The fate of poly- and perfluoroalkyl substances in a marine food web influenced by land-based sources in the Norwegian Arctic†
Received
14th December 2020
, Accepted 28th February 2021
First published on 11th March 2021
Abstract
Although poly- and perfluorinated alkyl substances (PFAS) are ubiquitous in the Arctic, their sources and fate in Arctic marine environments remain unclear. Herein, abiotic media (water, snow, and sediment) and biotic media (plankton, benthic organisms, fish, crab, and glaucous gull) were sampled to study PFAS uptake and fate in the marine food web of an Arctic Fjord in the vicinity of Longyearbyen (Svalbard, Norwegian Arctic). Samples were collected from locations impacted by a firefighting training site (FFTS) and a landfill as well as from a reference site. Mean
concentration in the landfill leachate was 643 ± 84 ng L−1, while it was 365 ± 8.0 ng L−1 in a freshwater pond and 57 ± 4.0 ng L−1 in a creek in the vicinity of the FFTS. These levels were an order of magnitude higher than in coastal seawater of the nearby fjord (maximum level
, at the FFTS impacted site). PFOS was the most predominant compound in all seawater samples and in freshly fallen snow (63–93% of
). In freshwater samples from the Longyear river and the reference site, PFCA ≤ C9 were the predominant PFAS (37–59%), indicating that both local point sources and diffuse sources contributed to the exposure of the marine food web in the fjord.
concentrations increased from zooplankton (1.1 ± 0.32 μg kg−1 ww) to polychaete (2.8 ± 0.80 μg kg−1 ww), crab (2.9 ± 0.70 μg kg−1 ww whole-body), fish liver (5.4 ± 0.87 μg kg−1 ww), and gull liver (62.2 ± 11.2 μg kg−1). PFAS profiles changed with increasing trophic level from a large contribution of 6:2 FTS, FOSA and long-chained PFCA in zooplankton and polychaetes to being dominated by linear PFOS in fish and gull liver. The PFOS isomer profile (branched versus linear) in the active FFTS and landfill was similar to historical ECF PFOS. A similar isomer profile was observed in seawater, indicating major contribution from local sources. However, a PFOS isomer profile enriched by the linear isomer was observed in other media (sediment and biota). Substitutes for PFOS, namely 6:2 FTS and PFBS, showed bioaccumulation potential in marine invertebrates. However, these compounds were not found in organisms at higher trophic levels.
Environmental significance
The distribution of poly- and perfluorinated alkyl substances (PFAS) was investigated in a marine food web of a Norwegian Arctic fjord. Local point sources (firefighting station and landfill) and diffuse sources contributed to the exposure of the Svalbard marine food web. PFOS substitutes, namely 6:2 FTS and PFBS showed a bioaccumulation potential in marine invertebrates. This study indicates that local sources should be considered in the assessment of PFAS exposure in the Arctic environment and focus should not only be on their long range transport.
|
Introduction
The presence of poly- and perfluoroalkyl substances (PFAS) in the environment has attracted significant attention and research during the two last decades.1,2 PFAS are a group of man-made chemicals and are classified and subdivided based on their characteristic functional groups. The most commonly studied PFAS groups include perfluoroalkyl carboxylates (PFCA) and perfluoroalkane sulfonates (PFSA), fluorotelomer alcohols (FTOH), sulfonamido ethanols (FOSE), and fluorotelomer sulfonates (FTS).3 Several PFAS are regulated nationally and/or internationally through the Stockholm Convention (http://www.pops.int) and their use has been, or is currently being phased out. However, they have been replaced by other substitute PFAS, which are of unknown environmental concern.4 Perfluorooctane sulfonate (PFOS) is one of the most widely known PFSA being detected worldwide in the aquatic and the terrestrial environment, including humans.5,6 PFOS and its precursors were only manufactured with electrochemical fluorination (ECF) which yields a mixture of linear and branched isomers with known percentages (70 ± 1.1% and 30 ± 0.8%, respectively).7,8
The extremely broad product application range for PFAS has resulted in the ubiquitous detection of these persistent chemicals, even in remote environments such as the Arctic.9,10 PFAS are considered priority chemicals of emerging concern for the Arctic.11 The transport pathways that result in PFAS ultimately ending up in the Arctic ecosystem is a focus of current research. The most frequently used PFAS are amphiphobic and ionic, and hence, not expected to be prone to long-range atmospheric transport.12 Oceanic long-range transport is a known transport pathway for ionic PFAS.13 However, the observation of the occurrence of neutral precursors in outdoor air14–17 suggests that precursors with long atmospheric lifetimes have the potential to be transported over long distances and subsequently degraded in the atmosphere to environmental stable perfluoroalkyl acids (PFAA).18 Once PFAA are formed in the atmosphere, they deposit to the surface through wet or dry deposition.19 Degradation of these precursor compounds (e.g. fluorotelomer alcohols and polyfluorinated sulfonamides based chemicals) may increase environmental loads as it leads to the formation of PFAA.20,21 In addition, recent field and laboratory studies have suggested water-to-air transfer of PFAA through sea spray aerosol as an important additional source of PFAAs to the atmosphere.22,23
High concentrations of PFAS have been reported in Arctic environments influenced by local sources such as landfills, sewage discharge and airports.9,24 The use of aqueous film forming foams (AFFF) for firefighting training activities at airports has previously been noted to be a significant point source of PFAS to a variety of environmental media.4,25 The disposal of PFAS containing consumer products (food wrappings, non-stick cook ware, stain-resistant coatings, cleaning products, etc.) has also resulted in elevated PFAS levels in landfill leachate.26–28 In the Svalbard archipelago, further studies are needed to elucidate the contribution of such local sources to the Arctic environment as well as how these sources affect the marine food web. Further, the direct link between the release from local sources and accumulation in the Arctic marine environment has not been studied previously.
Hence, the main objective of the present study is to investigate the fate of PFAS released by certain point sources in a marine food web in the Norwegian Arctic. Thus, the PFAS distribution patterns in terrestrial, limnic and marine abiotic matrices (water, snow and sediments) and biota at various trophic levels in the marine food web was investigated.
Materials and methods
The study sites
Longyearbyen is the largest settlement on Svalbard, with approximately 2400 inhabitants.29 During the tourist season, up to 100
000 visitors, arriving by cruise ship or plane at the small local airport are recorded each year.30 Following the cessation of most coal mining activities in 2018, both tourism and education drive the local economy. The mean temperature varies from −16 °C in February to +6 °C in July, and the annual precipitation is approximately 200 mm (Norwegian Meteorological Institute). The following point sources were included in this study (Fig. 1), representing the main point sources of the study area: Svalbard Airport (N 78°14′, E 15°30′), situated approximately five kilometres northwest of Longyearbyen centre and a decommissioned landfill in Adventdalen (N 78°10′, E 15°56′). Diffuse sources to the marine environment include wastewater from the municipality and the airport which is discharged without pre-treatment into the Adventfjord, at approximately 60 m depth 2 km off the coast,31 and runoff from the municipality. It was estimated that Longyearbyen city annually releases about 285
000 m3 of untreated wastewater into the Adventfjord.32 To investigate the PFAS load from diffuse sources, the Longyear river (N 78°13′, E 15°38′) which runs through Longyearbyen and is by glacier and snow melt, was sampled, as well as a meltwater creek (N 78°12′, E 15°12′), which is fed by snow melt. A snow sample, collected directly after a precipitation event, was sampled from a nearby mountain side, Breinosa (N 78°09′, E 16°03′), which could represent PFAS from atmospheric deposition.
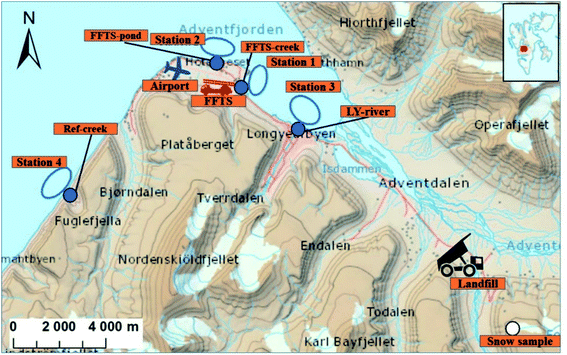 |
| Fig. 1 Marine sampling stations (blue circles representing St1–4), sampling points for freshwater samples (blue dots for Ref-creek, FFTS-pond, FFTS-creek, LY-river), the landfill, and snow sample (white dot) locations in the vicinity of Longyearbyen (Svalbard, Norway, source: http://toposvalbard.npolar.no). | |
Svalbard airport was opened in 1975 and has two firefighting training stations (FFTS), one decommissioned area north-east of the runway and one newer active training area south-east of the runway (Fig. 1). The main source of contamination at the airport site is AFFF containing PFAS used during training which is assumed to have been transported with run-off to the Adventfjord during the short spring snow melting season. The landfill received municipal and industrial waste between 1991 and 2007. From 2007, most municipal waste from Longyearbyen was transported to mainland Norway for incineration and mainly non-degradable waste (e.g. gypsum, steel, concrete and slag) has been disposed of at the landfill.33
Water samples
To investigate the contribution of PFAS to the marine food web from the active FFTS, runoff water from a creek running from the FFTS to the coast was collected in duplicate using 2.5 L methanol rinsed polyethylene bottles (FFTS-creek, Fig. 1). Water from the pond down gradient of the old FFTS, receiving runoff from the airport, was also collected (FFTS-pond, Fig. 1). At the landfill site, leachate water was sampled (landfill). To investigate the contribution from the various sources, seawater was sampled from four representative stations located in the fjord system (the Adventfjord and Isfjord, St1–St3, Fig. 1), and one reference location (St4, Fig. 1). All of these water samples were collected in June 2018. From each of the marine sampling stations in the fjord, surface (1 m below surface), subsurface (mid water column) and deep seawater (1 m above the seabed) were sampled using a Ruttner Water Sampler (KC Denmark A/S). Seawater samples were analysed without filtration thus representing total water concentrations. Station 1 is impacted by the active FFTS and located close to where the creek drains into the fjord (N 78°14′, E 15°33′), while station 2 is impacted by the old FFTS site (FFTS-pond) and it receives general runoff from the airport (N 78°15′, E 15°29′). Station 3 is located directly outside the Longyearbyen settlement, where the Longyearbyen river flows into the fjord (N 78°14′, E 15°39′). Station 3 is also affected by water from Adventdalen, where the landfill is located. The reference station, station 4 is located in the fjord, Isfjord, approximately 10 km from any known PFAS source (airport, landfill or settlement). This station was chosen as a background site that reflects the coastal waters of the fjord. However, it cannot be excluded that this site may be affected by these sources. Runoff from a small meltwater creek draining into the Isfjord at station 4 was sampled to represent PFAS from atmospheric deposition (Ref-creek, N 78°12′, E 15°12′). The Longyear river is a meltwater river receiving meltwater from the adjacent glaciers (Longyearbreen and Larsbreen glaciers). This was sampled to represent atmospheric deposition and contamination from Longyearbyen town before draining into the Adventfjord (LY-river, N 78°13′, E 15°38′). A surface snow sample was collected on the mountainside above the active coal mine (snow sample, N 78°09′, E 16°03′, 545 above mean sea level, Fig. 1) in October 2018. Surface snow (0–10 cm depth) was collected following recent precipitation during the previous 7 days and so it presumably represents newly deposited PFAS. The snow was melted and analyzed as an aqueous sample. Sampling data are presented in Table S1 and Fig. S1.†
Sediment and biota samples
Bottom sediments (0–5 cm depth) were collected in triplicate at the four marine stations (St1–St4) using a van Veen grab sampler. Sediment from the upper centimetres of the landfill leachate drainage channel was also collected. Marine biota samples were collected at the four marine stations (St1–St4). To determine PFAS levels in benthic organisms, polychaetes were collected (approximately 10 g from each station) from the sediments sampled and individuals from the same station were pooled into one representative sample (Table S2†). Polychaetes were depurated overnight in seawater in order to separate sediment-bound PFAS from accumulated PFAS. Pelagic zooplankton (copepods, mainly Calanus spp.) was collected and triplicate samples from each station were pooled for analyses (approximately 20 g per station, Table S2†). One to 14 crabs (Hyas araneus) were collected from each station and one to seven individuals were analysed (Table S3†). Two local fish species were collected: sculpin (Myoxocephalus scorpius) (n = 29) and wolffish (Anarhichas lupus) (n = 3) from stations St1, St2 and St4. Liver and muscle samples were obtained from each fish individually and analysed separately (see Table S4 and Fig. S2† for fish and liver weights). Twenty glaucous gulls specimen (Larus hyperboreus) were sampled in the proximity of Svalbard airport at Adventpynten (between station 1 and 2) in April 2018 and liver samples were obtained (biological parameters of the collected glaucous gulls are shown in Table S5†). None of the investigated biota species are threatened according to the IUCN Red List Categories. The sampling was performed in accordance with the Norwegian animal welfare act and national regulations.
PFAS levels in biota are calculated on a wet weight basis (ww), while concentrations in sediment are given on dry weight basis (dw) due to the potential variability in moisture content.
Sample preparation and HPLC−MS/MS analysis
Two previously published analytical methods were adopted with some modification for the analysis of abiotic and biotic samples.34,35 The methods were subjected to a comprehensive validation before being applied for the simultaneous quantification of all the selected PFAS (see Table S6†). A detailed description of the methods is available in the ESI.† Briefly, sediment and biota samples were extracted with methanol. Clean-up of methanol extracts was conducted using active carbon (EnviCarb, Sigma-Aldrich Co., PA, USA). Water and melted snow samples were extracted on Oasis® Waters (Mildford, MA, USA) weak-anion exchange (WAX) SPE cartridges (6 mL volume, 0.5 g). The quantitative determination of PFAS was done with high-performance liquid chromatography (HPLC) using an Agilent 1200 series HPLC (Agilent Technologies, Waldbronn, Germany) and an Agilent 6460 (Agilent Technologies, Santa Clara, CA, USA) triple quadrupole mass spectrometer equipped with a jet stream electrospray ion source.
Quality assurance, quality control, and method validation
All samples were analysed under standardized conditions (NS-EN ISO/IEC 17025 – TEST 137) and spiked with a mixture of 12 internal standards (ISTDs) (see Tables S7 and S8†) before extraction. In order to monitor contamination during transportation and sample preparation, field and laboratory blank samples made of Milli-Q water (for water samples) and sodium sulfate standard - 99.99% (for sediment, and each organism type) were included and processed as real samples. Potential contamination resulting from the HPLC system was avoided by using a delay column (Agilent Eclipse Plus C18, 4.6 × 50 mm, 3.5 μm), installed after the mixing valve, and before the autosampler.36 This helps to resolve problems related to PFAS that originate from the instrumental contamination, as depicted in Fig. S4.† Additionally, a methanol blank was injected after every 10 samples. None of the targeted PFAS were detected in the methanol blanks, indicating the absence of carryover effects. Instrument limit of detection (LOD) and lower limit of quantification (LOQ) were determined by the compound specific amount corresponding to a signal to noise ratio (S/N) = 3 (LOD) and 10 (LOQ). These calculations were based on the three lowest calibrations standards prepared in solvent (0.05, 0.1, 5 pg μL−1). For compounds not detected in procedural blank samples, the method detection limit (MDL) was determined as the concentration resulting in S/N = 3, based on the three lowest calibrations standards (0.1, 0.5, 5 pg μL−1) prepared in real sample extracts. MDLs for compounds detected in procedural blank samples were determined as C + 3SD, where C is the mean concentration measured in blanks and SD is the standard deviation. No blank correction was made for these compounds. Signals detected below LOD were presented as non-detected (nd), while levels detected above LOD but below the calculated MDL, were reported as <LOQ (see Table S9†).
In addition to the 19 PFAS targeted in this study, the proportion of total branched PFOS isomers were quantified. All target PFAS were quantified using internal standard calibration curves with eight concentration points (R2 > 0.99). For seawater, standards including both native and internal standards were prepared in similar matrix extracts. Samples with minimal PFAS concentrations were used for matrix matched calibration (see the ESI for details, Table S10†) which has resulted in better recovery. In sediment and biota matrices, matrix-matched calibration remained necessary for the quantification of 6:2 FTS, which showed unacceptable recoveries > 140% which was attributed to a lack of exactly-matched, isotopically-labelled ISTD. However, due to the lack of PFAS free biota material, and the observed low salinity in meltwater samples, these matrices were analysed with solvent matched calibration for PFAS other than 6:2 FTS. The proportion of total branched PFOS isomers was calculated using the chromatographic peak area against the calibration curve of the linear PFOS isomer.37,38 For this, concentrations were calculated using the average of m/z 499/80 and 499/99 ions for both PFOS isomers, as described in Riddell et al.39 However, in order to enhance the selectivity, 499/99 ion was selected for PFOS quantification in fish and gull livers samples due to endogenous interferences associated with the m/z 499 → 80 transition.40 For each sample type, matrix spiked apparent recovery percentages of all target PFAS were calculated from samples with low-contamination levels (4–6 replicates) spiked at two concentration levels (1.0 and 25 μg kg−1 for sediment and biota; 3.0 and 25 ng L−1 for water). Most target PFAS showed acceptable recoveries (40–125%, Table S11†). Additionally, relative recoveries of internal standards were also calculated based on their linear calibration curves applying [13C8]-PFOA as a recovery standard (see Table S12†). N-Methyl perfluorooctanesulfonamido ethanol (N-MeFOSE), N-methyl perfluorooctanesulfonamide (N-MeFOSA), N-ethyl perfluorooctanesulfonamido ethanol (N-EtFOSE), and N-ethyl perfluorooctanesulfonamide (N-EtFOSA) showed unacceptable low recoveries for several matrices, and consequently were excluded from the dataset. PFBA was excluded from quantification due to some concerns of interference affecting the results which could not be excluded with only one MRM transition. Therefore, 14 PFAS (and Br-PFOS) were quantified. Analyte names, acronyms, CAS numbers, and structures of the 14 target compounds are shown in Table S6.†
Statistics and data handling
Principal component analysis (PCA) was performed to investigate the main patterns of variation in PFAS profiles within the dataset after a normalization to sum PFAS concentrations. For PCA, the R-software (R-Studio Version 1.1.143 based on R version 3.5.2.) was used under the GNU public license (Boston, MA, USA) with prcomp function and the package ggbiplot. The non-parametric unpaired Wilcoxon Test/Mann–Whitney U test was applied for testing the differences in PFAS concentrations between FFTS-impacted sites and the background reference site and between female and male crabs and glaucous gull individuals. The Spearman's correlation test was used for testing the correlation between
concentrations and biological parameters for individual organisms and to investigate the correlation among individual PFAS. The significance threshold was set to p < 0.05. Values reported in the current paper, indicate average values and ±standard error of the mean (SEM). For compounds detected at concentrations < LOQ, values were set at half LOQ for the summation of
.
Results and discussion
Concentration and distribution patterns of PFAS in water: point sources
PFAS concentration and distributions patterns for the 14 target PFAS are shown in Fig. 2 and PFAS concentrations in all water samples are listed in Table S13.† The highest
concentrations were detected in the landfill leachate (643 ± 84 ng L−1). These
levels were similar to the concentrations reported in the leachate of Norwegian landfills (median 630 ng L−1) and in Spain (639–1379 ng L−1).33,41 Higher values have been found in landfill leachates in USA (2000 to 29
000 ng L−1) and Australia (2000 to 15
000 ng L−1).27,41,42 Water samples collected from the pond that receives drainage water from the Svalbard airport (FFTS-pond) and a creek downstream from the FFTS (FFTS-creek) also showed elevated
concentrations (365 ± 8.0 and 57.4 ± 4.0 ng L−1, respectively). This is in agreement with a recent study conducted in Longyearbyen where Skaar et al.35 reported high
concentrations in run-off water samples collected in June 2015 at 600 m downstream of the local FFTS at Svalbard airport in Longyearbyen (113 ± 2.9 ng L−1).
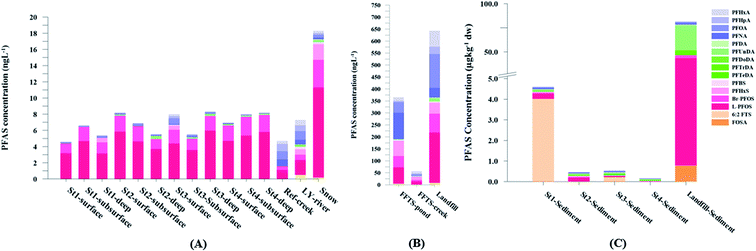 |
| Fig. 2 Distribution and average concentrations of PFAS in abiotic samples in the vicinity of Longyearbyen (Svalbard). (A) and (B) Aqueous samples from the fresh water and marine environment (standard error of the mean is given in Table S13†). (C) Marine sediment samples (St1–4) and landfill sediments (n = 3 at each station, standard error of the mean is given in Table S14†). (Levels < LOQ were treated as zero in this figure.) | |
The predominant PFAS in the FFTS-creek, which receives runoff from the firefighting training area where AFFF is actively used, were PFOS (35% of
), PFHxS (22%), PFHxA (18%), PFOA (11%), PFHpA (6%) and smaller percentages of the remaining compounds (≤3% per compound). The occurrence of 6:2 FTS at 1.46 ± 0.08 ng L−1 (2.5% of
) might indicate the use of new AFFF formulations at the FFTS, as 6:2 FTS and related compounds have replaced PFOS after the phase-out in the 2000s according to the Norwegian Aviation Organisation.43 A similar profile was identified in the FFTS-pond which is close to the old firefighting training area and receives general runoff from the airport (without runoff from the active FFTS). The leachate water from the decommissioned Longyearbyen landfill was characterized by a high relative contribution of PFCA C6–11 (43% of
of which PFOA accounts for approximately 20%) and the sum of linear and branched PFOS representing 48% of
. The formation of PFCA e.g. PFHxA and PFOA from the degradation of fluorotelomers, precursors to PFCA, can be a potential source for PFCA in the landfill leachate.44 A similar PFAS pattern is reported for landfill leachate from Spain, where PFOA was the predominant compound at 43% of the total PFAS.41
Concentration and distribution patterns of PFAS in water: diffuse sources
The
concentrations in samples from LY-river, Ref-creek, the snow sample as well as in the seawater samples from the Adventfjord were lower than the concentrations reported in freshwater at the landfill and the FFTS pond (Fig. 2 and Table S13†). In the LY-river sample, the predominant PFAS were PFHxA, PFHpA, PFOA, PFHxS, and PFOS. The concentrations of PFCA C6–12 in LY-river reported in the current study (6.44 ng L−1) were higher than previously reported (3.51 ng L−1)45 (Table S13†). This could be related to the season and the water-flow in the river, where the previously reported samples were taken during May 2006.
In the Ref-creek sample, a similar concentration as in the LY-river was found and the predominant PFAS were PFOA and PFOS (19 and 20% respectively). The
in the snow sample from the mountain side was somewhat higher (18.70 ng L−1, Table S13†), and was dominated by PFHxS (17%) and PFOS (64%). The PFOA concentration in the snow sample (0.360 ± 0.007 ng L−1, Table S13†) was similar to a previous study in which snow was sampled near Longyearbyen town (0.396 ± 0.161 ng L−1).45 However, ∑PFAS (1.47 ng L−1) and PFOS (0.118 ± 0.052 ng L−1) concentrations were much lower in that particular study.45
In the seawater samples, PFOS was the most dominant compound (Fig. 2, Table S13†). Depth profiles in the fjord based on the three sampling levels (surface, subsurface, and bottom waters) showed that PFAS were detected throughout the water column of the Adventfjord (St1–St4). At station 3 and station 4, which was the reference station,
concentrations increased with depth, indicating a PFAS contribution from the deep marine water in contact with the bottom sediments. In contrast, in station 2, which receives direct runoff from the airport,
decreased with depth, while in station 1 (impacted by the active FFTS) the highest
value was found in the mid water column. In a previous study where surface water samples were collected in the coastal zone just outside Longyearbyen in the Adventfjord during May 2006 the
concentrations were 0.73 ng L−1,45 which is lower than reported herein. Although this may indicate a temporal increase in PFAS levels in the Adventfjord, the differing concentrations may also be due to seasonal variations in runoff from the point-sources caused by snow-melting and/or precipitation events. Nevertheless, the PFAS concentrations in the Adventfjord are higher than those previously reported for the open North Sea and Norwegian Sea (0.01–0.07 ng L−1),46 indicating that the local point-sources contribute to the levels of PFAS in the Adventfjord.
Contribution of different PFAS sources to water pollution
The differences in the PFAS distribution patterns between water samples were considered to be indicative of the different input from the different PFAS sources. A principal component analysis (PCA), using PFAS profiles, i.e. individual PFAS are expressed as percentages of the
, was used to investigate groupings between sample locations (Fig. S5†). The PCA revealed that the water samples were distributed into five distinct groups: (1) marine water samples, (2) the snow sample, (3) LY-river, (4) FFTS-pond, FFTS-creek, and (5) landfill leachate and Ref-creek.
Concentrations of PFHxA, PFBS and PFOS were 1.7 to 5 times higher in the LY river compared to Ref-creek. The samples from LY-river represent glacial meltwater as well as run-off from the town of Longyearbyen, whereas the Ref-creek sample represents meltwater from the annual snowpack. PFBS is known as a major contaminant in wastewater effluents.47 Thus, a significant local source of PFAS originating from the Longyearbyen settlement has most likely resulted in the elevated PFAS concentrations in the downstream part of the LY-river.45 In contrast, PFHpA and PFNA were detected at higher concentrations in the Ref-creek than in the LY-river, which might indicate that their source is more due to atmospheric transport than a local source. Previously, PFOA and long-chain PFCA were detected on particles collected from the Arctic atmosphere.9 Due to their limited commercial production,48 the presence of long chain PFCA with C ≥ 10 in the river and the snow, points towards long range transport and atmospheric oxidation of PFAS precursors to terminal end products, and their subsequent atmospheric deposition.14,21 However, these compounds were detected at LOQ concentrations as depicted in Table S13.†
The concentration ratio of PFOA to PFNA (C8
:
C9) in Ref-creek was 1.2 ± 0.2, whereas it was 1.9 ± 0.1 in the LY-river sample. Ratios observed in an Arctic ice core, which was presumed to receive input solely from the atmosphere degradation of precursors were 1.5 ± 0.8.21 Further study of remote Arctic ice cores found that PFCA molar ratios of even–odd pairs were typically less than 2 and and greater than 0.5.49 This is close to the ratio of C8
:
C9 in the snow sample in this study (1.63 ± 0.04). Although this is inline with the C8
:
C9 ratios from remote Arctic locations, further snow sampling is required to understand if this as a result of atmospheric precursor degradation at this site, given its proximity to known sources.
Possible sources of PFAS in snow could include marine aerosols,50 direct local contamination35 and long range transport of PFAS precursors and, their subsequent degradation and deposition.21 Previous studies in Longyearbyen concluded that direct local inputs45 were more important than inputs from the atmospheric degradation of precursors or marine aerosols.
The concentration of L-PFOS (6.07 ng L−1, 54.7% of
) in the snow sample in this study was significantly higher than PFOS previously reported in snow and ice cores at remote sites in Svalbard and the wider Arctic.35 This suggests a significant local source, such as from firefighting training at the active coal mine (1.3 km from the sampling site), or from known local PFAS sources such as the FFTS (16.1 km).
Concentration and distribution patterns of PFAS in sediment
Fig. 2 shows the concentration of PFAS in the sediments sampled at the landfill leachate channel and the Adventfjord. All individual concentrations are listed in Table S14.† Concentrations of
were higher in the sediment from the landfill (81.65 ± 2.13 μg kg−1) than in sediment from the fjord (maximum
) (Fig. 1 and Table S14†), reflecting, similar to in the water samples, the difference in PFAS input. In sediment collected from the landfill, ∑PFOS (average concentration 45.4 ± 1.54 μg kg−1) contributed 55% and PFUnDA contributed 31% to
(Table S14†). The high concentrations of PFAS in the sediment from the landfill are likely due to a combination of settling leachate particles as well as sorption of PFAS to the peat that dominated sediments at the landfill site.51 It has previously been reported that FTOH in sediments can be biodegraded to PFCA44 and this may explain the presence of long chain PFCAs in sediment samples of the landfill (PFCA C10, C11, C12, C13 and C12 at 0.86, 25.5, 0.69, 4.21, and 0.04 μg kg−1 respectively) given that these compounds only have a limited number of direct applications in products. It is worth mentioning that PFAS emission from this landfill is considered low as the cold climate of Svalbard limits the volume of leachate production which has been estimated as 25
000 m3 per year.33
Concentrations in the marine sediments from the Adventfjord were generally low (Fig. 3 and Table S14†). However, the
in the marine sediment samples collected in the vicinity of FFTS influenced sites (St1 and St2;
) were significantly higher (Mann–Whitney-U-test, p < 0.02) than
in sediment samples from the reference station
(Fig. 2 and Table S14†). This confirms that there is a contribution from local sources to levels of PFAS observed in the marine sediments. 6:2 FTS was detected in all samples collected from station St1 (influenced by the active FFTS) at an average concentration of 4.0 μg kg−1. 6:2 FTS was the most predominant compound (86% of
) at this station followed by PFOS (10%). PFOS was the most dominant compound in sediment samples collected from St2 (influenced by airport runoff), accounting for 45% of
. This indicates that this station was influenced by the FFTS. Long chain PFCAs (C8–C14) are the most predominant compounds (69% of
) in sediment collected from the background station, St4, followed by the PFBS (18%) and PFOS (7%), indicating at least a different source.
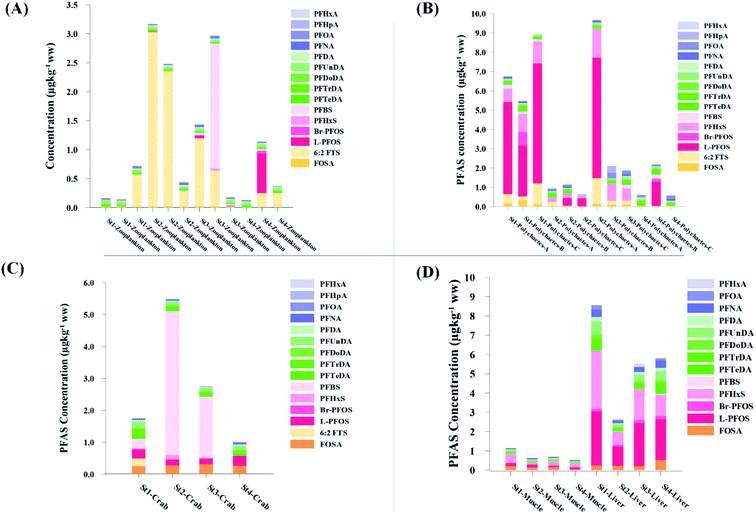 |
| Fig. 3 Average concentration of PFAS (μg kg−1 ww) detected in biota samples in the vicinity of Longyearbyen (Svalbard); (A) zooplankton; (B) polychaetes; (C) local crab samples (standard error of the mean is given in Table S17†); (D) local fish samples (muscle and liver) collected in the vicinity of Longyearbyen (Svalbard, standard error of the mean is given in Tables S19 and S20†). | |
Concentration and distribution patterns of PFAS in pelagic marine biota – zooplankton
Fig. 3 shows the PFAS concentrations (μg kg−1 ww) in zooplankton (dominated by Calanus spp.) collected from St1–St4. PFAS were quantified in zooplankton at low concentrations (0.342–2.03 μg kg−1 ww). This can be attributed to the low levels of PFAS observed in the water column. Long chain PFCA (C8–C13) dominated the profiles at St1 (67% of
) and St4 (48% of
) with a maximum concentration observed for PFUnDA (0.045 μg kg−1 ww). 6:2 FTS dominated the profile at St2 which is directly impacted by FFTS emissions (accounting for 82% of
, 1.9 μg kg−1 ww). 6:2 FTS was the second most predominant PFAS in St1 which is also impacted by FFTS emissions (accounting for 26% of
, 0.19 μg kg−1 ww). The occurrence of 6:2 FTS in nine of the twelve zooplankton samples confirms its bioaccumulation potential which has been reported recently for invertebrates near a military airport.25 Although neither PFHxA, nor PFHpA, were detected in any zooplankton samples, the short chain PFAS, PFBS was detected in four of the twelve zooplankton samples investigated at a maximum concentration of 0.735 μg kg−1 ww, confirming that PFSA are more bioaccumulative than PFCA.52 Studies reporting PFAS concentrations in zooplankton in the Arctic are sparse in the scientific literature. PFOS was found at similar concentrations in zooplankton collected in the Baltic Sea 0.10 ± 0.02 μg kg−1 ww.53 A higher concentration range has been reported for PFOS in zooplankton from the Canadian Arctic, 1.1–2.6 μg kg−1 ww.54
Zooplankton plays an important role in the marine Arctic food web by transferring energy and carbon based nutrients from the primary producers (phytoplankton) to higher trophic levels.55 Therefore, the bioconcentration of PFAS in zooplankton found in this study indicates an important exposure route of the marine ecosystem.
Concentration and distribution patterns of PFAS in benthic invertebrates – polychaetes
Unlike pelagic organisms, benthic organisms live in direct contact with the sediments, and therefore have the potential to scrape, tear, and filter sediment.56,57 Ingesting sediments and absorbing the released PFAS represent their main exposure pathways.58 Polychaetes are representatives of the local benthic marine ecosystems and are expected to be indicators of the local PFAS exposure due to the relative immobility of polychaete species.59
in pooled polychaetes samples collected from the sediment samples was found in the range of 0.90 to 7.0 μg kg−1 ww (Fig. 3 and Table S17†). Similar to in the marine sediments, the maximum average
was observed in samples collected from the FFTS impacted station St1 (7.0 ± 0.95 μg kg−1) and the minimum
was observed in samples collected from the reference station (St4, 1.1 ± 1.7 μg kg−1). However, this difference was not found to be statistically significant (p > 0.05). PFOS was the predominant PFAS in most polychaete samples accounting for 22% (St4) to 67% (St1) of the
. Long chain PFCA (C9–14), PFOS, and FOSA were detected in all polychaete samples from all stations. 6:2 FTS was also detected in three of the four samples at low concentrations (<LOQ – 0.60 μg kg−1 ww) with the exception of the reference station (St4). On average, 6:2 FTS occurs at the highest concentration in the active FFTS influenced station St1 (0.60 μg kg−1 ww), followed by station St3 (0.58 μg kg−1 ww). The detection of 6:2 FTS in polychaetes and sediment might indicate that sediment is a potential source for this PFAS in the marine ecosystem. In a previous study 6:2 FTS was detected in benthic invertebrates collected from the Canadian High Arctic at 0.43 ± 0.74 μg kg−1 ww.60 Much higher concentrations (up to 630 mg kg−1 ww) were measured in earthworms collected from a AFFF impacted site at a major Canadian Airport.61 Therefore the present study confirms the bioaccumulation potential of 6:2 FTS in invertebrates. PFHpA was detected at St 2 at concentrations of 0.12 μg kg−1 ww. Since PFHpA was not detected in sediment samples, this might indicate that this short chain PFCA is a biotransformation product of PFAS precursors. Ruus et al.62 also reported PFAS at the same concentration range in polychaetes collected from the densely populated Oslo Fjord (0.1 to 1.6 μg kg−1 ww). The levels of PFAS found in the current study were, however, considerably lower than those measured by Lescord et al. 201560 in Canadian benthic invertebrates in Arctic fresh water lakes influenced by AFFF from airport activities (12–466 μg kg−1 ww).
Concentration and distribution patterns of PFAS in crabs
Concentrations of PFAS determined in 18 samples (whole body) of great spider crab (Hyas Araneus) (body weight = 76 ± 8 g) collected from the four stations are shown in Table S18† and Fig. 3. PFCA (C6,7,12) were not detected in any crab samples, indicating that these compounds were not enriched in crab tissues at detectable concentrations. In general, somewhat higher PFAS levels in crabs collected from contaminated sites (stations St1–3,
= 3.75 ± 0.77 μg kg−1 ww, p = 0.059) 1.28 ± 0.95 were found compared to the reference station (St4,
= 1.28 ± 0.95 μg kg−1 ww). Long chain PFCA (C8–11,13,14), FOSA, and PFOS were the predominant compounds in local crab samples with average percentage contributions to
of 28, 18, and 15%, respectively. However, PFBS dominated the profile of crab samples collected from the contaminated stations (St1–3) with average percentage contributions to
of 33% but was not detected in any crab sample collected from the reference station (St4, n = 7).
PFHxS was found in a single crab collected at St2 at 0.14 μg kg−1 ww. FOSA was detected in all individuals collected from all stations at trace concentration ranging from 0.1 to 0.4 μg kg−1 ww. 6:2 FTS was quantified in only two crab individuals collected from station St1 at an average concentration of 0.66 ± 0.43 μg kg−1 ww. A comparison between PFAS concentrations detected in crab samples from FFTS influenced sites (St1 and St2) and in those collected at the reference site (St4) is shown in Fig. 6.
in FFTS contaminated crabs was significantly higher than in individuals from the reference site. This clearly indicates the contribution of the local sources to the levels of PFAS in crabs.
No correlation was observed between the biological parameters of crab individuals (size and weight, data not shown) and the concentrations of
. Similarly, no correlation was observed between crab sex and
levels. However, the highest
(9.5 μg kg−1) was observed in a female individual collected from the FFTS impacted station (St2), and the lowest
(0.37 μg kg−1) was observed in a male crab collected from the reference station (St4).
Previous studies reporting the concentration of PFAS in crabs are limited. In general, levels of PFAS determined here were in the lower range compared to previously reported levels for a military airport in Norway.25 Langberg et al.25 reported average levels of 5.50 ± 0.80 and 3.92 ± 0.79 μg kg−1 ww for PFOS in green shore crab (Carcinus maenas) and great spider crab respectively, collected at a military airport in Norway. These levels are higher than PFOS levels found in the current study (average 0.28 ± 0.04 μg kg−1 ww). Similar, the authors reported higher 6:2 FTS concentrations (5.57–56.8 μg kg−1) in great spider crab collected nearby the emission source compared to the average concentration observed at St1 in the present study where two individuals had quantifiable concentrations of 6:2 FTS (0.66 ± 0.43 μg kg−1 ww). Higher PFOS concentrations (3.70–39.00 μg kg−1 ww) were also reported for mud crab (claw meat) from a contaminated Australian coastal estuary.63 PFOS at relatively high levels (38–82 μg kg−1 dry weight) were measured in swimming crab collected from a river located in an industrial area of Tianjin, China.64
PFBS was detected at higher whole body concentrations (up to 8.5 μg kg−1 ww at St 2) than reported by Langberg et al.25 and was detected in 1 to 3 individuals at all impacted stations (St1–3). This indicates that PFBS has a bioaccumulation potential in crabs. This contradicts the pharmacokinetics reported for PFAS in rats, monkeys, and humans,65 although PFBS has been recently reported at 0.08 ± 0.11 μg kg−1 ww (whole body) in crabs (Goniopsis cruentata),66 and at trace levels in polar bear plasma (max 0.69 μg kg−1).53,67–69 Alternatively, PFBS in sediment can be an additional source for the invertebrates investigated. Higher PFBS concentrations were measured in fish tissues (<LOD to 16.90 ng g−1 of ww) from Yadkin-Pee Dee River, USA.70 Penland et al.70 assumed that the biotransformation of an unquantified PFBS precursor may be responsible for the unexpected high level of this compound.
Concentration and distribution patterns of PFAS in marine fish
PFAS levels were determined in muscle and liver samples of individuals from two species (sculpin and wolffish, as described earlier) collected from St1–St4, as summarized in Fig. 3, Tables S19 and S20.† Overall, low levels of PFAS were found in fish muscle samples (range
of 0.170–1.68 μg kg−1 ww) compared to liver samples (
ranged from 0.72 to 24.0 μg kg−1 ww). However, compared to water concentrations, PFAS enrichment (bioaccumulation) was seen for several PFAS in both muscle and liver (see Tables S19 and S20†).
For muscle samples (sculpin: n = 26 and wolffish: n = 3) long chain PFCA (C8–14), FOSA, PFHxS, as well as PFOS were detected in all samples investigated, whereas the short chain PFCA (PFHxA and PFHpA), and 6:2 FTS were not detected in any muscle sample. Likewise, PFBS was not detected in any muscle sample, and only in the liver of tow sculpin individuals at around 0.9 μg kg−1 ww concentration. PFOS was the predominant compound detected in all liver samples (sculpin: n = 13 and wolffish: n = 4) at an average concentration of ∑PFOS 2.2 ± 0.27 μg kg−1 ww. The average contribution of PFOS to
in fish liver was 40 ± 5%, and 18 ± 2% in muscle. The higher abundance of PFOS in liver confirms that PFOS tends to bioaccumulate in the liver compared to muscle tissue. This is in agreement with previous studies conducted on PFAS and PFOS specifically.71–74 In contrast, PFHxS showed a high contribution to
in muscle (31 ± 11%; maximum concentration = 0.48 μg kg−1 ww) compared to liver (28 ± 4%; maximum concentration = 3.0 μg kg−1 ww). Likewise, the contribution of long chain PFCA (C8–14) to
was 33 ± 5% and 26 ± 3% in fish muscle and liver, respectively. PFUnDA was the most predominant of these long chain PFCA detected at a maximum concentration of 0.127 μg kg−1 ww (in muscle) and 1.55 μg kg−1 ww (in liver) of individuals collected from St1.
Quantifiable concentrations of PFHxA (0.16 ± 0.10 μg kg−1 ww, average for 3 individuals out of 10) were detected in liver of individuals collected from St 3.
As expected for the benthic sculpin species, which do not migrate over significant distances,75 muscle samples of individual collected from the FFTS influenced stations (St1 and 2) showed significantly higher
concentration (0.955 ± 0.127 μg kg−1 ww; p = 0.030) than in individuals from the reference site (St4) (0.523 ± 0.127 μg kg−1 ww). Although, this difference is insignificant in liver samples (5.34 ± 1.74 and 5.20 ± 2.89 μg kg−1 ww in FFTS impacted station and the reference site, respectively), the highest average
concentration was observed in liver of fish collected from the FFTS impacted station (St1; 24.0 μg kg−1 ww) and the lowest was observed in the liver of the individual collected from the reference station (St4; 0.72 μg kg−1 ww). There were no significant differences in PFAS concentrations observed between the two investigated fish species, although differences in specific accumulation and elimination behaviour of individual PFAS have been found for different fish species in Lake Ontario.73
Overall, the PFAS profile in fish investigated here is consistent with the PFAS profile of fish collected from AFFF impacted waters.76 Data for PFAS levels in Arctic coastal fish populations is limited. In a previous study, PFOS and FOSA dominated in livers from the same sculpin species (Myoxocephalus scorpius) sampled close to a city or settlement from Iceland and the Faroe islands.74 PFHxA constituted a significant proportion of the ∑PFAS in sculpin livers from Iceland.74 These authors reported similar PFAS concentrations (
< 10 μg kg−1 ww) in sculpins collected from Faroe Islands, and higher PFAS concentrations (
> 60 μg kg−1 ww) in those from Iceland.
Based upon the current results, bioaccumulation factors (BAF) were calculated for selected PFAS where concentrations were above the LOQ for both water and fish (Table S21†). The log
BAFs of the investigated PFAA in the fish liver were higher than in the muscle, which is consistent with a recent study.77 For instance, the tendency of PFOS to bioconcentrate in liver rather than fish muscle is clearly shown (Tables S19 and S20†), as previously reported.78 This is most likely due to the affinity of PFAS to bind to proteins involved in fatty acid transport and metabolism such as liver fatty acid binding proteins.79,80 In line with several previous studies, log
BAFs of PFCA positively correlate with the perfluorinated carbon chain length.73,77 log
BAF increased from PFOA (log
BAFmuscle = 2.09 ± 0.103 and log
BAFliver = 2.87 ± 0.210) to PFDA (log
BAFmuscle = 3.19 ± 0.161 and log
BAFliver = 3.78 ± 0.357). However, PFUnDA showed lower log
BAF (log
BAFmuscle = 2.61 ± 0.110 and log
BAFliver = 3.41 ± 0.221), possibly due to a decreased gill permeability.73 A similar trend was observed for forage fish from Etobicoke and Spring Creeks nearby Toronto International Airport where the author reported comparable log
BAFliver values.81 However, differences in fish species and diets are the important factors for PFAS accumulation, and hence determine BAF values.
In the current study, PFOS liver/muscle concentration ratios calculated for individual fish ranged between 5 and 52 with an average of 18. These ratios are comparable to most previously reported values for different fish species collected from various locations. For instance, Pan et al.71 reported ratios for PFOS ranging from 6.9 to 42 for fish species collected from Chinese rivers. Becker et al.82 reported a value of 9.5 for PFOS in chub from a German river. In addition, Nania et al.83 reported a ratio of 61.5 for different pelagic and benthic marine fishes collected from the Mediterranean Sea, which is comparable to the range in the current study.
Concentration and distribution patterns of PFAS in glaucous gull (Larus hyperboreus)
The occurrence of a multitude of organic pollutants (including PFAS) in seabirds is one of the main causes of concern for seabird species in the Norwegian Arctic. Previous analyses of glaucous gull samples collected from Svalbard have detected several organic pollutants accumulated in their tissues.84–86 It has been estimated that the breeding population of the glaucous gull on Bjørnøya in the Svalbard archipelago declined with 65% from 1986 to 2010 mainly due to elevated pollutant levels.87
Glaucous gull represents a high trophic level in the Arctic marine food web. In this study, 20 glaucous gulls were collected in the vicinity of Longyearbyen and analysed for PFAS. In total, 9 PFAS could be quantified in 20 glaucous gull livers as shown in Table S22† and Fig. 4. PFOS was the predominant PFAS detected in all individual samples at concentrations varying from 12.7–433 μg kg−1 ww, representing approximately 80% of
. Haukås et al.88 reported a concentration of 65.8 ± 22.4 μg kg−1 ww (n = 9) for PFOS in glaucous gull livers collected in the Eastern Barents Sea close to Svalbard, which is slightly higher than the PFOS concentration reported herein (55.0 ± 20.5 μg kg−1 ww). Tomy et al.54 reported PFOS concentrations (20.2 ± 3.9 μg kg−1 ww) in glaucous gulls livers sampled in 2007 from Eastern Arctic background locations which were approximately 40% of the herein reported concentrations.
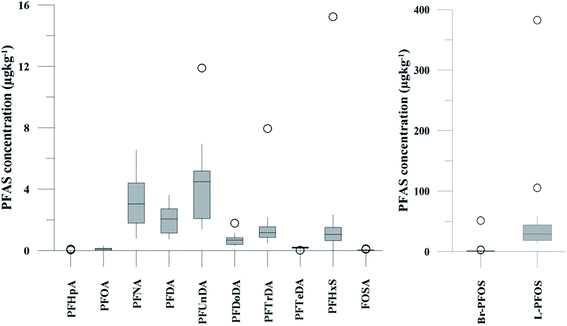 |
| Fig. 4 PFAS concentration measured in glaucous gull individuals (n = 20) collected in the vicinity of Longyearbyen (Svalbard) sampled in 2018. The horizontal bisecting lines show the medians; boxes show the first and third quartiles; whiskers represent the interquartile range. The circles represent concentrations > 1.5 times the interquartile range from the edge of the box. | |
The second most abundant PFAS group was the odd-numbered long chain length PFCA (C9, C11, C13), which were detected at high concentration (3.16 ± 0.375, 4.38 ± 0.556, 1.55 ± 0.351 μg kg−1 ww, respectively) compared to the even-length PFCA homologues (8, 10, and 12 14, at 0.101 ± 0.025, 1.95 ± 0.190, 0.710 ± 0.078 and 0.182 ± 0.015 μg kg−1 ww respectively). This means that the odd-numbered PFCA was higher than the adjacent shorter even-numbered PFCA. This is in agreement with recent studies conducted on PFASs in plasma samples of glaucous gull from Svalbard.86,89 This observation has also been made for other Arctic biota, including fish, birds and mammals.90 Long-range transport and degradation of FTOHs is assumed as a source for the observed long-chain PFCAs in arctic animals.18 Strong positive correlations were observed between PFOA and PFNA and between PFDA and PFUnDA (r > 0.7, p < 0.001), confirming their similar source. In contrast PFCA showed strong negative correlations with PFOS, suggesting a different source and transformation pathway (r > 0.8, p < 0.001). Assuming that the source of the long-chain PFCAs is the transformation of typically even-numbered FTOHs which degrade into odd and even-numbered PFCAs at similar yield, the abundance of odd-numbered PFCAs of higher chain length can be attributed to the higher bioaccumulation.18 For instance, 8
:
2 FTOH forms both PFOA and PFNA in equal yields, but as PFNA is more bioaccumulative,91 suggesting that glaucous gull samples carry a higher load of PFNA than the even-numbered homologue PFOA. Likewise, the degradation of 10
:
2 FTOH and 12
:
2 FTOH and the subsequent bioaccumulation interpret the higher abundance of PFUnDA compared to PFDA and PFTrDA compared to PFDoDA.
Overall, high individual variability in distribution of PFAS in the livers of the collected glaucous gulls was observed, indicating individual differences in their feeding habits. Glaucous gulls have opportunistic feeding habits throughout the year, feeding on food items from human wastes, and preying on other seabirds, such as little auks (Alle alle) and black-legged kittiwakes (Rissa tridactyla), as well as fish, crabs and amphipods.92–94
At Svalbard, the glaucous gull (Larus hyperboreus) is considered the most important avian predator and occupies the same ecological niche as birds of prey further south.94 Most bird species in Svalbard migrate to Greenland and the open Barents Seas outside the nesting season.87 Some glaucous gulls also winter in the restricted ice-free waters near shore off Svalbard,87 although the wintering locations of the sampled individuals are not known. Food related uptake is today considered the main source of PFAS for seabirds, however other factors can drive accumulation patterns of PFAS such as metabolic capabilities, habitat use, or migration.60,95 Although there were no significant sex related differences in PFAS levels among the individuals investigated, female individuals showed a relatively higher
concentration 68.2 ± 14.7 than male individual 53.0 ± 18.0. This agrees with recent studies where sex-related differences in PFAS concentrations were reported.86,89 Since the gulls were sampled prior to breeding, it is likely that they have been exposed to and accumulated PFAS when feeding in their wintering grounds. Thus, the PFAS body burdens of the bird constitutes of a mixture of PFAS compounds that have accumulated during feeding in their unknown wintering grounds, and in the Adventfjord following their return to Svalbard. This makes it difficult to conclude on the dominant source. Nevertheless, the
liver concentrations in the present gulls were twice those in the fish livers, clearly documenting biomagnification of PFAS. Two gulls had relatively high concentrations of PFHxS, that could be related to local sources, but also could indicate that they wintered in the same area.
Pattern of perfluorooctane sulfonate (PFOS) isomers in the local marine environment
The production of PFOS and perfluorooctanesulfonyl fluoride (POSF) based products by 3M was carried out using electrochemical fluorination (ECF) which resulted in 70 ± 1.1% of the linear isomer and 30 ± 0.8% of the branched isomer.7,8 Therefore, comparing the profile of PFOS isomers (branched and linear) in environmental samples with their profile in the technical mixture produced by ECF could provide insights in to the transport and distribution of PFOS in the environment.96–98 Further, elevated percentages of branched PFOS isomers (Br-PFOS) caused by the preferential transformation of branched PFOS precursors can be used as an indicator of the contribution from PFOS precursors.99 However, this can be complicated by the fact that PFOS isomer patterns can be significantly influenced by differences in sorption and by the differential uptake and elimination of Br-PFOS compared to L-PFOS.99,100Fig. 5 and Table S23† show the relative distribution of total branched PFOS isomer (Br-PFOS) and linear PFOS (L-PFOS) in abiotic and biotic samples investigated in the current study.
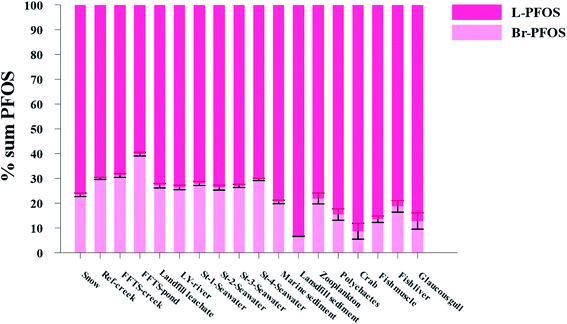 |
| Fig. 5 Relative distribution (mean ± SEM) of the sum [∑] branched PFOS isomer (Br-PFOS) versus linear PFOS (L-PFOS) in abiotic and biotic samples in the Longyearbyen area. (Error bars show ±standard error of mean, SEM.) | |
Abiotic samples
From Fig. 5 it is clear that the percentage of the isomers varies between sample matrices. Overall, it is notable that a reduced contribution of branched PFOS content was observed in most biota and sediment samples compared to water. For water samples, the PFOS isomer profile in run-off from the active FFTS area (FFTS-creek), appeared similar to the historical 3M ECF PFOS (30 ± 0.8% branched isomers), with Br-PFOS contribution of 30.0 ± 0.78% of total PFOS. Likewise, the isomer profiles observed in LY-river and landfill leachate (26.3 ± 0.91% and 27.1 ± 0.91% Br-PFOS, respectively) are comparable to the historical 3M ECF profile. Similar isomer profiles were observed in seawater samples (Br-PFOS contribution of 26–29% of total PFOS), suggesting that a large proportion of the PFOS contamination in seawater can be attributed to these local sources.
In the pond affected by the drainage from the airport and the old FFTS station, a higher Br-PFOS percentage was found (39.8 ± 0.78% of total PFOS). A similar PFOS isomer profile was observed in AFFF impacted sample collected at the training ground of a FFTS at Bergen airport, Norway.97 The reason for this branched enrichment at the pond is unclear, however the preferential degradation of the branched precursors of PFOS used at the FFTS and stronger sorption/uptake of L-PFOS are possible reasons.97,101,102 The limited water exchange between FFTS-pond water and seawater might be the reason for the observed branched enriched profile which allowed steady increase in the percentage of branched isomers over time, while water exchange reduces the branched isomers in seawater and river water. The deficiency in branched PFOS found in the surface snow sample could be explained by the preferential sorption of L-PFOS to the suspended particulate matter fraction.102 It should be noted that the Br-PFOS percentage did not correlate with the total PFOS concentration change (Fig. S7†). It has previously been reported that L-PFOS binds more strongly to organic matter than Br-PFOS, owing to its greater hydrophobicity.100 Compared to the marine sediment from the Adventfjord, sediment samples collected from the Longyearbyen landfill showed a higher contribution of L-PFOS. This can be attributed to the peat like nature of the landfill sediments in which PFAS can partition.51
Biotic samples
The biotic samples were dominated by L-PFOS with percentages ranging from 78 to 91. This is in agreement with several previous studies.103 For instance, in minnow (Hemiculter lcucisculus), and white shrimp (Exopalaemon), L-PFOS was found to contribute 78.6% and 95.5% of the total PFAS, respectively.103 Similarly, L-PFOS was found in more than 88% of the biota collected from Lake Ontario100 and was predominant in herring gull and polar bear from the Great Lakes and Arctic.104 These results indicate the selective bioaccumulation of L-PFOS in biota and/or the preferential excretion of Br-PFOS, which has been documented in different laboratory studies.105,106
The PFOS isomer pattern found in all benthic organisms investigated (polychaetes, crab, benthic fish) was similar to that found in sediment and more enriched in L-PFOS than in pelagic organisms 21.9 ± 2.2 (zooplankton). This greater relative percentage of Br-PFOS in the lowest tropic level could be due to the exposure of zooplankton to PFOS precursors (e.g. N-EtFOSE), where branched PFOS precursors are biotransformed at a higher rate compared to the linear precursors which leads to an enriched branched PFOS isomer profiles.99 Some systematic analytical bias resulting from matrix-induced ionization should be anticipated which is not unusual.107 Therefore, isomer specific analytical method applying exactly-matched isotopically-labelled internal standards for all isomers is needed for accurate isomer profiles. However, this does not diminish the importance of the observed relative differences of the isomer profiles between the samples.
Changes in distribution pattern of PFAS in the local environment
In this study, the PFAS distribution was characteristically different for the respective sampled media types. As shown in Fig. 6 and S6,† the abiotic samples from the assumed point source zones (FFTS-pond, -creek and landfill) contained high percentages of short and long-chained PFCA, specifically PFCA C6 to C9. This is also characteristic for the samples from LY-river and the assumed background sample Ref-creek. However, the source zones also contained 6:2 FTS. The snow sample was dominated by the long-chained PFSA (PFOS), similar to the profile observed in seawater. However, the snow sample contained a higher percentage of short-chained PFHxS. The marine sediment samples were dominated by the long chain PFCA > C10 in addition to 6:2 FTS and PFOS and contained short chain PFSA. Zooplankton was characterised by the presence of 6:2 FTS and long chain PFCA in addition to the presence of PFBS and PFOS. Similar to sediment, PFAS composition in the benthic organism polychaetes and crabs was dominated by long chain PFCA, PFOS, and PFBS in addition to some FOSA, and 6:2 FTS. The characteristic PFAS profiles in fish and glaucous gull were composed of a high proportion of PFOS.
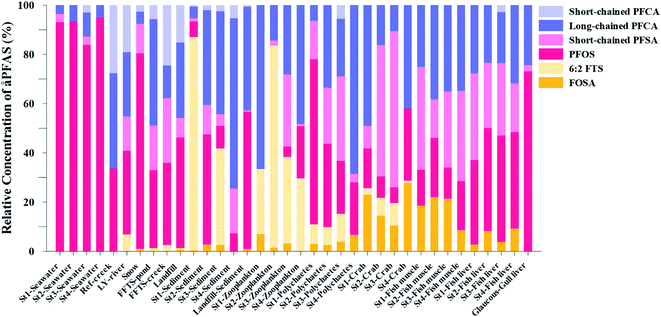 |
| Fig. 6 Average relative composition profile [%] for PFAS in abiotic and biotic samples collected from the Adventfjord and Isfjord, near the Svalbard Airport. (Levels < LOQ were treated as zero in this figure.) Short-chained PFCA: PFHxA and PFHpA; long-chained PFCA: C8–C14; short-chained PFSA: PFBS and PFHxS; long-chained PFSA: PFOS. | |
The relative contribution of total PFOS to
increased from zooplankton (30%), polychaetes (36%), crab (30%), fish (40%), to glaucous gull (72%), which indicates a high biomagnification potential of PFOS compared to other PFAS as previously reported.88,103,108,109 Transformation of PFOS precursor compounds110 has been suggested to be one reason for this.103 Supporting this, the relative contribution for FOSA, which is a PFOS precursor, decreased in our samples from zooplankton (71%) to crab and polychaetes (21.6 and 5% respectively) to 0.09% in glaucous gull. This suggests that the biotransformation of this precursor increases with increasing trophic level and consequently contributes to the relative amount of PFOS in the sampled organisms.54,111
Conclusion
The firefighting training stations (FFTS) at Svalbard airport and diffuse release from the local settlement were the major local PFAS sources. The high concentration observed in landfill leachate illustrates the wide application of PFAS in consumer products. Thus, local anthropogenic activity represents a significant source of PFAS. The much lower concentrations detected in the seawater samples suggests that following release from the point sources, significant dilution of PFAS occurred by seawater circulation in the coastal waters of the Adventfjord.
In the marine biota, PFAS levels increased from zooplankton to polychaete, crab, fish liver and gull liver. The distribution among the 14 target PFAS changed with increasing trophic level from low percentages of L-PFOS in in zooplankton and polychaetes to being dominated by linear PFOS in fish (40%) and gull liver (73%). Although the possible contribution of local sources to the relatively high PFAS concentrations in glaucous gulls cannot be evaluated, the high concentrations in their livers clearly demonstrates the biomagnification potential of PFAS, in particular L-PFOS. Results in the current study indicate bioaccumulation potential of compounds that have been taken into use as substitutes for PFOS, namely 6:2 FTS and PFBS in marine invertebrates, however they were not found in organisms at higher trophic levels. Toxicological information of this compounds remains unclear. Hence, further studies are needed to investigate the effect of exposure to these PFAS at the base of the marine food web.
Conflicts of interest
There are no conflicts to declare.
Acknowledgements
The research was funded by the Norwegian Research Council under the MILJØFORSK program for project number 268258 and under the POLARPROG for project number 268419. Martin Munch, Svalbard, is thanked for assistance in the field. We would like to thank the three anonymous reviewers for their valuable comments on our manuscript.
References
- K. J. Hansen, H. Johnson, J. Eldridge, J. Butenhoff and L. Dick, Quantitative characterization of trace levels of PFOS and PFOA in the Tennessee River, Environ. Sci. Technol., 2002, 36, 1681–1685 CrossRef CAS.
- K. Kannan, J. Koistinen, K. Beckmen, T. Evans, J. F. Gorzelany, K. J. Hansen, P. D. Jones, E. Helle, M. Nyman and J. P. Giesy, Accumulation of perfluorooctane sulfonate in marine mammals, Environ. Sci. Technol., 2001, 35, 1593–1598 CrossRef CAS.
- R. C. Buck, J. Franklin, U. Berger, J. M. Conder, I. T. Cousins, P. De Voogt, A. A. Jensen, K. Kannan, S. A. Mabury and S. P. van Leeuwen, Perfluoroalkyl and polyfluoroalkyl substances in the environment: terminology, classification, and origins, Integr. Environ. Assess. Manage., 2011, 7, 513–541 CrossRef CAS.
- Z. Wang, J. C. De Witt, C. P. Higgins and I. T. Cousins, A never-ending story of per- and polyfluoroalkyl substances (PFASs), Environ. Sci. Technol., 2017, 5, 2508–2518 CrossRef PubMed.
- M. Houde, J. W. Martin, R. J. Letcher, K. R. Solomon and D. C. Muir, Biological monitoring of polyfluoroalkyl substances: a review, Emerg. Contam., 2006, 40, 3463–3473 CrossRef CAS PubMed.
- C. Lau, K. Anitole, C. Hodes, D. Lai, A. Pfahles-Hutchens and J. Seed, Perfluoroalkyl acids: a review of monitoring and toxicological findings, Toxicol. Sci., 2007, 99, 366–394 CrossRef CAS PubMed.
- S. M. Vyas, I. Kania-Korwel and H.-J. J. Lehmler, Differences in the isomer composition of perfluorooctanesulfonyl (PFOS) derivatives, J. Environ. Sci. Health, Part A: Toxic/Hazard. Subst. Environ. Eng., 2007, 42, 249–255 CrossRef CAS PubMed.
-
3M Company, Fluorochemical isomer distribution by 19F-NMR spectroscopy, Public Docket AR226-0564, U.S. Environmental Protection Agency, Washington DC, 1997 Search PubMed.
- N. L. Stock, V. I. Furdui, D. C. Muir and S. A. Mabury, Perfluoroalkyl contaminants in the Canadian Arctic: evidence of atmospheric transport and local contamination, Environ. Sci. Technol., 2007, 41, 3529–3536 CrossRef CAS.
- D. Muir, R. Bossi, P. Carlsson, M. Evans, A. De Silva, C. Halsall, C. Rauert, D. Herzke, H. Hung and R. Letcher, Levels and trends of poly- and perfluoroalkyl substances in the Arctic environment – an update, Emerg. Contam., 2019, 5, 240–271 CrossRef.
- C. A. de Wit, J. Balmer, D. C. Muir, K. Vorkamp and S. Wilson, Chemicals of emerging Arctic concern: preface, Emerg. Contam., 2019, 5, 1–3 CrossRef.
- F. Wania and D. Mackay, A Global Distribution Model for Persistent Organic-Chemicals, Sci. Total Environ., 1995, 160–161, 211–232 CrossRef.
- M. So, S. Taniyasu, N. Yamashita, J. Giesy, J. Zheng, Z. Fang, S. Im and P. K. Lam, Perfluorinated compounds in coastal waters of Hong Kong, South China, and Korea, Environ. Sci. Technol., 2004, 38, 4056–4063 CrossRef CAS.
- J. W. Martin, D. C. Muir, C. A. Moody, D. A. Ellis, W. C. Kwan, K. R. Solomon and S. A. Mabury, Collection of airborne fluorinated organics and analysis by gas chromatography/chemical ionization mass spectrometry, Anal. Chem., 2002, 74, 584–590 CrossRef CAS PubMed.
- M. Shoeib, T. Harner, M. Ikonomou and K. Kannan, Indoor and outdoor air concentrations and phase partitioning of perfluoroalkyl sulfonamides and polybrominated diphenyl ethers, Environ. Sci. Technol., 2004, 38, 1313–1320 CrossRef CAS.
- M. Shoeib, T. Harner, B. H. Wilford, K. C. Jones and J. Zhu, Perfluorinated sulfonamides in indoor and outdoor
air and indoor dust: occurrence, partitioning, and human exposure, Environ. Sci. Technol., 2005, 39, 6599–6606 CrossRef CAS PubMed.
- N. L. Stock, F. K. Lau, D. A. Ellis, J. W. Martin, D. C. Muir and S. A. Mabury, Polyfluorinated telomer alcohols and sulfonamides in the North American troposphere, Environ. Sci. Technol., 2004, 38, 991–996 CrossRef CAS PubMed.
- D. A. Ellis, J. W. Martin, A. O. De Silva, S. A. Mabury, M. D. Hurley, M. P. Sulbaek Andersen and T. J. Wallington, Degradation of fluorotelomer alcohols: a likely atmospheric source of perfluorinated carboxylic acids, Environ. Sci. Technol., 2004, 38, 3316–3321 CrossRef CAS PubMed.
- H. M. Pickard, A. S. Criscitiello, C. Spencer, M. J. Sharp, D. C. Muir, A. O. De Silva and C. J. Young, Continuous non-marine inputs of per- and polyfluoroalkyl substances to the High Arctic: a multi-decadal temporal record, Atmos. Chem. Phys., 2018, 18, 5045–5058 CrossRef CAS.
- C. M. Butt, U. Berger, R. Bossi and G. T. Tomy, Levels and trends of poly- and perfluorinated compounds in the arctic environment, Sci. Total Environ., 2010, 408, 2936–2965 CrossRef CAS PubMed.
- C. J. Young, V. I. Furdui, J. Franklin, R. M. Koerner, D. C. Muir and S. A. Mabury, Perfluorinated acids in arctic snow: new evidence for atmospheric formation, Environ. Sci. Technol., 2007, 41, 3455–3461 CrossRef CAS PubMed.
- J. H. Johansson, M. E. Salter, J. A. Navarro, C. Leck, E. D. Nilsson and I. T. P. Cousins, Global transport of perfluoroalkyl acids via sea spray aerosol, Environ. Sci.: Processes Impacts, 2019, 21, 635–649 RSC.
- F. Wong, M. Shoeib, A. Katsoyiannis, S. Eckhardt, A. Stohl, P. Bohlin-Nizzetto, H. Li, P. Fellin, Y. Su and H. Hung, Assessing temporal trends and source regions of per- and polyfluoroalkyl substances (PFASs) in air under the Arctic Monitoring and Assessment Programme (AMAP), Atmos. Environ., 2018, 172, 65–73 CrossRef CAS.
- N. A. Warner, K. Sagerup, S. Kristoffersen, D. Herzke, G. W. Gabrielsen and B. M. Jenssen, Snow buntings (Plectrophenax nivealis) as bio-indicators for exposure differences to legacy and emerging persistent organic pollutants from the Arctic terrestrial environment on Svalbard, Sci. Total Environ., 2019, 667, 638–647 CrossRef CAS PubMed.
- H. A. Langberg, G. D. Breedveld, H. M. Grønning, M. Kvennås, B. M. Jenssen and S. E. Hale, Bioaccumulation of fluorotelomer sulfonates and perfluoroalkyl acids in marine organisms living in aqueous film-forming foam impacted waters, Environ. Sci. Technol., 2019, 53, 10951–10960 CrossRef CAS PubMed.
- J. P. Benskin, B. Li, M. G. Ikonomou, J. R. Grace and L. Y. Li, Per- and polyfluoroalkyl substances in landfill leachate: patterns, time trends, and sources, Environ. Sci. Technol., 2012, 46, 11532–11540 CrossRef CAS PubMed.
- B. M. Allred, J. R. Lang, M. A. Barlaz and J. Field, Orthogonal zirconium diol/C18 liquid chromatography-tandem mass spectrometry analysis of poly and perfluoroalkyl substances in landfill leachate, J. Chromatogr. A, 2014, 1359, 202–211 CrossRef CAS PubMed.
- L. J. Ahrens, Polyfluoroalkyl compounds in the aquatic environment: a review of their occurrence and fate, Environ. Monit. Assess., 2011, 13, 20–31 RSC.
-
Statistics Norway, 2020, http://ssb.no/en/befsvalbard/ Search PubMed.
-
R. Roura, The footprint of polar tourism: tourist behaviour at cultural heritage sites in Antarctica and Svalbard, 2011, Circumpolar Studies Volume 7, University of Groningen, Groningen, Arctic Centre Search PubMed.
-
M. E. Granberg, A. Ask and G. W. Gabrielsen, Local Contamination in Svalbard – Overview and Suggestions for Remediation Actions, Norwegian Polar Institute, Report 044, 2017 Search PubMed.
- A. Kalinowska, M. Szopińska, S. Chmiel, M. Kończak, Ż. Polkowska, W. Artichowicz, K. Jankowska, A. Nowak and A. Łuczkiewicz, Heavy metals in a high arctic fiord and their introduction with the wastewater: a case study of Adventfjorden-Longyearbyen system, Svalbard, Water, 2020, 12, 794 CrossRef CAS.
- H. Knutsen, T. Mæhlum, K. Haarstad, G. A. Slinde and H. P. H. Arp, Leachate emissions of short- and long-chain per- and polyfluoroalkyl substances (PFASs) from various Norwegian landfills, Environ. Sci.: Processes Impacts, 2019, 21, 1970–1979 RSC.
- C. R. Powley, S. W. George, T. W. Ryan and R. C. Buck, Matrix effect-free analytical methods for determination of perfluorinated carboxylic acids in environmental matrixes, Anal. Chem., 2005, 77, 6353–6358 CrossRef CAS PubMed.
- J. S. Skaar, E. M. Ræder, J. L. Lyche, L. Ahrens and R. J. E. S. Kallenborn, Elucidation of contamination sources for poly- and perfluoroalkyl substances (PFASs) on Svalbard (Norwegian Arctic), Environ. Sci. Pollut. Res., 2019, 26, 7356–7363 CrossRef CAS PubMed.
- C. R. Powley, S. W. George, M. H. Russell, R. A. Hoke and R. C. Buck, Polyfluorinated chemicals in a spatially and temporally integrated food web in the Western Arctic, Chemosphere, 2008, 70, 664–672 CrossRef CAS PubMed.
- F. Menger, J. Pohl, L. Ahrens, G. Carlsson and S. Örn, Behavioural effects and bioconcentration of per- and polyfluoroalkyl substances (PFASs) in zebrafish (Danio rerio) embryos, Chemosphere, 2020, 245, 125573 CrossRef CAS PubMed.
- E. Nyberg, R. Awad, A. Bignert, C. Ek, G. Sallsten and J. P. Benskin, Inter-individual, inter-city, and temporal trends of per- and polyfluoroalkyl substances in human milk from Swedish mothers between 1972 and 2016, Environ. Sci.: Processes Impacts, 2018, 20, 1136–1147 RSC.
- N. Riddell, G. Arsenault, J. P. Benskin, B. Chittim, J. W. Martin, A. McAlees and R. McCrindle, Branched perfluorooctane sulfonate isomer quantification and characterization in blood serum samples by HPLC/ESI-MS (/MS), Environ. Sci. Technol., 2009, 43, 7902–7908 CrossRef CAS PubMed.
- J. W. Martin, K. Kannan, U. Berger, P. D. Voogt, J. Field, J. Franklin, J. P. Giesy, T. Harner, D. C. Muir and B. Scott, Peer reviewed: analytical challenges hamper perfluoroalkyl research, Environ. Sci. Technol., 2004, 38, 248A–255A CrossRef CAS PubMed.
- I. Fuertes, S. Gómez-Lavín, M. Elizalde and A. Urtiaga, Perfluorinated alkyl substances (PFASs) in northern Spain municipal solid waste landfill leachates, Chemosphere, 2017, 168, 399–407 CrossRef CAS PubMed.
- C. Gallen, D. Drage, S. Kaserzon, C. Baduel, M. Gallen, A. Banks, S. Broomhall and J. J. Mueller, Occurrence and distribution of brominated flame retardants and perfluoroalkyl substances in Australian landfill leachate and biosolids, J. Hazard. Mater., 2016, 312, 55–64 CrossRef CAS PubMed.
- Avinor, 2020, PFOS i fokus, https://avinor.no/en/corporate/community-and-environment/pfos-i-fokus/pfos-i-fokus, 5. October 2020 Search PubMed.
- C. Bach, V. Boiteux, J. Hemard, A. Colin, C. Rosin, J.-F. Munoz and X. J. Dauchy, Simultaneous determination of perfluoroalkyl iodides, perfluoroalkane sulfonamides, fluorotelomer alcohols, fluorotelomer iodides and fluorotelomer acrylates and methacrylates in water and sediments using solid-phase microextraction-gas chromatography/mass spectrometry, J. Chromatogr. A, 2016, 1448, 98–106 CrossRef CAS PubMed.
- K. Y. Kwok, E. Yamazaki, N. Yamashita, S. Taniyasu, M. B. Murphy, Y. Horii, G. Petrick, R. Kallerborn, K. Kannan and K. Murano, Transport of perfluoroalkyl substances (PFAS) from an arctic glacier to downstream locations: implications for sources, Sci. Total Environ., 2013, 447, 46–55 CrossRef CAS PubMed.
- L. Ahrens, W. Gerwinski, N. Theobald and R. Ebinghaus, Sources of polyfluoroalkyl compounds in the North Sea, Baltic Sea and Norwegian Sea: evidence from their spatial distribution in surface water, Mar. Pollut. Bull., 2010, 60, 255–260 CrossRef CAS PubMed.
- L. Ahrens, S. Felizeter, R. Sturm, Z. Xie and R. Ebinghaus, Polyfluorinated compounds in waste water treatment plant effluents and surface waters along the River Elbe, Germany, Mar. Pollut. Bull., 2009, 58, 1326–1333 CrossRef CAS PubMed.
- K. Prevedouros, I. T. Cousins, R. C. Buck and S. H. Korzeniowski, Sources, fate and transport of perfluorocarboxylates, Environ. Sci. Technol., 2006, 40, 32–44 CrossRef CAS PubMed.
- J. J. MacInnis, K. French, D. C. Muir, C. Spencer, A. Criscitiello, A. O. De Silva and C. S. P. Young, Emerging investigator series: a 14 year depositional ice record of perfluoroalkyl substances in the High Arctic, Environ. Sci.: Processes Impacts, 2017, 19, 22–30 RSC.
- C. J. McMurdo, D. A. Ellis, E. Webster, J. Butler, R. D. Christensen and L. K. Reid, Aerosol enrichment of the surfactant PFO and mediation of the water–air transport of gaseous PFOA, Environ. Sci. Technol., 2008, 42, 3969–3974 CrossRef CAS PubMed.
- C. P. Higgins and R. G. Luthy, Sorption of perfluorinated surfactants on sediments, Environ. Sci. Technol., 2006, 40, 7251–7256 CrossRef CAS PubMed.
- J. M. Conder, R. A. Hoke, W. d. Wolf, M. H. Russell and R. C. Buck, Are PFCAs bioaccumulative? A critical review and comparison with regulatory criteria and persistent lipophilic compounds, Environ. Sci. Technol., 2008, 42, 995–1003 CrossRef CAS PubMed.
- W. A. Gebbink, A. Bignert and U. Berger, Perfluoroalkyl acids (PFAAs) and selected precursors in the Baltic Sea Environment: do precursors play a role in food web accumulation of PFAAs?, Environ. Sci. Technol., 2016, 50, 6354–6362 CrossRef CAS PubMed.
- G. T. Tomy, W. Budakowski, T. Halldorson, P. A. Helm, G. A. Stern, K. Friesen, K. Pepper, S. A. Tittlemier and A. T. Fisk, Fluorinated organic compounds in an eastern Arctic marine food web, Environ. Sci. Technol., 2004, 38, 6475–6481 CrossRef CAS PubMed.
- M. Frederiksen, M. Edwards, A. J. Richardson, N. C. Halliday and S. Wanless, From plankton to top predators: bottom-up control of a marine food web across four trophic levels, J. Anim. Ecol., 2006, 75, 1259–1268 CrossRef PubMed.
- D. Bertin, P. Labadie, B. J. Ferrari, A. Sapin, J. Garric, O. Geffard, H. Budzinski and M. Babut, Potential exposure routes and accumulation kinetics for poly- and perfluorinated alkyl compounds for a freshwater amphipod: Gammarus spp. (Crustacea), Chemosphere, 2016, 155, 380–387 CrossRef CAS PubMed.
- D. Bertin, B. J. Ferrari, P. Labadie, A. Sapin, J. Garric, H. Budzinski, M. Houde and M. Babut, Bioaccumulation of perfluoroalkyl compounds in midge (Chironomus riparius) larvae exposed to sediment, Environ. Pollut., 2014, 189, 27–34 CrossRef CAS.
- M. Chen, Q. Wang, Y. Zhu, L. Zhu, B. Xiao, M. Liu and L. Yang, Species dependent accumulation and transformation of 8
:
2 polyfluoroalkyl phosphate esters in sediment by three benthic organisms, Environ. Int., 2019, 133, 105171 CrossRef CAS PubMed.
- F. A. Labra, R. A. Moreno, S. A. Alvarado, F. D. Carrasco, S. A. Estay and M. Rivadeneira, The relative role of ecological interactions and environmental variables on the population dynamics of marine benthic polychaetes, Mar. Biodivers., 2018, 48, 1203–1212 CrossRef.
- G. L. Lescord, K. A. Kidd, A. O. De Silva, M. Williamson, C. Spencer, X. Wang and D. C. Muir, Perfluorinated and polyfluorinated compounds in lake food webs from the Canadian High Arctic, Environ. Sci. Technol., 2015, 49, 2694–2702 CrossRef CAS PubMed.
- G. Munoz, M. Desrosiers, L. Vetter, S. Vo Duy, J. Jarjour, J. Liu and S. Sauvé, Bioaccumulation of zwitterionic polyfluoroalkyl substances
in earthworms exposed to aqueous film-forming foam impacted soils, Environ. Sci. Technol., 2020, 54, 1687–1697 CrossRef CAS PubMed.
-
A. Ruus, K. Bæk, K. Petersen, I. Allan, B. Beylich, M. Schlabach, N. A. Warner, K. Borgå and M. J. N.-r. Helberg, Environmental Contaminants in an Urban Fjord, 2017, 2018 Search PubMed.
- M. D. Taylor, K. C. Bowles, D. D. Johnson and N. A. Moltschaniwskyj, Depuration of perfluoroalkyl substances from the edible tissues of wild-caught invertebrate species, Sci. Total Environ., 2017, 581, 258–267 CrossRef PubMed.
- T. Wang, Y. Lu, C. Chen, J. E. Naile, J. S. Khim and J. P. Giesy, Perfluorinated compounds in a coastal industrial area of Tianjin, China, Environ. Geochem. Health, 2012, 34, 301–311 CrossRef CAS PubMed.
- G. W. Olsen, S.-C. Chang, P. E. Noker, G. S. Gorman, D. J. Ehresman, P. H. Lieder and J. L. Butenhoff, A comparison of the pharmacokinetics of perfluorobutanesulfonate (PFBS) in rats, monkeys, and humans, Toxicology, 2009, 256, 65–74 CrossRef CAS PubMed.
- D. A. Miranda, J. P. Benskin, R. Awad, G. Lepoint, J. Leonel and V. Hatje, Bioaccumulation of per- and polyfluoroalkyl substances (PFASs) in a tropical estuarine food web, Sci. Total Environ., 2021, 754, 142146 CrossRef CAS PubMed.
- W. A. Gebbink, R. Bossi, F. F. Rigét, A. Rosing-Asvid, C. Sonne and R. Dietz, Observation of emerging per- and polyfluoroalkyl substances (PFASs) in Greenland marine mammals, Chemosphere, 2016, 144, 2384–2391 CrossRef CAS PubMed.
- H. Routti, G. W. Gabrielsen, D. Herzke, K. M. Kovacs and C. Lydersen, Spatial and temporal trends in perfluoroalkyl substances (PFASs) in ringed seals (Pusa hispida) from Svalbard, Environ. Pollut., 2016, 214, 230–238 CrossRef CAS PubMed.
- H. Routti, J. Aars, E. Fuglei, L. Hanssen, K. Lone, A. Polder, Å. Ø. Pedersen, S. Tartu, J. M. Welker and N. G. Yoccoz, Emission changes dwarf the influence of feeding habits on temporal trends of per- and polyfluoroalkyl substances in two Arctic top predators, Environ. Sci. Technol., 2017, 51, 11996–12006 CrossRef CAS.
- T. N. Penland, W. G. Cope, T. J. Kwak, M. J. Strynar, C. A. Grieshaber, R. J. Heise and F. W. Sessions, Trophodynamics of Per- and Polyfluoroalkyl Substances in the Food Web of a Large Atlantic Slope River, Environ. Sci. Technol., 2020, 54, 6800–6811 CrossRef CAS PubMed.
- C.-G. Pan, J.-L. Zhao, Y.-S. Liu, Q.-Q. Zhang, Z.-F. Chen, H.-J. Lai, F.-J. Peng, S.-S. Liu and G.-G. Ying, Bioaccumulation and risk assessment of per- and polyfluoroalkyl substances in wild freshwater fish from rivers in the Pearl River Delta region, South China, Ecotoxicol. Environ. Saf., 2014, 107, 192–199 CrossRef CAS.
- Y. Shi, J. Wang, Y. Pan and Y. Cai, Tissue distribution of perfluorinated compounds in farmed freshwater fish and human exposure by consumption, Environ. Chem., 2012, 31, 717–723 CrossRef CAS PubMed.
- J. W. Martin, S. A. Mabury, K. R. Solomon and D. C. Muir, Bioconcentration and tissue distribution of perfluorinated acids in rainbow trout (Oncorhynchus mykiss), Environ. Toxicol. Chem., 2003, 22, 196–204 CrossRef CAS.
-
R. Kallenborn, Perfluorinated alkylated substances (PFAS) in the Nordic environment, Nordic Council of Ministers, Copenhagen, 2004, vol. 552 Search PubMed.
- B. P. Gray, B. L. Norcross, A. H. Beaudreau, A. L. Blanchard and O. Seitz, Food habits of Arctic staghorn sculpin (Gymnocanthus tricuspis) and shorthorn sculpin (Myoxocephalus scorpius) in the northeastern Chukchi and western Beaufort Seas, Deep Sea Res., Part II, 2017, 135, 111–123 CrossRef.
- H. A. Lanza, R. S. Cochran, J. F. Mudge, A. D. Olson, B. R. Blackwell, J. D. Maul, C. J. Salice and T. A. Anderson, Temporal monitoring of perfluorooctane sulfonate accumulation in aquatic biota downstream of historical aqueous film forming foam use areas, Environ. Toxicol. Chem., 2017, 36, 2022–2029 CrossRef CAS.
- M. Chen, L. Zhu, Q. Wang and G. Shan, Tissue distribution and bioaccumulation of legacy and emerging per- and polyfluoroalkyl substances (PFASs) in edible fishes from Taihu Lake, China, Environ. Pollut., 2021, 268, 115887 CrossRef CAS.
- S. Falk, K. Failing, S. Georgii, H. Brunn and T. Stahl, Tissue specific uptake and elimination of perfluoroalkyl acids (PFAAs) in adult rainbow trout (Oncorhynchus mykiss) after dietary exposure, Chemosphere, 2015, 129, 150–156 CrossRef CAS PubMed.
- D. J. Luebker, K. J. Hansen, N. M. Bass, J. L. Butenhoff and A. M. Seacat, Interactions of fluorochemicals with rat liver fatty acid-binding protein, Toxicology, 2002, 176, 175–185 CrossRef CAS PubMed.
- C. A. Ng and K. Hungerbühler, Bioconcentration of perfluorinated alkyl acids: how important is specific binding?, Environ. Sci. Technol., 2013, 47, 7214–7223 CrossRef CAS PubMed.
- E. Awad, X. Zhang, S. P. Bhavsar, S. Petro, P. W. Crozier, E. J. Reiner, R. Fletcher, S. A. Tittlemier and E. Braekevelt, Long-term environmental fate of perfluorinated compounds after accidental release at Toronto airport, Environ. Sci. Technol., 2011, 45, 8081–8089 CrossRef CAS PubMed.
- A. M. Becker, S. Gerstmann and H. Frank, Perfluorooctanoic acid and perfluorooctane sulfonate in two fish species collected from the Roter Main River, Bayreuth, Germany, Bull. Environ. Contam. Toxicol., 2010, 84, 132 CrossRef CAS PubMed.
- V. Nania, G. E. Pellegrini, L. Fabrizi, G. Sesta, P. De Sanctis, D. Lucchetti, M. Di Pasquale and E. J. Coni, Monitoring of perfluorinated compounds in edible fish from the Mediterranean Sea, Food Chem., 2009, 115, 951–957 CrossRef CAS.
- K. Sagerup, L. B. Helgason, A. Polder, H. Strøm, T. D. Josefsen, J. U. Skåre and G. W. Gabrielsen, Persistent organic pollutants and mercury in dead and dying glaucous gulls (Larus hyperboreus) at Bjørnøya (Svalbard), Sci. Total Environ., 2009, 407, 6009–6016 CrossRef CAS PubMed.
- K. Sagerup, V. Savinov, T. Savinova, V. Kuklin, D. C. Muir and G. W. Gabrielsen, Persistent organic pollutants, heavy metals and parasites in the glaucous gull (Larus hyperboreus) on Spitsbergen, Environ. Pollut., 2009, 157, 2282–2290 CrossRef CAS PubMed.
- M. Melnes, G. W. Gabrielsen, D. Herzke, K. Sagerup and B. M. Jenssen, Dissimilar effects of organohalogenated compounds on thyroid hormones in glaucous gulls, Environ. Res., 2017, 158, 350–357 CrossRef CAS PubMed.
-
T. Anker-Nilssen, R. T. Barrett, J. O. Bustnes, S. Christensen-Dalsgaard, K. E. Erikstad, P. Fauchald, S.-H. Lorentsen, H. Steen, H. Strøm and G. H. Systad, SEAPOP studies in the Barents and Norwegian Seas in 2007, 2008, http://hdl.handle.net/11250/2467433 Search PubMed.
- M. Haukås, U. Berger, H. Hop, B. Gulliksen and G. W. Gabrielsen, Bioaccumulation of per- and polyfluorinated alkyl substances (PFAS) in selected species from the Barents Sea food web, Environ. Pollut., 2007, 148, 360–371 CrossRef PubMed.
- M. Sebastiano, F. Angelier, P. Blevin, C. Ribout, K. Sagerup, S. Descamps, D. Herzke, B. Moe, C. Barbraud and J. O. Bustnes, Exposure to PFAS is associated with telomere length dynamics and demographic responses of an arctic top predator, Environ. Sci. Technol., 2020, 54, 10217–10226 CrossRef CAS PubMed.
- J. W. Martin, M. M. Smithwick, B. M. Braune, P. F. Hoekstra, D. C. Muir and S. A. Mabury, Identification of long-chain perfluorinated acids in biota from the Canadian Arctic, Environ. Sci. Technol., 2004, 38, 373–380 CrossRef CAS PubMed.
- J. W. Martin, S. A. Mabury, K. R. Solomon and D. C. Muir, Dietary accumulation of perfluorinated acids in juvenile rainbow trout (Oncorhynchus mykiss), Environ. Toxicol. Chem., 2003, 22, 189–195 CrossRef CAS.
-
C. Lydersen, I. Giertz and J. M. Weslawski, Aspects of vertebrate feeding in the marine ecosystem in Hornsund, Svalbard, 1985 Search PubMed.
-
H. J. S. Løvenskiold, Avifauna Svalbardensis//Norsk Polarinsr, 1964, 129 Search PubMed.
- G. W. Gabrielsen, J. U. Skaare, A. Polder and V. Bakken, Chlorinated hydrocarbons in glaucous gulls (Larus hyperboreus) in the southern part of Svalbard, Sci. Total Environ., 1995, 160, 337–346 CrossRef.
- E. H. Leat, S. Bourgeon, E. Magnusdottir, G. W. Gabrielsen, W. J. Grecian, S. A. Hanssen, K. Olafsdottir, A. Petersen, R. A. Phillips and H. Strøm, Influence of wintering area on persistent organic pollutants in a breeding migratory seabird, Mar. Ecol.: Prog. Ser., 2013, 491, 277–293 CrossRef CAS.
- J. P. Benskin, L. W. Yeung, N. Yamashita, S. Taniyasu, P. K. Lam and J. W. Martin, Perfluorinated acid isomer profiling in water and quantitative assessment of manufacturing source, Environ. Sci. Technol., 2010, 44, 9049–9054 CrossRef CAS.
- A. Kärrman, K. Elgh-Dalgren, C. Lafossas and T. Møskeland, Environmental levels and distribution of structural isomers of perfluoroalkyl acids after aqueous fire-fighting foam (AFFF) contamination, Environ. Chem., 2011, 8, 372–380 CrossRef.
- H. A. Langberg, H. P. H. Arp, G. D. Breedveld, G. A. Slinde, Å. Høiseter, H. M. Grønning, M. Jartun, T. Rundberget, B. M. Jenssen and S. E. Hale, Paper product production identified as the main source of per- and polyfluoroalkyl substances (PFAS) in a Norwegian lake: source and historic emission tracking, Environ. Pollut., 2021, 273, 116259 CrossRef CAS PubMed.
- J. P. Benskin, A. Holt and J. W. Martin, Isomer-specific biotransformation rates of a perfluorooctane sulfonate (PFOS)-precursor by cytochrome P450 isozymes and human liver microsomes, Environ. Sci. Technol., 2009, 43, 8566–8572 CrossRef CAS PubMed.
- M. Houde, G. Czub, J. M. Small, S. Backus, X. Wang, M. Alaee and D. C. Muir, Fractionation and bioaccumulation of perfluorooctane sulfonate (PFOS) isomers in a Lake Ontario food web, Environ. Sci. Technol., 2008, 42, 9397–9403 CrossRef CAS PubMed.
- Y. Li, X. Feng, J. Zhou and L. Zhu, Occurrence and source apportionment of novel and legacy poly/perfluoroalkyl substances in Hai River basin in China using receptor models and isomeric fingerprints, Water Res., 2020, 168, 115145 CrossRef CAS PubMed.
- G. Shan, X. Chen and L. Zhu, Occurrence, fluxes and sources of perfluoroalkyl substances with isomer analysis in the snow of northern China, J. Hazard. Mater., 2015, 299, 639–646 CrossRef CAS PubMed.
- S. Fang, X. Chen, S. Zhao, Y. Zhang, W. Jiang, L. Yang and L. Zhu, Trophic magnification and isomer fractionation of perfluoroalkyl substances in the food web of Taihu Lake, China, Environ. Sci. Technol., 2014, 48, 2173–2182 CrossRef CAS.
- S. Chu and R. Letcher, Linear and branched perfluorooctane sulfonate isomers in technical product and environmental samples by in-port derivatization-gas chromatography-mass spectrometry, Anal. Chem., 2009, 81, 4256–4262 CrossRef CAS PubMed.
- J. P. Benskin, A. O. De Silva, L. J. Martin, G. Arsenault, R. McCrindle, N. Riddell, S. A. Mabury and J. W. Martin, Disposition of perfluorinated acid isomers in Sprague-Dawley rats; part 1: single dose, Environ. Toxicol. Chem., 2009, 28, 542–554 CrossRef CAS PubMed.
- R. L. Sharpe, J. P. Benskin, A. H. Laarman, S. L. MacLeod, J. W. Martin, C. S. Wong and G. G. Goss, Perfluorooctane sulfonate toxicity, isomer-specific accumulation, and maternal transfer in zebrafish (Danio rerio) and rainbow trout (Oncorhynchus mykiss), Environ. Toxicol. Chem., 2010, 29, 1957–1966 CAS.
- D. A. Miranda, J. P. Benskin, R. Awad, G. Lepoint, J. Leonel and V. Hatje, Bioaccumulation of per- and polyfluoroalkyl substances (PFASs) in a tropical estuarine food web, Sci. Total Environ., 2021, 754, 142146 CrossRef CAS PubMed.
- B. C. Kelly, M. G. Ikonomou, J. D. Blair, B. Surridge, D. Hoover, R. Grace and F. A. Gobas, Perfluoroalkyl contaminants in an Arctic marine food web: trophic magnification and wildlife exposure, Environ. Sci. Technol., 2009, 43, 4037–4043 CrossRef CAS PubMed.
- J. W. Martin, D. M. Whittle, D. C. Muir and S. A. Mabury, Perfluoroalkyl contaminants in a food web from Lake Ontario, Environ. Sci. Technol., 2004, 38, 5379–5385 CrossRef CAS PubMed.
- D. A. Jackson and S. A. Mabury, Polyfluorinated amides as a historical PFCA source by electrochemical fluorination of alkyl sulfonyl fluorides, Environ. Sci. Technol., 2013, 47, 382–389 CrossRef CAS.
- G. T. Tomy, S. A. Tittlemier, V. P. Palace, W. R. Budakowski, E. Braekevelt, L. Brinkworth and K. Friesen, Biotransformation of N-ethyl perfluorooctanesulfonamide by rainbow trout (Oncorhynchus mykiss) liver microsomes, Environ. Sci. Technol., 2004, 38, 758–762 CrossRef CAS.
Footnote |
† Electronic supplementary information (ESI) available. See DOI: 10.1039/d0em00510j |
|
This journal is © The Royal Society of Chemistry 2021 |
Click here to see how this site uses Cookies. View our privacy policy here.