume. BMP bottles were initially loaded with an organic substrate mixture (OS),55 PDMS, micronutrient solution, and WWTP sludge as microbial inoculum. PDMS (100 cSt – Texas Inc.) was added to each BMP bottle at the following concentrations: 50, 100, and 500 ppm in a mass basis. Once loaded, the bottles were gassed with pure nitrogen, sealed, and placed in a shaker incubator (90 rpm, 37 °C ± 1). 12 days after the BMP experimental set up started, pure oxygen (99.8%) was added to each bottle by internal atmospheric volume substitution, using gas tight syringes, to reach the following target partial pressures: 0%, 1%, 3% and 5% v/v. Oxygen was re-placed weekly on a biogas day measurement (when necessary) to maintain the selected microaerated conditions. Tested oxygen partial pressures were chosen based on other reports of microaerophile biota growth (i.e., 1–10% PO2)57,58 and reported methanogenic archaea tolerance (i.e., <5% PO2).59
Additional bottles only containing inoculum but no organic substrate, micronutrients, or added oxygen were incubated to account for background methane production from the sludge itself, which was then subtracted from the other treatments at the end of the assay. As well, treatments with organic substrate, micronutrients, oxygen and not PDMS were performed (i.e., blanks). The BMP assay was ended when the cumulative biomethane production curve reached a plateau phase, which was on average, 55 days. An infographic with the experimental design, including treatments and analyses, is shown in Fig. S1 – ESI.† Also, a detailed description of the oxygen addition procedure is included in MM1 – ESI.† Biogas volumetric production in each BMP bottle was measured using a glass syringe using the volume displacement method. For every biogas production measurement, methane content and volume were determined by pumping the biogas through a two-step system based on Standard Methods 2720B, where a first vessel containing NaOH (20% m/v) removes carbon dioxide and a second vessel, containing MgSO4, retains water, obtaining methane volume by difference. These measurements were confirmed weekly using a gas chromatography thermal conductivity detector (GC-TCD), which in addition to methane and carbon dioxide, determine oxygen and nitrogen in the bottles' gas phase according to the Standard Methods 2720C.
Theoretical biochemical methane potential and biodegraded fraction
The theoretical biochemical methane potential of the organic substrate (OS) and the different PDMS concentrations was estimated using the Buswell equation,60 which assumes that all the organic content is converted to methane and carbon dioxide. Buswell estimations were performed with the molecular formula of each compound in the OS (i.e., glucose, sodium acetate, sodium casein, cellulose, and coconut oil). For coconut oil, the calculation was based on a mixture of capric, lauric, myristic, oleic, and palmitic acid, according to the characterization reported by Otamiri et al.61 characterisation. Finally, the molecular formula CH3(C2H6SiO)nSi(CH3)3 was used for the PDMS calculation, assuming 20 siloxane units (n = 20) in a 100 cSt-siloxane oil. The biodegraded fraction (fD), which defines the maximum extent of substrate converted to methane, was determined as follows: |  | (1) |
where, fD is the substrate biodegraded fraction (decimal, %), Bo and Bu correspond to the observed and theoretical methane potential (mL CH4 per g VS added or g COD added), respectively. Bo was determined directly from the BMP assay, whereas Bu was calculated using the Buswell formula, as described above.
Characterisation of bacterial community dynamics
Changes in the bacterial communities were characterised using the Denaturant Gradient Gel Electrophoresis (DGGE) fingerprinting technique and 16S rRNA gene sequencing. The Operative Taxonomic Unit (OTU) was defined per phylotype, assuming each one of the different bands elucidated on the DGGE gel corresponded to one of the most dominant bacterial populations present under the tested conditions,62 here in particular for the different PDMS concentrations and oxygen partial pressures. From the BMP initial sludge and final digestate (from the combined triplicates), total DNA extraction was performed using a DNeasy power soil kit (Quiagen Inc) extraction kit. Extracted DNA integrity was assessed by 1% agarose electrophoresis gel-red stained, according to Chen et al.63 and Cheng et al.64 methodologies. 16S rRNA gene amplification from bacterial populations was accomplished by Polymerase Chain Reaction (PCR), using universal bacterial primers 358F-GC and 907R. For DGGE analyses, a 40 bp GC clamp was added to the 5′ extreme of the forward primer.62 Bacterial community profiles in DGGE were conducted for amplicons of ±500 bp in length.
DGGE electrophoresis was performed using a 0.75 mm thick polyacrylamide gel (acrylamide
:
bisacrylamide – 37.5
:
1) submerged in a TAE buffer (40 mM Tris, 40 mM acetic acid, 1 mM EDTA; pH 7.4) at 60 °C.62 Denaturant gradient was carried out in urea–formamide at differential concentrations between 40% to 80%.62,65 The DGGE was run at 100 V for 16 h and later stained with SYBr Gold (Molecular Probes) at 0.01% for 30 min to then be revealed using UV transilluminator equipment (BioRad Technologies). Most-intensive, visible profile bands were excised from the DGGE gel, considering the assumption of each band represented a different bacterial population. The 16S rRNA gene from DNA in each band was amplified using the same previously described primers without the 40 bp GC clamp.62 PCR products were confirmed by 1% electrophoresis on an agarose gel prior shotgun sequencing (Macrogen Inc.). DGGE band sequences were compared to the 16S ribosomal RNA sequences (Archaea and Bacteria) database (NCBI) using BLASTN (Megablast program, default parameters).66 The first 50 sequences were downloaded from NCBI and clustered to 97% identity using cd-hit-est.67 The representative 16S rRNA sequences were aligned using MAFFT (auto mode, L-INS-i strategy).68 The 16S rRNA phylogenetic tree was inferred by maximum likelihood (ML) with IQ-TREE v1.6.8 (-m TESTNEW-bb 1000-alrt 1000).69,70 The DGGE bands were phylogenetically positioned using the 16S rRNA ML tree as a reference using EPA-ng v0.3.6,71 to then be visualised and edited with iTOL.72
Analytical and statistical methods
Biogas composition was measured using an Agilent 7820A GC-TCD with a Carbosphere 1010 capillary column with helium as a carrier gas, according to ASTM method D3612.73 The BMP anaerobic sludge was characterised pre- and post-digestion for the following physicochemical parameters: total solids (APHA- TS2540B), chemical oxygen demand (APHA-COD5220D-), fixed and volatile solids (APHA-TVS-2540E-) in accordance to Standard Methods.74 The lipophilic fraction of siloxanes was recovered from the pre- and post-digestion anaerobic sludge by solvent-assisted extraction75,76 (from both the solid and liquid fractions) using a n-hexane-acetone mixture (1
:
1-v/v-). Extracted cyclic siloxanes, D4 and D5, were determined using an Agilent 7820A GC equipped with a flame ionisation detector (FID) and an HP-5 capillary column, as described in Popat and Deshusses.77 Chemical structure identification of extracted compounds was performed using a Shimadzu 8050 gas chromatography-mass spectrometer (GC-MS) triple quadrupole. Samples were injected in a splitless mode, and separation was carried out using a capillary column-Rtx-5MS (30 m × 0.25 mm × 0.1 μm) with a helium flow of 1 mL min−1 as a gas carrier, according to Sanchís et al.78 Selected ion monitoring was used to elucidate siloxanes presence, and molecules were identified by comparing the NIST14.L mass-spectra library based on an 85% similarity for the cut-off. As phenyl-siloxanes were identified using this method, column bleeding was tested running samples with the solvent extraction mixture alone under the same protocol as real samples,79 assuring no column bleeding. Further details on analytic measurements and quality assurance/quality control (QA/QC) strategies can be found in MM2 – ESI.†
All treatments and determinations were performed in triplicate. First, parametric statistical assumptions were tested using the Levenne (homoscedasticity), Kolmogorov–Smirnov (normality), and residual regression model (graphical normality) tests. Then, all the data were analysed under a General Linear Model (GLM) under the test for factorial analysis of variance (2-way ANOVA) to elucidate the interactions of the tested factors. Finally, the HDS Tukey and Scheffé tests were performed as post hoc analysis. For all the tests, the α error assumed was 0.05 under a significance of 95%, using the IBM SPSS statistics 22 software (IBM).
3. Results and discussion
Results based on the biochemical methane potential (BMP) assay show that microorganisms under either anaerobic or microaerated conditions could use the organic substrate (OS). Furthermore, strictly anaerobic conditions produced 11%, 17%, and 25% less methane than the microaerobic conditions, respectively under 3%, 5%, and 1% oxygen partial pressures (PO2), without PDMS added (Fig. 2 – green background). The calculated theoretical biochemical methane potential for OS, OS + PDMS 50 ppm, OS + PDMS 100 ppm, and OS + PDMS 500 ppm, were 39.45 mL, 45.99 mL, 52.53 mL, and 104.83 mL, respectively (Fig. 2). The 100 ppm treatment results were omitted for clarity in the graphs, given that they were almost identical (ANOVA: P < 0.05; α0.05) to the 50 ppm treatment (Fig. S2 – ESI†).
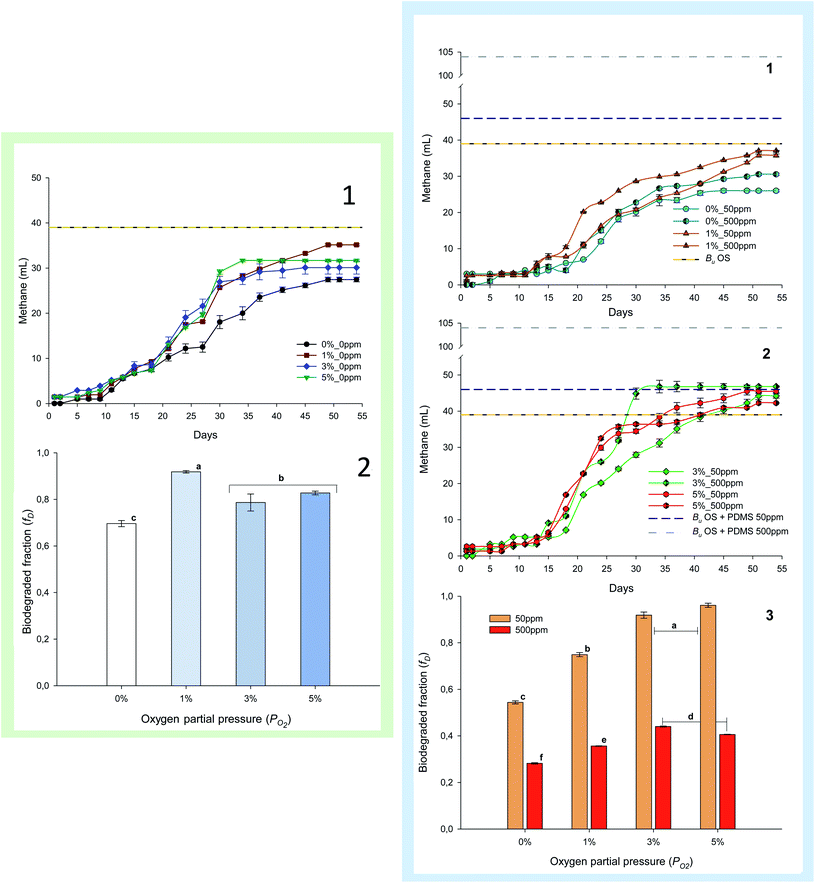 |
| Fig. 2 Green background (GB): cumulative methane production (1) and biodegraded fraction (2) of the organic substrate (OS) alone under anaerobic and microaerated conditions (i.e., blanks). Blue background (BB): cumulative methane production (1 and 2) and biodegraded fraction (3) of the organic substrate (OS) supplemented with increasing PDMS concentrations under anaerobic and microaerated conditions. Methane production is normalised by the substrate's chemical oxygen demand (COD), including PDMS, when applicable, and it is expressed at STP conditions. The yellow, blue, and grey segmented lines in both backgrounds denote the theoretical biochemical methane potential (Bu) of the OS, OS + PDMS 50 ppm, and OS + PDMS 500 ppm, respectively. Letters a, b, c, d, e, f denote HSD Tukey groups with significant differences (α0.05). | |
Microaerated conditions favour the overall methane production of simple and recalcitrant compounds
Results show that methane production was not negatively affected by the change from anaerobic to microaerobic conditions (Fig. 2). In fact, methane production was improved on a volumetric basis. As expected, no treatments reached the theoretical methane production due to the overestimation from the Buswell equation value that does not consider the fraction of substrate allocated to microbial synthesis.80 The anaerobic treatments (i.e., without oxygen) reached 70% of the theoretical methane production of the OS (fD = 0.7), whereas the microaerated treatments (i.e., PO2 = 1, 3, and 5%) reached between ca. 80 and 90% (Fig. 2 GB). This is in agreement with the results of previous studies, where the addition of small amounts of oxygen has increased methane production and organic matter removal81,82 – a result that previous studies have attributed to an improvement in the hydrolytic capabilities of the microbial consortium.83,84 In this case, microaeration promoted the development and/or enhancement of facultative anaerobes (i.e., heterotrophs) which also exhibit higher enzymatic diversity than the anaerobic counterpart. Also, as facultative organisms can use the available dissolved oxygen in the medium, an increased microbial growth, and therefore higher enzymatic production to catabolise siloxanes or the organic matter. An example of this assumption is Thauera sp. dominance in the microaerated conditions (Fig. 3), this organism is also related to an increase in the biodegradation potential of siloxanes and organic matter due to its widely reported enzymatic diversity.85 The latest, evidence how small oxygen amounts can shape the microbial ecology and therefore the enzymatic potential to enhance the recalcitrant compounds biodegradation. As for the OS supplemented with PDMS, higher methane yields were observed both, for increasing PDMS concentrations and for increasing oxygen partial pressures (PO2) up to 3% (Fig. 2 BB 1, 2). Therefore, comparing the results with the supplemented PDMS treatments with the blanks suggest that extra methane production comes from the PDMS catabolism. As well it is clear that treatments with PDMS exceed the theoretical methane production calculated from the organic substrate alone (Fig. 2 BB yellow line). This indicates that PDMS may be used as a carbon source for methane production despite being only partly biodegradable under these conditions.
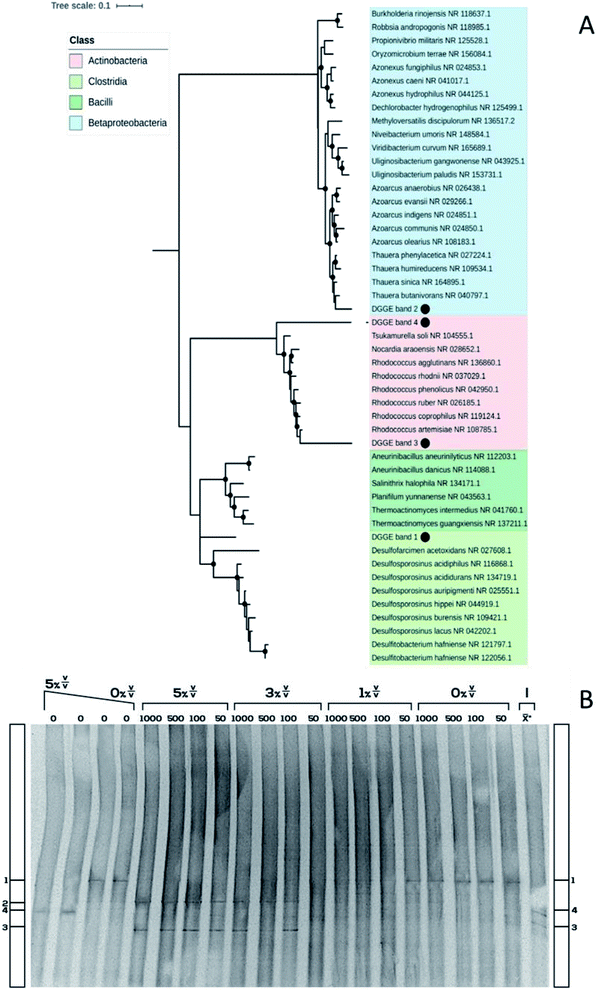 |
| Fig. 3 (A) Maximum likelihood inferred 16S rRNA phylogenetic reconstruction from excised DGGE bands. Black circles correspond to a 1000 bootstrap, SH-aLRT ≥80% and ultra-fast bootstrap ≥95%. (B) DGGE fingerprinting image from the BMP sludge with anaerobic treatment (0% v/v), microaerobic 1% v/v O2, 3% v/v O2, 5% v/v O2 and inoculum (I). Numbers on the top of each column (0, 50, 100, 500 and 1000 ppm) correspond to PDMS concentrations per treatment, * corresponds to the PDMS concentration inferred from literature. Dominant DGGE bands correspond to the chart at the bottom and the phylogenetic reconstruction. | |
The biodegraded fraction decreases along with by PDMS concentration increment, particularly under strictly anaerobic conditions. As well, biodegraded fraction increases with the oxygen partial pressures showing a better performance in the general biodegradation of the used substrate (Fig. 2 BB 3). For example, the biodegraded fraction of the OS supplemented with 50 ppm PDMS increased from 0.54 to 0.96 under oxygen partial pressures of 0% and 5%, respectively. However, the biodegraded fraction of the OS supplemented with 500 ppm PDMS was only 0.44 and 0.41 under oxygen partial pressures of 3% and 5% and decreased even further under anaerobic conditions (fD = 0.28). This suggests that high concentrations of PDMS, or the products of its hydrolysis, may be inhibitory to the anaerobic and/or aerobic microbiota, but its effects may become less significant to the trophic web under microaerobic conditions (1 ≤ PO2 ≤ 3%). Indeed, a myriad of alcohols such as phenol and aliphatic alcohols, which are known to be toxic to microorganisms,86–90 were found at the end of the BMP trials in the liquid phase characterized by the GC-MS analyses (Fig. S3 – ESI†). Alcohols like methanol are one of the products from the oxidation and subsequent hydrolysis of VOSiCs (i.e., D4 and D5),33 therefore it is apparent that from PDMS hydrolysis also alcohols were produced as demonstrated in this study with antimicrobial compounds such as phenol and aliphatic alcohols.
Results showed that oxygen addition not only can improve the biodegradability of simple substrates (i.e., OS), but also of more complex, recalcitrant compounds (i.e., PDMS). This is not surprising, considering the high energy required to cleave the siloxane (Si–O–Si) and methyl-siloxane (Si–CH3) bonds (i.e., 103 and 69 kcal per mole, respectively31). This type of energy requirements cannot be easily reached by the conventional anaerobic microbiota, which harvests considerably less energy than its aerobic counterpart.91 Based on the energy premise, other studies have used nitrate as a final electron acceptor to overcome the energy requirements for the cleavage of siloxane bonds.92 However, nitrate use creates an anoxic environment where the AD microbial diversity only could be narrowed and become unstable,93 instead of microaeration were general diversity (i.e., enzymatic, microbial) and resilience is greatly increased.57 On the other hand, controlled oxygen additions appear as a more suitable option, due to its easy management, low concentration required, and more regulated redox potential. Furthermore, the anaerobic digestion (AD) microbiome has a lower diversity of microorganisms than its microaerobic counterpart,40,42,43 impacting both the production and variety of hydrolytic enzymes. This is why hydrolysis usually becomes the rate-limiting step for the anaerobic digestion of influent streams composed of particulate and/or recalcitrant compounds.43,94
It is apparent that, in this study microaeration improved methane production which must come from a better enzymatic hydrolysis and therefore a PDMS oxidation and use (as the methane production exceed the theoretical calculations of OS alone) (Fig. 2). For treatments containing OS alone, exponential methane production started after day 10, but for microaerated treatments supplemented with PDMS it started on, or shortly after, day 12, coinciding with the first addition of oxygen to the reactors. Such an effect is apparent when observing the methane production of the strictly anaerobic treatments, which exponential phase took between 18 to 20 days to start (Fig. 2 BB 1). In general, microaerated treatments showed a shorter lag phase, not only coinciding with the beginning of oxygen addition but also with its consumption, as discussed below. Jenicek et al.81 and Cirne et al.93 demonstrated that microaeration decreases the concentration of inhibitory compounds, such as lactic acid, sulphide, and ethanol, resulting in an improved and faster COD conversion. Thus, methane production from siloxane-containing substrates may be faster under microaerobic conditions due to the improved process kinetics resulting from the presence of low dissolved oxygen concentrations.
In summary, the presence of oxygen in trace concentrations is likely to enhance the thermodynamics of the anaerobic digestion due to an increased production and diversity of hydrolytic enzymes; thus, improving process kinetics and biodegradability of both, simple substrates, and siloxane polymers, as can be seen from the comparison between anaerobic and microaerated treatments in our experimental conditions. Nonetheless, microaeration should be tested in each specific case since its effect is highly dependent on the microbial potential and diversity of each sludge, and the bioreactor capabilities to maintain a homogeneous microaerated regime.
Coupled effects of oxygen and PDMS: a shift driver of bacterial community structure and sludge ecology
Comparisons of the 16S rRNA bacterial populations under anaerobic and microaerobic conditions revealed that oxygen dosage may have driven significant changes of the native anaerobic sludge community (Fig. 3). 16S rRNA-DGGE fingerprinting profiles and dominant DGGE bands (here defined as bacterial populations) sequenced, evidenced that the abundance of members closely related to Desulfofarcimen sp. decrease as oxygen partial pressures increase (Fig. 3B, band 1). This shift can be explained by the presence of reactive oxygen species (ROS), produced in redox reactions (triggered by oxygen),41 which are toxic to strict anaerobes95 such Desulfofarcimen sp. These organisms do not have the enzymatic machinery to reduce ROS (i.e., aerobic respiration), consequently causing a decrease in its viability and abundance,96 as evidenced by the disappearance of DGGE band 1. However, in treatments where these organisms were not part of the dominant population (PO2 = 1% treatments 100 to 1000 ppm PDMS) (Fig. 3B, B and 1), methane production did not seem to be compromised. In fact, methane production almost reached the theoretical maximum compared with the ones under anaerobic conditions (Fig. 2, BB1). In these cases, it seems that methanogens may have been benefited from the loss of abundance of Clostridia-related organisms, such as Desulfofarcimen sp., considering that these sulphate-reducing bacteria (SRB) may compete for hydrogen, acetate, and carbon dioxide with methanogens. Furthermore, it is likely that other, non-dominant populations of facultative fermentative organisms efficiently contribute to sustain and maintain the balance of the AD trophic chain, showing an apparent community replacement with the same functional capabilities, as suggested by Wu et al.95 on synergetic systems. Nonetheless, considering the limitations of DGGE analyses, above-mentioned hypotheses must be confirmed with metagenomic and metatranscriptomics further analysis.
Additionally, under microaerobic conditions, the abundance of strict anaerobes decreased, while other, better-adapted populations seemed to have thrived, changing the structure of the native anaerobic sludge microbiota. Consequently, in the 3% and 5% PO2 treatments, aerobic/facultative populations, such as those closely related to Thauera sp. (β-Proteobacteria) and Rhodococcus sp. (Actinobacteria), increased their abundance due to the presence of oxygen as final electron acceptor (Fig. 3B, Bands 2 and 3) – a bacterial replacement that has been shown in previous studies.44,96 The increase in abundance of aerobic bacteria, stimulated by the presence of oxygen, could be the result of cooperative relationships between aerobic and anaerobic organisms.97,98 Aerobic bacteria consume oxygen and may use the extra energy available from respiration to cleave siloxane molecules, thus depleting oxygen concentrations and maintaining oxygen reduction potential (ORP) levels low enough to allow methanogenesis.91 Indeed, our results show that oxygen is rapidly consumed after its addition, requiring subsequent re-additions to re-establish the target partial pressures of each treatment (i.e., PO2 = 1%, 3%, 5%) (Fig. 2 and 4). Accordingly, the population of methanogenic archaea within the BMP microbiota did not show any significant change according to the DGGE profile, suggesting that methanogens were not significantly compromised or affected by oxygen addition. This supports the hypothesis that facultative heterotrophic organisms were able to consume most of the oxygen entering the liquid phase, maintaining its levels low enough to protect methanogenic archaea and avoid disruption of the AD trophic web as a whole.
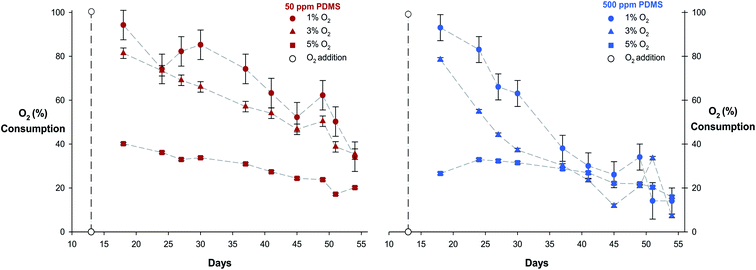 |
| Fig. 4 Oxygen consumption (%) relative to the amount added during the BMP in the PDMS treatments. Vertical segmented lines represent the first addition of oxygen (subsequent additions, after each PO2 measurement, are omitted for clarity). | |
Our results show high rates of oxygen consumption during the exponential methane production stage of the BMP, but it decreases when methane production reaches a plateau phase, and facultative hydrolytic/acidogenic bacteria are expected to be less active (Fig. 2 and 4). Also, oxygen consumption was significantly higher at 1 and 3% oxygen partial pressures as compared to PO2 = 5%, for both 50 and 500 ppm PDMS concentrations (Fig. 4), suggesting that the “extra” available oxygen may have not been used by the aerobic bacteria, and/or, may have been inhibitory to strictly anaerobes. This is supported by the fact that, when the oxygen partial pressure was changed from 3% to 5%, the biodegraded fraction only increased from 0.92 to 0.96 for the 50 ppm PDMS treatments, and even decreased from 0.44 to 0.41 for the 500 ppm PDMS treatments (Fig. 2).
The correlation between oxygen consumption and methane production demonstrates that aerobic/facultative bacteria reduce dissolved oxygen concentrations (and thus oxygen partial pressures), protecting strictly anaerobic methane-producing organisms. This particular mutualistic relationship, studied previously using non-siloxane substrates,42,82,95 supports the hypothesis that the presence of oxygen leads to a change in the bacterial community structure. This change could favour the production of new enzymes and the possibility of harvesting more energy from substrates, which may explain why PDMS is more biodegradable under microaerated conditions. This phenomenon may be the basis that allows the cleavage of the high energy covalent bonds formed by silicon, resulting in the biodegradation of recalcitrant compounds such as siloxanes (i.e., PDMS). Our results show that microaeration coupled with PDMS presence led to an evident change in the bacterial structure and then in the system ecology, evidenced by the differences in population dynamics shown on the DGGE fingerprinting profiles. We could infer that the PDMS cleavage (and biodegradation), requires the oxygen stimulation of the whole anaerobic sludge to bring about specific groups of aerobic/facultative bacteria, which can collaborate and develop mutualistic relationships with the existing microorganisms of the native sludge.95–97
Finally, it should be stressed that the presence of oxygen alone may not be the only driver for the microbial ecology changes observed in the AD microcosm. Microaerated conditions together with the presence of siloxanes, may have been responsible for the increase of Thauera sp. and Rhodococcus sp., as suggested by the DGGE profiles (Fig. 3B). First, because Desulfofarcimen sp. are shown to be dominant under anaerobic conditions and 1% oxygen partial pressures without PDMS addition, as well as under anaerobic conditions with PDMS addition (Fig. 3B, band 1); demonstrating that PDMS by itself is not capable of driving a change in the ecological composition. Second, because when the oxygen partial pressures are ≥3% in treatments without PDMS, the aerobic bacteria Tsukamurella sp. increase its dominance (Fig. 3B, band 4), but they are absent under the same oxygen partial pressures in the PDMS-supplemented treatments, where Thauera sp. and Rhodococcus sp. are dominants. These differences demonstrate that PDMS and oxygen interactions drive the AD sludge ecological changes, enabling the siloxanes biodegradation without hindering methane production (Fig. 2).
Oxygen drives the PDMS metabolism to volatile and non-volatile siloxanes
Proof that the bio stimulation of siloxane-hydrolytic microorganisms is driven by the presence of oxygen is apparent when we analyse the bacterial community structure (Fig. 3) and the metabolic products resulting from the PDMS degradation, as revealed by GC-MS analyses (Fig. 5). The presence of cyclic siloxanes and its relative concentrations (%) per treatment, not only demonstrate that the microbial metabolism of PDMS is feasible, but also that produce cyclic siloxanes such as D7, D8, and D9, as they were not present in the initial, native anaerobic sludge. As the Fig. 5 shows, D7 to D9 siloxanes are absent in the initial sludge and, as the oxygen concentration increases, they begin to appear in concentrations higher than 10% m/m (e.g., D8 at PO2 = 5% and 50 ppm PDMS). Also, Fig. 5 shows that initial concentration of VOSiCs (i.e., D4, D5, and D6) in inoculum sludge increased from 0.60% up to 10% in treatments with oxygen partial pressures of 1% and 3%, evidencing a contribution in VOSiC concentration from the above explained PDMS hydrolysis.
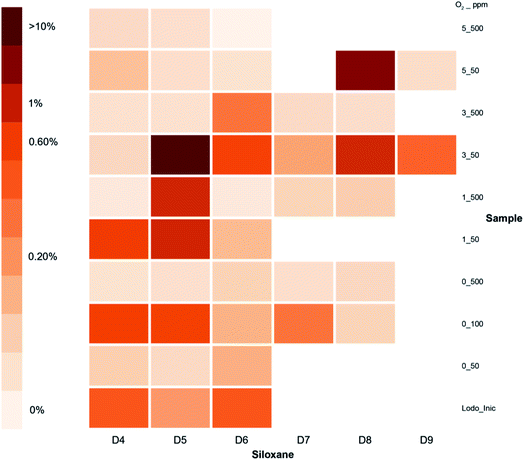 |
| Fig. 5 Heat map showing relative concentrations (% m/m) of cyclic siloxanes identified by GC-MS (QQQ) for the treatments supplemented with PDMS. Left bar shows the colour key according to the relative concentration found for each sample. Right bar shows the treatment pair of oxygen partial pressure (PO2) and PDMS concentration. Cyclic siloxanes were recovered with n-hexane : acetone 1 : 1 solvent extraction. | |
In most anaerobic treatments, short-chain, volatile siloxanes (i.e., D4 to D6) maintained concentrations between 0.03% and 1% (Fig. 5), suggesting that under anaerobic conditions cyclic siloxanes could also be metabolised to silanes or other non-cyclic or volatile metabolic products. Under anaerobic conditions, the presence of long-chain, non-volatile siloxanes (i.e., D7 and D8) was only observed when PDMS concentrations were higher than 100 ppm (Fig. 5). This result could be due to the increase in volatile siloxanes that can be re-polymerised up to D7 and D8 via α-ω-silanediols, as Cabrera-Codony et al.29,99 and Soreanu et al.31 proposed. However, it is also possible that PDMS was only partly catabolised due to the lack of a more energetic final electron acceptor. In this way, remaining products from PDMS cleavage could have undergone a chemical rearrangement into larger cyclic siloxanes, as previously described for soil and anaerobic sludge matrices.2,47 Previous studies report that PDMS is hardly to non-biodegradable under aerobic or anaerobic conditions.2,15,34,100,101 It is suggested that D4 and D5 siloxanes came in wastewaters attached to the AD sludge biosolids (due to their hydrophobicity), and then released to the biogas by volatilisation under mesophilic temperatures.1,19,46 Other studies hypothesised that D4 and D5 present in the biogas also could come from the resulting products of PDMS hydrolysis as compounds that looked for more stable, less energy-repulsive cyclic structures.31,34,102 In our study, when oxygen was present, higher concentrations of volatile cyclic siloxanes (D4 to D6) and non-volatile siloxanes (D7 to D9) were found (Fig. 5 and S4 – ESI†). This demonstrates that metabolic products from PDMS degradation might contribute to the pool of volatile siloxanes found in WWTP-derived biogas.
Also, the presence of intermediates, such as phenyl-siloxanes, linear siloxanes, tripropyl-silanes, and other siloxane-type molecules with fragmentation patterns in mass/charge peaks (m/z) 73 m/z, 355 m/z, and 429 m/z (Fig. S4 – ESI†), strongly suggests that the bacteria present under microaerobic conditions could catabolise the PDMS molecules, despite the characteristic steric hindrance of methyl-siloxane molecules.26,103,104 Furthermore, these metabolic intermediates are susceptible to being re-polymerised into cyclic siloxanes or stabilised to silanols, at which point could be used as a carbon source within the AD trophic chain and produce biogas (Fig. 2). This may explain the presence of higher cyclic siloxanes in the microaerated treatments (Fig. 5) and the resulting increased methane production. As these results demonstrate that microaeration may be a viable alternative to biodegrade siloxane polymers, it may also represent a viable option to catabolize VOSiCs attached to the AD sludge, as our preliminary research shows (data not published). Therefore, while enhanced PDMS degradation may increase the production of VOSiCs, their concentration arising from both direct (influent) and indirect (PDMS degradation) inputs may also decrease, ultimately decreasing biogas pollution. This strategy may become more effective if a strong microaerophilic microbiota is identified and isolated, and used in a separate system (e.g., biotrickling filter) to efficiently remove and catabolise biogas VOSiCs, protecting AD-based energy production systems. In addition, PDMS catabolism in the digester will also protect the environment from the siloxanes released through WWTP biosolids. Indeed, studies15,101 have shown that an important portion of the soils amended with treated AD sludge (biosolids) may be contaminated with organosilicon pollutants, being a potential VOSiCs source and therefore an ecological risk. Above hypotheses must be carefully evaluated in further studies to assess their validity. If they can be proven at commercial stage, it will, in turn, decrease VOSiC concentration in the environment, and open a possibility to reduce subsequent biogas cleaning efforts required for its use in WWTPs.
Our results demonstrate that the microbial metabolism of PDMS is possible under both, microaerobic and (partially) anaerobic conditions, and that the resulting products are transformed into cyclic molecules, namely D4 to D9. This conclusion is critical to understand the fate and production of cyclic siloxane molecules in the AD biogas from PDMS cleavage, which up to now most of the studies refer as slow or negligible.31,34,102
Biochemical considerations in the PDMS biodegradation under a microaerated environment
The diversity of the cyclic siloxanes (different from the ones in the initial sludge Fig. 5) found in this study may be due to the use of oxygen and the presence of siloxanes, which drive the microbial community composition changes in the anaerobic sludge. Oxygen reduction and substrate oxidation likely lead to higher energy yields than those obtained from fermentation. This enables enzymes to harvest the necessary energy to degrade the Si–C bonds in siloxane molecules, yielding additional carbon to produce additional methane, as suggested in this study (Fig. 2). Indeed, it is expected that the new dominant bacterial groups will produce additional and/or new enzymes that may be responsible for the PDMS degradation in the BMP sludge. But, overcoming the steric hindrance of siloxanes and the unfavourable thermodynamics of degradation, requires microbial associations, such as the ones between anaerobic and aerobic bacteria that allowed the extra methane production under oxygen presence. Other studies have evidenced this cooperative behaviour by observing associations between Pseudomonas sp. strains and other α and β-Proteobacteria.32,34 This phenomenon could not be demonstrated in our study due to the limitations of using 16S rRNA-DGGE analyses; however, it is strongly suggested that Thauera sp. and Rhodococcus sp. (β-Proteobacteria and Actinobacteria, respectively) members in this study, might be working together to catabolise PDMS molecules. These organisms are known to have robust enzymatic capabilities to metabolise recalcitrant pollutants, possibly explaining the formation of metabolic products and cyclic siloxanes in our study (Fig. S4 – ESI†).
Several studies have reported that Thauera sp. are the main bacterial group responsible for the decomposition of cyclic hydrocarbons in anaerobic digesters, when nitrate is supplemented as a final electron acceptor.85 However, when oxygen is present, Thauera sp. could yield more energy via aerobic respiration, consequently enhancing metabolic diversity. Under these conditions, the metabolism of aromatic recalcitrant compounds (i.e., phenylsiloxanes in Fig. S4 – ESI†) is possible, mainly through the benzoyl-CoA pathway.105 These natural capacities, suggest that the presence of oxygen enabled Thauera sp. to degrade cyclic siloxane metabolic intermediaries into simpler molecules that can be used by other members of the AD trophic web for biogas production. On the other hand, Rhodococcus sp., a member of the Actinobacteria phylum, is a well-known metabolically diverse organism that could degrade recalcitrant compounds such as naphthalene, aromatic substrates, herbicides, among others.106 One of the reported features of this organism is its capacity to deal with steric hindrances of organic compounds.107 This feature may be key for the degradation and conversion of PDMS and its metabolic intermediates to biogas.
4. Conclusions
This study tested and confirmed the hypothesis that PDMS can be microbially degraded under anaerobic, and especially under microaerated conditions. Also, we found that PDMS catalysis is microbially-mediated producing metabolic intermediates that are less recalcitrant and serve as electron donors for methane production. Results show that trace amounts of oxygen in an otherwise strictly anaerobic environment drive the ecological and biochemical changes needed to biologically degrade PDMS, producing additional methane to that resulting from the degradation of the organic substrate alone. Data suggests that the loss of abundance of strict anaerobes (e.g., Clostridia class-related) and the increase of abundance of facultative anaerobes (e.g., Thauera sp., Rhodococcus sp.) could modify and res-structure the AD trophic chain. Facultative anaerobes, with enhanced metabolic capabilities, can improve the catabolism of organic molecules in general, while increasing the methane production and protecting the anaerobic environment by depleting the oxygen. Our study demonstrates that the presence of VOSiC in biogas may not be the sole result of volatile siloxanes coming in wastewaters, but also a consequence of PDMS microbial catabolism. This process is likely favoured by the traces of oxygen entering the system when loading, resulting in additional VOSiC emissions within the anaerobic digester. Although microaerobic conditions enhances PDMS degradation in the liquid phase, increasing the concentrations of D4 and D5 in biogas, it also enhances the biodegradation of VOSiCs in the liquid phase, resulting in alcohols and other soluble, less toxic derivatives that remain in the liquid phase, thus preventing their release as VOSiCs in the biogas. This study suggests that microaeration of the anaerobic sludge will significantly decrease the concentration of PDMSs in the WWTP effluent. However, for microaeration to be beneficial to WWTPs, and for WWTPs to become barriers for the emission of these ecotoxic contaminants to the environment, such a strategy needs to be coupled with an efficient biodegradation of VOSiCs from the biogas.
Conflicts of interest
There are no conflicts to declare.
References
- Y. Horii, K. Nojiri, K. Minomo, M. Motegi and K. Kannan, Volatile methylsiloxanes in sewage treatment plants in Saitama, Japan: Mass distribution and emissions, Chemosphere, 2019, 233, 677–686, DOI:10.1016/j.chemosphere.2019.05.247.
- C. Rücker and K. Kümmerer, Environmental chemistry of organosiloxanes, Chem. Rev., 2015, 115(1), 466–524, DOI:10.1021/cr500319v.
- S. Pioquinto García, L. Á. Garza Rodríguez and D. Bustos Martínez,
et al., Siloxane removal for biogas purification by low cost mineral adsorbent, J. Cleaner Prod., 2021, 286, 124940, DOI:10.1016/j.jclepro.2020.124940.
- R. Dewil, L. Appels and J. Baeyens, Energy use of biogas hampered by the presence of siloxanes, Energy Convers. Manage., 2006, 47(13–14), 1711–1722, DOI:10.1016/j.enconman.2005.10.016.
- C. A. Hepburn, B. D. Martin, N. Simms and E. J. McAdam, Characterization of full-scale carbon contactors for siloxane removal from biogas using online Fourier transform infrared spectroscopy, Environ. Biotechnol., 2015, 36(2), 178–187, DOI:10.1080/09593330.2014.941310.
- R. Gao, S. Cheng and Z. Li, Research progress of siloxane removal from biogas, Int. J. Agric. Biol. Eng., 2017, 10(1), 30–39, DOI:10.3965/j.ijabe.20171001.3043.
-
G. Piechota, B. Iglí Nski, M. Zaborowicz and W. Qiao, Biomethane in Poland-Current Status, Potential, Perspective and Development, 2021, DOI:10.3390/en14061517, published online.
- J. Sherman, B. Chin, P. D. T. Huibers, R. Garcia-Valls and T. A. Hatton, Solvent replacement for green processing, Environ. Health Perspect., 1998, 106(suppl 1), 253–271, DOI:10.1289/ehp.98106s1253.
- S. R. Lunney, J. F. Szabat, S. J. Landon and J. L. Lombardo, New Developments in Reduced CFC-11 Rigid Foam Appliance Systems, J. Cell. Plast., 1990, 26(6), 524–544, DOI:10.1177/0021955X9002600604.
-
R. F. Burow, Volatile methyl siloxanes (VMS) as replacements for CFCs and methyl chloroform in precision cleaning, in In 8th Annual Aerospace Hazardous Materials Management Conference, 1993 Search PubMed.
- S. Xu, Fate of cyclic methylsiloxanes in soils. 1. The degradation pathway, Environ. Sci. Technol., 1999, 33(4), 603–608, DOI:10.1021/es980803a.
- K. Mojsiewicz-Pieńkowska and D. Krenczkowska, Evolution of consciousness of exposure to siloxanes—review of publications, Chemosphere, 2018, 191, 204–217, DOI:10.1016/j.chemosphere.2017.10.045.
- G. Wang, Z. Zhang and Z. Hao, Recent advances in technologies for the removal of volatile methylsiloxanes: a case in biogas purification process, Crit. Rev. Environ. Sci. Technol., 2019, 49(24), 2257–2313, DOI:10.1080/10643389.2019.1607443.
- M. Shen, Y. Zhang, D. Hu, J. Fan and G. Zeng, A review on removal of siloxanes from biogas: with a special focus on volatile methylsiloxanes, Environ. Sci. Pollut. Res., 2018, 25(31), 30847–30862, DOI:10.1007/s11356-018-3000-4.
- N. De Arespacochaga, C. Valderrama, J. Raich-Montiu, M. Crest, S. Mehta and J. L. Cortina, Understanding the effects of the origin, occurrence, monitoring, control, fate and removal of siloxanes on the energetic valorization of sewage biogas-A review, Renewable Sustainable Energy Rev., 2015, 52, 366–381, DOI:10.1016/j.rser.2015.07.106.
- D. Capela, N. Ratola, A. Alves and V. Homem, Volatile methylsiloxanes through wastewater treatment plants – a review of levels and implications, Environ. Int., 2017, 102, 9–29, DOI:10.1016/j.envint.2017.03.005.
-
European Chemical Agency G., Proposal for Identification of a Substance of Very High Concern on the Basis of the Criteria Set out in Article 57 (Octamethylcyclotetrasiloxane, D4), 2018 Search PubMed.
-
R. Barham and G. Alexeeff, Background Material: 2007-09-13 OEHHA Review of Toxicity Information on D5, 2007 Search PubMed.
- L. Xu, Y. Shi and Y. Cai, Occurrence and fate of volatile siloxanes in a municipal Wastewater Treatment Plant of Beijing, China, Water Res., 2013, 47(2), 715–724, DOI:10.1016/j.watres.2012.10.046.
-
European Chemical Agency G., Annex XV Report Proposal for Identification of a Substance of Very High Concern on the Basis of the Criteria Set out in Article 57 (Decamethylcyclopentasiloxane; D5), 2018 Search PubMed.
-
US EPA, ENFORCEABLE CONSENT AGREEMENT FOR ENVIRONMENTAL TESTING FOR OCTAMETHYLCYCLOTETRASILOXANE (D4) (CASRN 556-67-2) Docket no . EPA-HQ-OPPT-2012-0209, published 2014, accessed 1 December, 2019, https://www.epa.gov/sites/production/files/2015-01/documents/signed_siloxanes_eca_4-2-14.pdf Search PubMed.
- Ministry of Economy Trade and Industry (METI) J., Deliberation Examination Sheet as to Whether or Not It Corresponds to a Class 1 Specified Chemical Substance or a Monitoring Chemical Substance (Siloxanes D4, D5, D&) Chromatography G. Standard Test Method for Analysis of Gases Dissolved in Electrical Insulating Oil by Methods, 2002, 05.
-
Environment Canada-Health Canada, Screening Assessment for the Challenge Octamethylcyclotetrasiloxane (D4), published 2008, accessed 1 December, 2019, https://www.ec.gc.ca/ese-ees/2481B508-1760-4878-9B8A-270EEE8B7DA4/batch2_556-67-2_en.pdf Search PubMed.
-
Environment Canada-Health Canada, Screening assessment for the challenge Decamethylcyclopentasiloxane (D5), published 2008, accessed 1 December, 2019, https://www.ec.gc.ca/ese-ees/13CC261E-5FB0-4D33-8000-EA6C6440758A/batch2_541-02-6_en.pdf Search PubMed.
-
D. Brooke, M. Crookes and D. R. S. Gray, Environmental Risk Assessment Report: Octamethylcyclotetrasiloxane and Decamethylcyclopentasiloxane, 2009, https://assets.publishing.service.gov.uk/government/uploads/system/uploads/attachment_data/file/290561/scho0309bpqx-e-e.pdf Search PubMed.
- G. Gatidou, O. S. Arvaniti, A. S. Stasinakis, N. S. Thomaidis and H. R. Andersen, Using mechanisms of hydrolysis and sorption to reduce siloxanes occurrence in biogas of anaerobic sludge digesters, Bioresour. Technol., 2016, 221, 205–213, DOI:10.1016/j.biortech.2016.09.018.
- M. Arnold and T. Kajolinna, Development of on-line measurement techniques for siloxanes and other trace compounds in biogas, J. Waste Manage., 2010, 30(6), 1011–1017, DOI:10.1016/j.wasman.2009.11.030.
- M. Ajhar, M. Travesset, S. Yüce and T. Melin, Siloxane removal from landfill and digester gas – A technology overview, Bioresour. Technol., 2010, 101(9), 2913–2923, DOI:10.1016/j.biortech.2009.12.018.
- A. Cabrera-Codony, E. Santos-Clotas, C. O. Ania and M. J. Martín, Competitive siloxane adsorption in multicomponent gas streams for biogas upgrading, Chem. Eng. J., 2018, 344, 565–573, DOI:10.1016/j.cej.2018.03.131.
- R. Kapoor, P. Ghosh, M. Kumar and V. K. Vijay, Evaluation of biogas upgrading technologies and future perspectives: a review, Environ. Sci. Pollut. Res., 2019, 26(12), 11631–11661, DOI:10.1007/s11356-019-04767-1.
- G. Soreanu, M. Béland and P. Falletta,
et al., Approaches concerning siloxane removal from biogas – a review, Canadian Biosystems Engineering, 2011, 53 Search PubMed.
- R. Wasserbauer and Z. Zadák, Growth of Pseudomonas putida and P. fluorescens on silicone oils, Folia Microbiol., 1990, 35(5), 384–393, DOI:10.1007/BF02821407.
- Y. Li, W. Zhang and J. Xu, Siloxanes removal from biogas by a lab-scale biotrickling filter inoculated with Pseudomonas aeruginosa S240, J. Hazard. Mater., 2014, 275, 175–184, DOI:10.1016/j.jhazmat.2014.05.008.
- F. Accettola, G. M. Guebitz and R. Schoeftner, Siloxane removal from biogas by biofiltration: Biodegradation studies, Clean Technol. Environ. Policy, 2008, 10(2), 211–218, DOI:10.1007/s10098-007-0141-4.
- L. Shan, Y. Yu and Z. Zhu,
et al., Microbial community analysis in a combined anaerobic and aerobic digestion system for treatment of cellulosic ethanol production wastewater, Environ. Sci. Pollut. Res., 2015, 22(22), 17789–17798, DOI:10.1007/s11356-015-4938-0.
- J. Wang, W. Zhang, J. Xu, Y. Li and X. Xu, Octamethylcyclotetrasiloxane removal using an isolated bacterial strain in the biotrickling filter, Biochem. Eng. J., 2014, 91, 46–52, DOI:10.1016/j.bej.2014.07.003.
- H. Du and F. Li, Characteristics of dissolved organic matter formed in aerobic and anaerobic digestion of excess activated sludge, Chemosphere, 2017, 168, 1022–1031, DOI:10.1016/j.chemosphere.2016.10.108.
- G. Song, Y. Yu, T. Liu, H. Xi and Y. Zhou, Performance of microaeration hydrolytic acidification process in the pretreatment of 2-butenal manufacture wastewater, J. Hazard. Mater., 2019, 369(February), 465–473, DOI:10.1016/j.jhazmat.2019.02.034.
- Z. Cheng, Y. Wei, Q. Zhang, J. Zhang, T. Lu and Y. Pei, Enhancement of surfactant biodegradation with an anaerobic membrane bioreactor by introducing microaeration, Chemosphere, 2018, 208, 343–351, DOI:10.1016/j.chemosphere.2018.06.001.
- P. Jenicek, C. A. Celis, J. Koubova and D. Pokorna, Comparison of microbial activity in anaerobic and microaerobic digesters, Water Sci. Technol., 2011, 63(10), 2244–2249, DOI:10.2166/wst.2011.579.
- D. Nguyen and S. K. Khanal, A little breath of fresh air into an anaerobic system: how microaeration facilitates anaerobic digestion process, Biotechnol. Adv., 2018, 36(7), 1971–1983, DOI:10.1016/j.biotechadv.2018.08.007.
- Y. N. Hou, C. Yang and A. Zhou,
et al., Microbial community response and SDS-PAGE reveal possible mechanism of waste activated sludge acidification enhanced by microaeration coupled thermophilic pretreatment, Process Biochem., 2018, 64(September 2017), 1–8, DOI:10.1016/j.procbio.2017.09.010.
- O. Menezes, R. Brito, F. Hallwass, L. Florêncio, M. T. Kato and S. Gavazza, Coupling intermittent micro-aeration to anaerobic digestion improves tetra-azo dye Direct Black 22 treatment in sequencing batch reactors, Chem. Eng. Res. Des., 2019, 146, 369–378, DOI:10.1016/j.cherd.2019.04.020.
- M. S. Duarte, S. A. Silva and A. F. Salvador,
et al., Insight into the Role of Facultative Bacteria Stimulated by Microaeration in Continuous Bioreactors Converting LCFA to Methane, Environ. Sci. Technol., 2018, 52(11), 6497–6507, DOI:10.1021/acs.est.8b00894.
- D. G. Wang, W. Norwood, M. Alaee, J. D. Byer and S. Brimble, Review of recent advances in research on the toxicity, detection, occurrence and fate of cyclic volatile methyl siloxanes in the environment, Chemosphere, 2013, 93(5), 711–725, DOI:10.1016/j.chemosphere.2012.10.041.
- D. G. Wang and M. Alaee, Fate of Volatile Methylsiloxanes in Wastewater Treatment Plants, Handb. Environ. Chem., 2018, 89, 119–130, DOI:10.1007/698_2018_365.
- R. G. Lehmann, J. R. Miller and G. E. Kozerski, Degradation of silicone polymer in a field soil under natural conditions, Chemosphere, 2000, 41(5), 743–749, DOI:10.1016/S0045-6535(99)00430-0.
- E. J. Hobbs, M. L. Keplinger and J. C. Calandra, Toxicity of polydimethylsiloxanes in certain environmental systems, Environ. Res., 1975, 10(3), 397–406, DOI:10.1016/0013-9351(75)90035-3.
- R. Grümping, K. Michalke, A. V. Hirner and R. Hensel, Microbial degradation of octamethylcyclotetrasiloxane, Appl. Environ. Microbiol., 1999, 65(5), 2276–2278, DOI:10.1128/aem.65.5.2276-2278.1999.
- A. Ohannessian, V. Desjardin, V. Chatain and P. Germain, Volatile organic silicon compounds: the most undesirable contaminants in biogases, Water Sci. Technol., 2008, 58(9), 1775–1781, DOI:10.2166/wst.2008.498.
- A. G. Hashimoto, Effect of Inoculum Substrate Ratio on Methane Yield and Production Rate from Straw Experiment design, Public Health, 1989, 28, 247–255 CAS.
- D. P. Chynoweth, C. E. Turick, J. M. Owens, D. E. Jerger and M. W. Peck, Biochemical methane potential of biomass and waste feedstocks, Biomass Bioenergy, 1993, 5(1), 95–111, DOI:10.1016/0961-9534(93)90010-2.
- F. Raposo, C. J. Banks, I. Siegert, S. Heaven and R. Borja, Influence of inoculum to substrate ratio on the biochemical methane potential of maize in batch tests, Process Biochem., 2006, 41(6), 1444–1450, DOI:10.1016/j.procbio.2006.01.012.
- W. F. Owen, D. C. Stuckey, J. B. Healy, L. Y. Young and P. L. McCarty, Bioassay for monitoring biochemical methane potential and anaerobic toxicity, Water Res., 1979, 13(6), 485–492, DOI:10.1016/0043-1354(79)90043-5.
- I. Angelidaki, M. Alves and D. Bolzonella,
et al., Defining the biomethane potential (BMP) of solid organic wastes and energy crops: a proposed protocol for batch assays, Water Sci. Technol., 2009, 59(5), 927–934, DOI:10.2166/wst.2009.040.
- R. A. R. A. Labatut, L. T. L. T. Angenent and N. R. Scott, Biochemical methane potential and biodegradability of complex organic substrates, Bioresour. Technol., 2011, 102(3), 2255–2264, DOI:10.1016/j.biortech.2010.10.035.
- D. Ruan, Z. Zhou, H. Pang, J. Yao, G. Chen and Z. Qiu, Enhancing methane production of anaerobic sludge digestion by microaeration: Enzyme activity stimulation, semi-continuous reactor validation and microbial community analysis, Bioresour. Technol., 2019, 289(May), 121643, DOI:10.1016/j.biortech.2019.121643.
- J. W. Lim, J. A. Chiam and J. Y. Wang, Microbial community structure reveals how microaeration improves fermentation during anaerobic co-digestion of brown water and food waste, Bioresour. Technol., 2014, 171, 132–138, DOI:10.1016/j.biortech.2014.08.050.
- Y. Tang, T. Shigematsu, Ikbal, S. Morimura and K. Kida, The effects of micro-aeration on the phylogenetic diversity of microorganisms in a thermophilic anaerobic municipal solid-waste digester, Water Res., 2004, 38(10), 2537–2550, DOI:10.1016/j.watres.2004.03.012.
- G. E. Symons and A. M. Buswell, The Methane Fermentation of Carbohydrates, J. Am. Chem. Soc., 1933, 55(5), 2028–2036, DOI:10.1021/ja01332a039.
- F. O. Otamiri, V. N. Ogugua, P. E. Joshua, A. S. Odiba and C. Y. Ukegbu, Physicochemical Characterization of Coconut Copra (Dry Flesh) oil and Production of Biodiesel from Coconut Copra Oil, Jökull Journal, 2014, 64(December 2015), 201–236 Search PubMed , https://www.researchgate.net/publication/286066222_Physicochemical_Characterization_of_Coconut_Copra_Dry_Flesh_oil_and_Production_of_Biodiesel_from_Coconut_Copra_Oil.
- B. Díez, C. Pedrós-Alió, T. L. Marsh and R. Massana, Application of Denaturing Gradient Gel Electrophoresis (DGGE) to Study the Diversity of Marine Picoeukaryotic Assemblages and Comparison of DGGE with Other Molecular Techniques, Appl. Environ. Microbiol., 2001, 67(7), 2942–2951, DOI:10.1128/AEM.67.7.2942-2951.2001.
- C. L. Chen, H. Macarie and I. Ramirez,
et al., Microbial community structure in a thermophilic anaerobic hybrid reactor degrading terephthalate, Microbiology, 2004, 150(10), 3429–3440, DOI:10.1099/mic.0.27193-0.
- L. Cheng, C. Ding, Q. Li, Q. He, D. L. Rong and H. Zhang, DNA-SIP Reveals That Syntrophaceae Play an Important Role in Methanogenic Hexadecane Degradation, PLoS One, 2013, 8(7), 66784, DOI:10.1371/journal.pone.0066784.
-
G. Muyzer and K. Smalla, Application of denaturing gradient gel electrophoresis (DGGE) and temperature gradient gel electrophoresis (TGGE) in microbial ecology, in Antonie van Leeuwenhoek, International Journal of General and Molecular Microbiology, 1998, DOI:10.1023/A:1000669317571.
- C. Camacho, G. Coulouris, V. Avagyan, N. Ma, J. Papadopoulos, K. Bealer and T. L. Madden, BLAST+: architecture and applications, BMC Bioinf., 2009, 10(1), 1–9, DOI:10.1186/1471-2105-10-421.
- W. Li and A. Godzik, Cd-hit: A fast program for clustering and comparing large sets of protein or nucleotide sequences, Bioinformatics, 2006, 22(13), 1658–1659, DOI:10.1093/bioinformatics/btl158.
- K. Katoh and D. M. Standley, MAFFT multiple sequence alignment software version 7: Improvements in performance and usability, Mol. Biol. Evol., 2013, 30(4), 772–780, DOI:10.1093/molbev/mst010.
- L. T. Nguyen, H. A. Schmidt, A. Von Haeseler and B. Q. Minh, IQ-TREE: a fast and effective stochastic algorithm for estimating maximum-likelihood phylogenies, Mol. Biol. Evol., 2015, 32(1), 268–274, DOI:10.1093/molbev/msu300.
- D. T. Hoang, O. Chernomor, A. Von Haeseler, B. Q. Minh and L. S. Vinh, UFBoot2: Improving the ultrafast bootstrap
approximation, Mol. Biol. Evol., 2018, 35(2), 518–522, DOI:10.1093/molbev/msx281.
- P. Barbera, A. M. Kozlov and L. Czech,
et al., EPA-ng: Massively Parallel Evolutionary Placement of Genetic Sequences, Syst. Biol., 2019, 68(2), 365–369, DOI:10.1093/sysbio/syy054.
- I. Letunic and P. Bork, Interactive Tree of Life (iTOL) v4: Recent updates and new developments, Nucleic Acids Res., 2019, 47(W1), 256–259, DOI:10.1093/nar/gkz239.
- Ministry of Economy Trade and Industry (METI) J., Deliberation Examination Sheet as to Whether or Not It Corresponds to a Class 1 Specified Chemical Substance or a Monitoring Chemical Substance (Siloxanes D4, D5, D&) Chromatography G. Standard Test Method for Analysis of Gases Dissolved in Electrical Insulating Oil by Methods, 2002, 05.
-
American Public Health Association (APHA), Standard Methods for the Examination of Water & Wastewater, American Public Health Association; American Water Works Association; Water Environment Federation, 21st edn, 2005 Search PubMed.
- C. Sánchez-Brunete, E. Miguel, B. Albero and J. L. Tadeo, Determination of cyclic and linear siloxanes in soil samples by ultrasonic-assisted extraction and gas chromatography-mass spectrometry, J. Chromatogr. A, 2010, 1217(45), 7024–7030, DOI:10.1016/j.chroma.2010.09.031.
- R. Dewil, L. Appels, J. Baeyens, A. Buczynska and L. Van Vaeck, The analysis of volatile siloxanes in waste activated sludge, Talanta, 2007, 74(1), 14–19, DOI:10.1016/j.talanta.2007.05.041.
- S. C. Popat and M. A. Deshusses, Biological removal of siloxanes from landfill and digester gases: Opportunities and challenges, Environ. Sci. Technol., 2008, 42(22), 8510–8515, DOI:10.1021/es801320w.
- J. Sanchís, E. Martínez, A. Ginebreda, M. Farré and D. Barceló, Occurrence of linear and cyclic volatile methylsiloxanes in wastewater, surface water and sediments from Catalonia, Sci. Total Environ., 2013, 443, 530–538, DOI:10.1016/j.scitotenv.2012.10.047.
- S. Varaprath, D. H. Stutts and G. E. Kozerski, A primer on the analytical aspects of silicones at trace levels-challenges and artifacts – A review, Silicon Chem., 2006, 3(1–2), 79–102, DOI:10.1007/s11201-006-9005-8.
- S. Achinas and G. J. W. Euverink, Theoretical analysis of biogas potential prediction from agricultural waste, Resour Technol., 2016, 2(3), 143–147, DOI:10.1016/j.reffit.2016.08.001.
- P. Jenicek, C. A. Celis, L. Krayzelova, N. Anferova and D. Pokorna, Improving products of anaerobic sludge digestion by microaeration, Water Sci. Technol., 2014, 69(4), 803–809, DOI:10.2166/wst.2013.779.
- J. Loughrin and N. Lovanh, Aeration to improve biogas production by recalcitrant feedstock, Environ – MDPI, 2019, 6(4), 4–13, DOI:10.3390/environments6040044.
- P. Jeníček, J. Horejš, L. Pokorná-Krayzelová, J. Bindzar and J. Bartáček, Simple biogas desulfurization by microaeration – Full scale experience, Anaerobe, 2017, 46, 41–45, DOI:10.1016/j.anaerobe.2017.01.002.
- S. Montalvo, S. Vielma, R. Borja, C. Huiliñir and L. Guerrero, Increase in biogas production in anaerobic sludge digestion by combining aerobic hydrolysis and addition of metallic wastes, Renewable Energy, 2018, 123, 541–548, DOI:10.1016/j.renene.2018.02.004.
- B. Zhang, X. Xu and L. Zhu, Structure and function of the microbial consortia of activated sludge in typical municipal wastewater treatment plants in winter, Sci. Rep., 2017, 7(1), 1–11, DOI:10.1038/s41598-017-17743-x.
- R. Camarillo and J. Rincón, Effect of inhibitory compounds on the anaerobic digestion performance of diluted wastewaters from the alimentary industry, J. Chem. Technol. Biotechnol., 2009, 84(11), 1615–1623, DOI:10.1002/jctb.2247.
- N. Kabelitz, P. M. Santos and H. J. Heipieper, Effect of aliphatic alcohols on growth and degree of saturation of membrane lipids in Acinetobacter calcoaceticus, FEMS Microbiol. Lett., 2003, 220(2), 223–227, DOI:10.1016/S0378-1097(03)00103-4.
- N. Togashi, A. Shiraishi and M. Nishizaka,
et al., Antibacterial Activity of Long-Chain
Fatty Alcohols against Staphylococcus aureus, Molecules, 2007, 12, 139–148 CrossRef CAS PubMed.
- K. Mukherjee, P. Tribedi, B. Mukhopadhyay and A. K. Sil, Antibacterial activity of long-chain fatty alcohols against mycobacteria, FEMS Microbiol. Lett., 2013, 338(2), 177–183, DOI:10.1111/1574-6968.12043.
- L. 0 Ingram and N. S. Vreeland, Differential Effects of Ethanol and Hexanol on the Escherichia coli Cell Envelopet, J. Bacteriol., 1980, 144(2), 481–488 CrossRef CAS PubMed.
- D. Nguyen, Z. Wu, S. Shrestha, P. H. Lee, L. Raskin and S. K. Khanal, Intermittent micro-aeration: New strategy to control volatile fatty acid accumulation in high organic loading anaerobic digestion, Water Res., 2019, 166, 115080, DOI:10.1016/j.watres.2019.115080.
- E. Boada, E. Santos-Clotas, S. Bertran, A. Cabrera-Codony, M. J. Martín, L. Bañeras and F. Gich, Potential use of Methylibium sp. as a biodegradation tool in organosilicon and volatile compounds removal for biogas upgrading, Chemosphere, 2020, 240, 124908, DOI:10.1016/j.chemosphere.2019.124908.
- D. G. Cirne, F. P. Van Der Zee, M. Fernandez-Polanco and F. Fernandez-Polanco, Control of sulphide during anaerobic treatment of S-containing wastewaters by adding limited amounts of oxygen or nitrate, Rev. Environ. Sci. Biotechnol., 2008, 7(2), 93–105, DOI:10.1007/s11157-008-9128-9.
- P. Tsapekos, P. G. Kougias, S. A. Vasileiou, G. Lyberatos and I. Angelidaki, Effect of micro-aeration and inoculum type on the biodegradation of lignocellulosic substrate, Bioresour. Technol., 2017, 225, 246–253, DOI:10.1016/j.biortech.2016.11.081.
- Z. Wu, D. Nguyen and T. Y. C. Lam,
et al., Synergistic association between cytochrome bd-encoded Proteiniphilum and reactive oxygen species (ROS)-scavenging methanogens in microaerobic-anaerobic digestion of lignocellulosic biomass, Water Res., 2021, 190, 116721, DOI:10.1016/j.watres.2020.116721.
- S. F. Fu, F. Wang, X. S. Shi and R. B. Guo, Impacts of microaeration on the anaerobic digestion of corn straw and the microbial community structure, Chem. Eng. J., 2016, 287, 523–528, DOI:10.1016/j.cej.2015.11.070.
- T. Niu, Z. Zhou and X. Shen,
et al., Effects of dissolved oxygen on performance and microbial community structure in a micro-aerobic hydrolysis sludge in situ reduction process, Water Res., 2016, 90, 369–377, DOI:10.1016/j.watres.2015.12.050.
- S. Xu, A. Selvam and J. W. C. Wong, Optimization of micro-aeration intensity in acidogenic reactor of a two-phase anaerobic digester treating food waste, J. Waste Manage., 2014, 34(2), 363–369, DOI:10.1016/j.wasman.2013.10.038.
- A. Cabrera-Codony, A. Georgi, R. Gonzalez-Olmos, H. Valdés and M. J. Martín, Zeolites as recyclable adsorbents/catalysts for biogas upgrading: Removal of octamethylcyclotetrasiloxane, Chem. Eng. J., 2017, 307, 820–827, DOI:10.1016/j.cej.2016.09.017.
- R. J. Watts, S. Kong, C. S. Haling, L. Gearhart, C. L. Frye and B. W. Vigon, Fate and effects of polydimethylsiloxanes on pilot and bench-top activated sludge reactors and anaerobic/aerobic digesters, Water Res., 1995, 29(10), 2405–2411, DOI:10.1016/0043-1354(95)00067-U.
- S. J. Traina, N. J. Fendinger, D. C. McAvoy, K. M. Kerr and S. Gupta, Fate of polydimethylsilicone in biosolids-amended field plots, J. Environ. Qual., 2002, 31(1), 247–255 CrossRef CAS PubMed.
-
Q. Zhang, J. Cui, W. Zhu, Y. Guo and X. Liu, Techniques for Siloxanes Removal from Biogas Produced by Sewage Sludge Digestion, in Int Conf Energy Dev Environ Prot, 2016, published online Search PubMed.
- D. Kino, K. Okada, Y. Tokudome, M. Takahashi, L. Malfatti and P. Innocenzi, Reactivity of silanol group on siloxane oligomers for designing molecular structure and surface wettability, J. Sol-Gel Sci. Technol., 2021, 97, 734–742, DOI:10.1007/s10971-020-05448-z.
- C. Y. Li, J. H. Chen, P. C. Chien, W. Y. Chiu, R. S. Chen and T. M. Don, Preparation of poly(IPDI-PTMO-siloxanes) and influence of siloxane structure on reactivity and mechanical properties, Polym. Eng. Sci., 2007, 47(5), 625–632, DOI:10.1002/pen.20734.
- C. S. Harwood, G. Burchhardt, H. Herrmann and G. Fuchs, Anaerobic metabolism of aromatic compounds via the benzoyl-CoA pathway, FEMS Microbiol. Rev., 1998, 22(5), 439–458, DOI:10.1111/j.1574-6976.1998.tb00380.x.
-
H. M. Alvarez, in Central Metabolism of Species of the Genus Rhodococcus, Springer, Berlin, Heidelberg, 2010, pp. 91–108, DOI:10.1007/978-3-642-12937-7_4.
- M. X. Wang, G. Lu, G. J. Ji, Z. T. Huang, O. Meth-Cohn and J. Colby, Enantioselective biotransformations of racemic α-substituted phenylacetonitriles and phenylacetamides using Rhodococcus sp. AJ270, Tetrahedron: Asymmetry, 2000, 11(5), 1123–1135, DOI:10.1016/S0957-4166(00)00025-2.
Footnote |
† Electronic supplementary information (ESI) available. See DOI: 10.1039/d1em00143d |
|
This journal is © The Royal Society of Chemistry 2021 |