Reactivity-directed analysis – a novel approach for the identification of toxic organic electrophiles in drinking water†
Received
12th November 2020
, Accepted 10th December 2020
First published on 12th January 2021
Abstract
Drinking water consumption results in exposure to complex mixtures of organic chemicals, including natural and anthropogenic chemicals and compounds formed during drinking water treatment such as disinfection by-products. The complexity of drinking water contaminant mixtures has hindered efforts to assess associated health impacts. Existing approaches focus primarily on individual chemicals and/or the evaluation of mixtures, without providing information about the chemicals causing the toxic effect. Thus, there is a need for the development of novel strategies to evaluate chemical mixtures and provide insights into the species responsible for the observed toxic effects. This critical review introduces the application of a novel approach called Reactivity-Directed Analysis (RDA) to assess and identify organic electrophiles, the largest group of known environmental toxicants. In contrast to existing in vivo and in vitro approaches, RDA utilizes in chemico methodologies that investigate the reaction of organic electrophiles with nucleophilic biomolecules, including proteins and DNA. This review summarizes the existing knowledge about the presence of electrophiles in drinking water, with a particular focus on their formation in oxidative treatment systems with ozone, advanced oxidation processes, and UV light, as well as disinfectants such as chlorine, chloramines and chlorine dioxide. This summary is followed by an overview of existing RDA approaches and their application for the assessment of aqueous environmental matrices, with an emphasis on drinking water. RDA can be applied beyond drinking water, however, to evaluate source waters and wastewater for human and environmental health risks. Finally, future research demands for the detection and identification of electrophiles in drinking water via RDA are outlined.
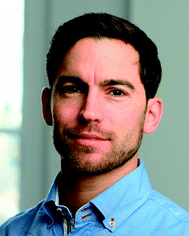 Carsten Prasse | Dr Carsten Prasse is an Assistant Professor in the Department of Environmental Health & Engineering at Johns Hopkins University. His research is driven by a fascination with environmental chemistry and a deep concern about the public and environmental health impacts of chemicals. His research focuses on the fate and effects of organic contaminants in the environment. Prioritizing which compounds are most threatening to human and environmental health is a great challenge given that thousands of chemicals are present in our waters. To tackle this problem, he is implementing concepts and methods from toxicology and public health with the goal of developing new methodologies to assess environmental exposure to anthropogenic chemicals. |
Environmental significance
The increasing number of contaminants detected in drinking water presents a major challenge for (bio)analytical water quality assessment. Organic electrophiles are a pressing concern due to their toxicity and widespread formation during (oxidative) water treatment. This critical review assesses the state of scientific knowledge on organic electrophiles in drinking water and proposes the use of a new analytical approach called Reactivity-Directed Analysis (RDA). RDA simultaneously identifies and assesses the toxicity of electrophiles in drinking water, bridging chemical and toxicological methodologies to tackle complex chemical mixtures.
|
A Introduction
Drinking water consumption exposes humans to complex mixtures of organic chemicals, which are present as a result of natural processes, contamination from anthropogenic activities, and formation during drinking water treatment.1,2 While modern analytical instruments allow us to detect an ever-increasing number of chemicals with increasing sensitivity, the complexity of these mixtures makes an evaluation of the resulting health impacts extremely challenging. Traditionally, the quality of drinking water has been assessed based on the occurrence of specific, regulated compounds with well-defined toxicities as determined by expensive, time-consuming animal studies.3,4 However, the growing number of anthropogenic chemicals that are detected in drinking water renders this chemical-by-chemical assessment approach unfeasible. Similarly, there is also a rising number of treatment processes, in particular those that use chemical oxidants (e.g., O3, O3/H2O2, UV/H2O2, HOCl, HOCl/NH3, UV/HOCl, and electrochemical oxidation) that result in the formation of a large spectrum of transformation products.5–7 Decades of research have demonstrated the presence of more than 700 disinfection by-products (DBPs) in drinking water treated by chlorination or chloramination.8 Yet, the dominant fraction of halogenated compounds remains unknown. In addition, only a small subset of DBPs (trihalomethanes (THMs), haloacetic acids (HAAs), bromate, and chlorite) are regulated, despite evidence of unregulated DBPs that exhibit higher toxicities.9 Health effects associated with exposure to DBPs include cancer, reduced fertility and fetal loss, respiratory diseases such as asthma, and skin diseases like allergic contact dermatitis.10–15 Based on epidemiological studies, clear evidence exists for the association between adverse health outcomes and exposure to regulated DBPs such as THMs.11 However, due to the lack of monitoring data for most drinking water contaminants, it is impossible to determine whether these regulated and widely monitored DBPs are indeed responsible, or whether the adverse health effects are attributable to other co-occurring DBPs. This uncertainty emphasizes the need for approaches that identify and prioritize the most relevant toxicants for toxicity assessment and drinking water monitoring.
In toxicology, high-throughput toxicity screening approaches, in particular in vitro bioassays, were developed to enable the evaluation of an increasing number of anthropogenic chemicals that are present in the environment.16–20 A large number of in vitro bioassays for most relevant toxicity endpoints are available today and have been utilized to assess thousands of chemicals. In addition, in vitro bioassays have also been increasingly applied for the toxicological assessment of environmental samples including wastewater, surface water, and drinking water.21–23 While these methods provide useful insights into the overall toxicity of complex mixtures, they do not provide any information regarding the chemicals responsible for the toxicity. With regard to drinking water and the expanding use of oxidative treatment technologies, another key limitation of current in vitro assays is the use of a preconcentration step such as specific resins (e.g. XAD), solid-phase extraction, liquid–liquid extraction, and freeze-drying, which is often necessary to achieve a sufficient sensitivity. The preconcentration methods, however, bias the results toward compounds that survive the work-up procedure. As such, highly polar compounds as well as substances that are volatile or reactive are likely lost.24–26
One approach gaining popularity to identify chemicals responsible for an observed toxic effect in complex environmental mixtures is the use of effect-directed analysis (EDA).27,28 As a first step, EDA employs in vitro bioassays to assess the toxicity of the investigated environmental sample. If a toxic effect is observed, the mixture is fractionated (generally using a chromatographic system and separation based on retention time) followed by in vitro toxicity testing of the individual fractions. For those fractions showing toxic effects, the process is typically repeated to further isolate the chemicals present in each fraction, which are then identified using analytical chemistry approaches such as gas chromatography-mass spectrometry (GC-MS) or liquid chromatography-mass spectrometry (LC-MS).27,29,30 However, similar to the aspects discussed for in vitro bioassays, the extensive manipulation of the samples, including sample extraction and fractionation, likely results in the loss of highly polar, volatile, and reactive compounds. This is particularly relevant for transformation products formed in oxidative drinking water, which typically show at least one of these characteristics.
Overcoming these shortcomings requires the development of approaches that enable a direct assessment of complex mixtures, i.e. that do not focus only on chemicals that survive the work-up procedure, but also allow for the identification of the responsible toxicants.18,31 This review introduces the application of a novel approach called reactivity-directed analysis (RDA) to address these shortcomings. In contrast to existing in vitro and in vivo approaches, RDA represents an in chemico approach (i.e., in the absence of organisms or cells) for the identification and toxicological assessment of organic electrophiles, the largest class of known toxic chemicals.32–36 This review is structured into four different sections that focus on (i) the relevance of electrophiles as environmental toxicants, (ii) occurrence of electrophiles in drinking water, (iii) application of RDA to identify the presence of electrophiles in complex environmental mixtures, and (iv) future research needs.
B Organic chemistry meets molecular toxicology: electrophiles as toxicants
Advances in molecular toxicology have fundamentally changed our understanding of how chemical exposure is linked to adverse health effects. This includes the development of the adverse outcome pathway conceptional framework that outlines the cascade of biochemical and cellular processes that lead to a toxic effect.37 Of particular relevance is the molecular interaction of a chemical with a biomolecule as an initial step, also called the molecular initiating event (MIE). MIEs include non-covalent interactions of chemicals with biomolecules (e.g. receptor binding), the oxidation of biomolecules by reactive oxygen species and reactive nitrogen species (e.g. due to oxidative stress), and covalent binding of chemicals to biomolecules, thus leading to the formation of adducts.38,39 For the latter, the reaction of electrophiles with nucleophilic sites in biomolecules such as proteins and DNA is of particular importance36,40,41 and is the focus of this review. Reactive nucleophilic moieties in biomolecules that are common targets of electrophiles include S- (cysteine and glutathione) and N-centers (lysine, histidine, and amine groups in DNA bases). The covalent binding of electrophiles to nucleophiles is attributable to substitution or addition reactions that involve donation of an electron pair by the nucleophile and subsequent formation of a covalent bond. The toxicological consequences of these covalent reactions are well-established in the case of DNA adducts, which can result in chemical-induced carcinogenesis,40,42–44 making electrophilicity one of the key characteristics of carcinogens.45 Similarly, reactions of electrophiles with proteins can result in changes of the target protein function or provocation of an antigen response.32,46,47 Increased exposure to electrophiles further results in the depletion of antioxidant capacities, e.g. by reaction with glutathione, and thus indirectly leads to a toxic response due to increased oxidative stress.48,49 The abundance and diversity of potential effects makes electrophiles the largest class of known toxic chemicals.32,33,50
Assessing the reaction mechanisms of electrophiles with nucleophiles requires an understanding of the physico-chemical characteristics of both species responsible for the reactivity.51–53 According to the Hard and Soft Acid and Base (HSAB) theory,54 electrophiles react preferentially with nucleophiles of similar hardness or softness, a characteristic that has been used to predict biological targets and molecular toxicological effects.55 Hard electrophiles including alkyl carbonium ions, benzylic carbonium ions, iminium ions and aldehydes react primarily with hard nucleophiles such as endocyclic nitrogen atoms of purine bases in DNA.56 Examples of soft electrophiles include epoxides, enones, quinone imines, quinone methides, and Michael acceptors such as α,β-unsaturated aldehydes and ketones, which react with soft nucleophiles such as protein thiol groups, sulfhydryl groups of glutathione, primary/secondary amino groups of proteins, and lysine and histidine residues.42,56 In addition, some electrophiles such as α,β-unsaturated carbonyl compounds show a high reactivity with both soft and hard nucleophiles, as they contain both a hard (carbonyl carbon) and a soft (α-carbon) electrophile group.51,55,57 The HSAB theory also provides useful insights into the potential toxicity endpoints resulting from exposure to different electrophiles based on the reaction with different nucleophile pools.58 For example, weak electrophiles are more likely to form adducts with slow turnover proteins that contain activated cysteine and lysine residues and have been associated with neuro- and reproductive toxicities. In contrast, reaction of strong electrophiles with nucleic acids results in cytotoxic effects (hard–hard covalent interactions) or systemic toxicity and skin sensitization (soft–soft covalent interactions).58
Evaluating the covalent interaction of electrophiles with nucleophiles and utilizing them to assess the presence of electrophiles in environmental matrices such as drinking water also requires an in-depth understanding of the underlying reaction mechanisms. The reaction of electrophiles with nucleophilic biomolecules can be categorized into different mechanistic or reactivity domains and include Michael addition, Schiff base formation, acylation, nucleophilic aromatic substitution (SNAr) and nucleophilic substitution (SN2) (Table 1).36,59–62 Michael addition is the reaction of a nucleophile with an α,β-unsaturated carbonyl such as an α,β-unsaturated ester, ketone, or aldehyde.63–66 The effectiveness of the reaction is dependent on the α-carbon substituent and its ability to stabilize a negative charge on the carbon it is bound to.67 For example, a methyl group reduces the reactivity while electron withdrawing groups such as aldehydes, ketones, and nitro groups increase the reactivity. Schiff base formation is another important mechanistic domain of carbonyl compounds and is particularly relevant for the reaction of aliphatic aldehydes and ketones with primary amines.35 Schiff base formation is reversible via hydrolysis of the amine adduct. To stabilize the adducts before chemical analysis, reducing agents like sodium cyanoborohydride are typically used to reduce the product to a stable secondary amine.68 Aromatic electrophiles undergoing reaction with nucleophiles via SNAr reactions require the presence of electron withdrawing groups in the ortho- and/or para-position to the leaving group.69 SNAr electrophiles are reactive with both soft and hard nucleophiles and thus react with a wide spectrum of biomolecules.70 Nucleophilic substitution (SN2) reactions are particularly important for compounds containing primary alkyl groups that are bound to leaving groups such as halides71 which include DBPs such as haloacetic acids, haloacetamides, and haloacetonitriles.72–74 In contrast, the presence of a halogen substituent β to the leaving group is strongly deactivating due to adverse nonbonding interactions between the lone pairs of the halogen and the incoming nucleophile.59 This explains the low reactivity of 1,2-dichloroethane with nucleophiles. Finally, acylation is the reaction of an electrophile via transfer of an acyl moiety to a nucleophile and is relevant for esters of acidic alcohols such as phenol esters, acyl halides, and cyclic anhydrides.66
Table 1 Mechanisms of the reaction of electrophiles with nucleophilic biomolecules
Reaction mechanism |
Examples of drinking water relevant electrophiles |
Michael addition |
|
Quinones, quinone imines, unsaturated aldehydes |
Schiff base formation |
|
Saturated and unsaturated aldehydes |
Aromatic substitution (SNAr) |
|
2,4-Dinitrochlorobenzene, tetrachloroisophthalonitrile (fungicide) |
Nucleophilic substitution (SN2) |
|
Haloacetic acids, haloaceto-nitriles, haloketones |
Acylation |
|
Phthalic anhydride, phenol esters |
C Occurrence of electrophiles in drinking water
Despite the importance of electrophiles as environmental toxicants, their presence in drinking water has not been systematically evaluated. However, there is a large body of literature that has described the presence and formation of specific organic electrophiles in drinking water. The literature indicates that electrophile formation in water treatment systems utilizing chemical oxidants including chlorine, chloramine, ozone, hydroxyl radicals, and ultraviolet (UV) light is of particular relevance (Table 2). For drinking water treated by chlorination and chloramination, halogenated furanones such as MX were among the first classes of electrophilic DBPs (eDBPs) that were identified in drinking water.75,76 They contribute significantly to the mutagenicity in disinfected drinking water, especially in that containing high concentrations of humic substances.77–79 Other eDBPs commonly detected in disinfected drinking water include haloaldehydes, haloacetic acids, and haloketones. Their formation is primarily attributable to the reaction of chlorine with aliphatic and aromatic compounds.80–83 While their formation can be significantly reduced by the use of chloramination, this typically results in increased production of electrophilic nitrogenous DBPs (eN-DBPs) including haloacetamides and haloacetonitriles.84 The formation of eN-DBPs has been associated with the reaction of amino acids and other dissolved organic nitrogen compounds.84,85 More recently, halogenated quinones, in particular 2,6-dichloro-1,4-benzoquinone and 2,6-dibromo-1,4-benzoquinone, have been reported in drinking water treated with chlorine and chloramine.86,87 Their formation can most likely be explained by the presence of natural and anthropogenic compounds that contain phenolic moieties. The formation of non-halogenated benzoquinones and benzoquinone imines has also been identified in the reaction of aminophenols with chlorine.88 Another important class is aldehydes, for which elevated concentrations have been reported in chlorinated waters containing tertiary alkylamines and primary amines.89–91 If ClO2 is used as a disinfectant, aldehydes can also be formed by reaction with aromatic compounds.92 This includes the formation of α,β-unsaturated aldehydes, in particular 2-butene-1,4-dial and its chlorinated analogue chloro-2-butene-1,4-dial, which were recently identified as novel eDBPs formed when phenolic compounds react with chlorine.93
Table 2 Electrophiles previously identified in drinking water, relevant precursors (pro-electrophiles), and treatment processes leading to their formation (see Table S1 for details on the chemical structures of the electrophiles)a
Electrophile class |
Examples |
Relevant pro-electrophiles |
Formation by natural or technical processes |
Electrophile reactivity domain |
Abbreviations: AOP – advanced oxidation process; ClO2 – chlorine dioxide; DON – dissolved organic nitrogen; NOM – natural organic matter; MCA – 3,4-dichloro-5-hydroxy-2(5H)-furanone; MCF – 3-chloro-4-methyl-5-hydroxy-2(5H)-furanone; MX – 3-chloro-4-(dichloromethyl)-5-hydroxy-2(5H)-furanone; SN2 – nucleophilic substitution; UV – ultraviolet.
|
Aldehydes |
Formaldehyde, acetaldehyde, glutaraldehyde, glyoxal, methylglyoxal |
Aliphatic and aromatic compounds containing double bonds, amino acids110 |
Ozonation,96,111 AOPs,112 UV photolysis,113 chlorination,89 chloramination,89 ClO2 92 |
Schiff base |
α,β-Unsaturated aldehydes |
Acrolein, 2-butene-1,4-dial, crotonaldehyde, 3-methyl-crotonaldehyde fluorotelomer aldehydes |
Phenols, aromatics, unsaturated fatty acids93,108,114 |
Ozonation,94 AOP,93 chlorination93 |
Michael addition |
Haloaldehydes |
Chloroacetaldehyde, bromoacetaldehyde, 2-chloropropenal |
Amino acids, low molecular weight NOM, phenolics |
Chlorination8,115,116 chloramination117 |
SN2 |
Chlorinated hydroxyfuranones |
MX, MCF, MCA |
Fulvic acids, humic acids117–119 |
Chlorination,4,120,121 chloramination4,78 |
Michael addition |
Haloketones |
1,1-Dichloropropanone, 1,3-dichloropropanone and 1,1,1-trichloropropanone |
Amino acids, humic acid85,116 |
Chlorination,8,116 chloramination8,116 |
SN2 |
Haloacetonitriles |
Chloroacetonitrile, bromoacetonitrile, dichloroacetonitrile |
Phenols, DON, haloaldehydes85,122–127 |
Chlorination,8,128 chloramination8,128 |
SN2 |
Haloacetic acids |
Chloroacetic acid, bromoacetic acid, dichloroacetic acid |
Hydrophilic, neutral NOM, hydrophobic NOM83,129 |
Chlorination,4 chloramination4 |
SN2 |
Haloacetamides |
Chloroacetamide, bromoacetamide, dichloroacetamide |
Organic acids, phenols122,127,130 |
Chlorination,4,8,84 chloramination4,8,84 |
SN2 |
Quinones |
Benzoquinone, ortho-quinone, 2,6-dichlorobenzoquinone |
Aromatic compounds, phenols, aromatic amines88,109,131,132 |
Chlorination,88 ozonation,101 AOPs109,133 |
Michael addition |
Quinone imines |
Benzoquinone imine, N-acetyl-p-benzoquinone imine |
Aromatic amines88,104 |
Ozonation,104 chlorination88 |
Michael addition |
Epoxides |
Carbamazepine epoxide, oxirane, methyloxirane |
Unsaturated aliphatic and aromatic compounds134–136 |
Ozonation,134,137,138 chlorination,134,139 chloramination134 |
SN2 |
Cyclic anhydrides |
Phthalic anhydride, maleic anhydride |
Azo dyes, hydroxylated aromatics140,141 |
Ozonation,141 AOPs140,142–144 |
Acylation |
Organo-phosphorus esters |
Chlorpyrifos oxon, diazinon oxon, malaoxon, parathion oxon |
Thiophosphates such as chlorpyrifos, malathion, and parathion145,146 |
Chlorination,145 photolysis,147 hydrolysis,148 ozonation146 |
SN2 |
Cyanogen halides |
Cyanogen chloride, cyanogen bromide |
Aliphatic amino acids, formaldehyde149,150 |
Chloramination,150,151 chlorination152 |
SN2 |
Hydro-peroxides |
Hydroxymethyl hydroperoxide, l-hydroxyethyl hydroperoxide |
Unsaturated alkyl compounds, thymidine, vinyl chloride107,137,153 |
Ozonation107,137,153 |
Unstable, form radicals and epoxides |
In addition to chlorine-based disinfection, aldehydes are one of the most important eDBPs in drinking water treatment using ozone and hydroxyl radicals. Of particular relevance are low molecular weight saturated aldehydes, including acetaldehyde, formaldehyde, glyoxal, and methylglyoxal, which have been detected in concentrations in the low to medium μg L−1-range in treated waters.94–96 Relevant precursors of aldehydes formed in ozonation and advanced oxidation processes include aromatic compounds, amino acids, and unsaturated fatty acids.97–99 Formation of quinones has been observed during ozonation and advanced oxidation processes with known precursors, including aromatic compounds, phenols, and azo dyes.100,101 Azo dyes are also important precursors of quinone imines, as are anilines and other phenolic compounds containing nitrogen substituents in the para- or ortho-position.102–104 Other classes of electrophiles likely to be formed during oxidative water treatment include peroxides, epoxides, and α,β-unsaturated aldehydes.31 Information about their presence in drinking water, however, is still widely lacking. In laboratory experiments, the formation of hydroperoxides has been observed for the reaction of ozone with unsaturated fatty acids, alkenes, and nucleic acids such as thymine and thymidine.105–107 Prasse et al. recently demonstrated the formation of α,β-unsaturated aldehydes when phenols react with hydroxyl radicals and/or UV light.108 The reaction of benzene and other monoaromatic compounds with hydroxyl radicals can further result in the formation of C6-α,β-unsaturated aldehydes.109 The results demonstrate the relevance of these electrophiles and highlight the importance of developing approaches to detect them in the environment and drinking water.
D Reactivity-directed analysis for the assessment of exposure to organic electrophiles
Despite the widespread occurrence of electrophiles in drinking water, their detection in complex environmental mixtures is challenging. This is particularly true for small, highly polar, and reactive electrophiles. Addressing this limitation requires the rethinking of the analytical approaches currently in use. This rethinking is necessary, too, if we want to move beyond the detection of individual, known electrophiles to investigate the formation and presence of unknown electrophiles.
In toxicology and biochemistry, novel analytical approaches have emerged to specifically identify individual electrophiles (Table 3).34,154 Initially, these methods were predominantly used in combination with in vitro-based approaches to elucidate adverse drug reactions facilitated by electrophilic metabolites.155–161 In addition, a large number of studies have focused on the identification of electrophiles used in cosmetics or associated with occupational exposure due to their importance as skin and respiratory sensitizers.65,162 Originally, these assessments were performed using animal models. Recognition of electrophile and nucleophilic biomolecule covalent reactions as critical MIEs in the adverse outcome pathways of these toxicity endpoints led to the development of alternative strategies to simulate these interactions via in chemico assays.163–168 In contrast to in vivo and in vitro assays, in chemico assays are used to investigate the interactions of chemicals with biomolecules in the absence of cells or organisms. In this review, the term Reactivity-Directed Analysis (RDA) is introduced, which encompasses existing in chemico assays as well as novel approaches to identify unknown electrophiles in complex environmental mixtures such as drinking water.
Table 3 Overview of existing RDA approaches used for the in chemico assessment of electrophiles, their main function, and their benefits and limitationsa
RDA approach |
Analytical detection method |
Benefits and limitations |
Abbreviations: ADRA – amino acid derivative reactivity assay; Cor1C420 – peptide derived from the sequence around Cys420 in the human Coronin1 protein; DCYA – dansylcysteamine; DPRA – direct peptide reactivity assay; EASA – electrophilic allergen screening assay; HPLC – high performance liquid chromatography; HTS – high-throughput screening; NMR – nuclear magnetic resonance; LC – liquid chromatography; MS – mass spectrometry; PPRA – peroxidase peptide reactivity assay; RDA – reactivity directed analysis; UV – ultraviolet.
|
ADRA |
HPLC-UV |
Naphthalene modified amino acids allow for higher sensitivity compared to DPRA; no information on adducts; requires high concentrations of electrophiles |
ADRA-DM |
LC-MS/MS |
Use of LC-MS/MS enables the use of lower concentrations of electrophiles; provides information on both nucleophile depletion and adduct formation |
Cor1C420 |
LC-MS |
Contains multiple electrophiles; detection of both depletion and adduct formation |
DPRA |
HPLC-UV |
Requires high concentrations of electrophiles; only addresses depletion of peptides |
EASA |
HPLC-UV and HPLC-fluorescence |
Use of nitrobenzenethiol and pyridoxylamine nucleophile probes; only addresses depletion of the nucleophilic probe |
HTS-DCYA |
Fluorescence microplate reader |
Allows for high-throughput processing of samples; no information on the chemical structure of adducts; potential interference by nucleophile dimerization (relevant for cysteine) |
NMR-DCYA |
NMR |
NMR allows detailed structural insights into adducts; work intensive as DCYA adducts have to be purified or synthesized in high quantities prior to NMR analysis |
Photo-DPRA |
HPLC-UV |
Requires high concentrations of electrophiles; can be used to study both direct and indirect photolytic formation of electrophiles; no information on the chemical structure of adducts |
Photo-ADRA |
HPLC-fluorescence |
Higher sensitivity compared to photo-DPRA; no information on the chemical structure of adducts |
PPRA |
LC-MS/MS |
Metabolic activation peroxidase has a low substrate specificity; limited to compounds that can be degraded by horseradish peroxidase |
Existing RDA approaches can be distinguished based on the type of molecule and the analytical techniques used to elucidate the reaction of electrophiles with nucleophilic targets. Most approaches focus on the identification of adducts with proteins, peptides, or amino acids.41,169,170 In contrast, they are less commonly used to assess reactions with DNA. The formation of DNA adducts themselves is a poor predictor of toxicity, in particular carcinogenicity, because the adducts have to be converted into mutations, which is dependent on whether they are recognized by cellular repair mechanisms.171 Thus, this review only discusses RDA approaches focusing on proteins and protein subunits, i.e., amino acids and peptides. It should be noted, however, that most protein reactive chemicals are also likely to react with DNA.172,173
The development of RDA approaches has been largely driven by efforts to develop new screening approaches to assess the skin and respiratory sensitization potential of chemicals. Up to now, more than 4,000 skin sensitizing compounds have been identified, and two-thirds of these are organic electrophiles.67,174,175 The direct peptide reactivity assay (DPRA)176 is the most widely used assay and has been approved by the Organization for Economic Co-operation and Development as an alternative to conventional animal testing methods.177 The DPRA uses synthetic peptides containing either lysine or cysteine to evaluate the reaction of individual organic chemicals with nucleophilic biomolecules.168,176 In this assay, the sensitizers are added in 10-fold excess to either a cysteine or lysine model peptide, and the sensitizer potency is evaluated based on the peptide depletion after 24 h of incubation at room temperature detected via high performance liquid chromatography-UV detection (HPLC-UV). To also determine the chemical kinetics of electrophile–nucleophile reactions, the high-throughput kinetic profiling (HTKP) assay178 and the electrophilic allergen screening assay (EASA) were developed.179,180 This EASA, which is currently undergoing evaluation by the National Toxicology Program, uses stopped-flow techniques and nitrobenzenethiol and pyridoxylamine as cysteine and lysine substitutes, respectively. While the previously mentioned RDA approaches primarily assessed the depletion of nucleophiles using HPLC-UV analysis, the low sensitivity of the methods requires the use of high concentrations of both test chemicals and nucleophiles, thus limiting the applicability of these methods for hydrophobic compounds. To address this issue, alternative approaches providing a higher sensitivity have been developed. The amino acid derivative reactivity assay (ADRA)181 uses naphthalene-modified lysine and cysteine amino acids which can be analyzed by HPLC-UV at 281 nm and significantly increases its sensitivity compared to the DPRA assay. The same is true when fluorescence-labelled amino acids are used.182,183 To enable the testing of large numbers of suspected electrophiles, high-throughput approaches have been developed. The high-throughput screening dansylcysteamine (HTS-DCYA) assay184 allows for the direct quantification of dansylcysteamine adducts by fluorescence detection in multi-well microplates. In this assay, the dosing of electrophiles is followed by addition of silica-supported maleimide, which reacts with the remaining, unreacted DCYA. Subsequently, the solutions are centrifuged, and the supernatants containing only the DCYA electrophile adducts are transferred into 96-well plates for fluorescence analysis via a microplate reader.
Although detection via UV or fluorescence allows for the quantification of nucleophile depletion, it does not provide any information on the identity of the formed adducts. To address this limitation, LC-MS and nuclear magnetic resonance (NMR) spectroscopy can be applied to simultaneously determine peptide depletion, peptide oxidation (e.g. dimerization of cysteine), and adduct formation.185–187 The high sensitivity of LC-MS compared to HPLC-UV also allows the use of lower concentrations of both electrophiles and nucleophiles. The ADRA-dilutional method (ADRA-DM) assay developed by Yamamoto et al. uses 100-fold lower concentrations than those used by the DPRA or ADRA, which is particularly advantageous for compounds with low water solubility.188 In addition to the RDA approaches that use individual nucleophilic targets, investigations have also focused on the use of peptides containing multiple nucleophilic sites. The Cor1C420 kinetic peptide reactivity assay186,189,190 uses a peptide derived from the sequence around Cys420 in the human Coronin1 protein, which shows a high reactivity toward electrophiles, as it contains two lysine residues at the N-terminal side of the cysteine residue.191 Ahlfors et al. developed an assay that uses the synthetic model peptide containing essentially all nucleophilic amino acids (H-Pro-His-Cys-Lys-Arg-Met-OH) followed by identification of adduct structures via NMR and LC-MS.187 This allows for the assessment of the relative affinity of electrophiles to different nucleophiles.
To also address the relevance of biotic and abiotic transformation processes leading to the formation of electrophiles, studies have also focused on the development of assays to identify precursors of electrophiles, the so-called pro-electrophiles. These assays include the peroxidase peptide reactivity assay (PPRA) that uses a horseradish peroxidase and hydrogen peroxide system.164,192,193 The active site of HRP is accessible by structurally diverse pro-electrophiles, making it ideal for screening purposes. Examples of relevant pro-electrophiles include aniline, 2-amino-phenol, 3-methylcatechol, and 2-methoxy-4-methylphenol, which are activated to benzoquinone and quinone imine electrophiles.65,194 The relevance of sensitization reactions caused by the degradation of skin care product ingredients has led to the development of the photo-DPRA and photo-ADRA to address the formation of electrophilic transformation products by exposure to sunlight.195,196 To investigate the formation of electrophiles during the photodegradation of various commonly used organic UV-filters, Stiefel et al. developed a high performance thin-layer chromatography-based assay.197 Exposure of UV-filters to both artificial and natural sunlight revealed the formation of electrophiles for ketone-containing UV filters such as benzophenone-3 and 4-t-butyl-4′-methoxydibenzoylmethane. Similar results were observed for the photodegradation of UV-filters and identification of electrophiles via reaction with lysine-containing model peptides and bovine serum albumin.198 In addition, the photo-DPRA has also been used to investigate the photosensitization of glyphosate and glyphosate-containing agrochemical formulations.199
E Application of reactivity-directed analysis in the analysis of electrophiles in drinking water
The existing studies on the application of RDA demonstrate the general applicability of this new methodology to the identification of electrophiles. For the assessment of drinking water relevant contaminants, Pals et al. used a thiol reactivity assay to determine the toxicity of brominated DBPs including bromoacetic acid, bromoacetonitrile, and bromoacetamide.72,200 The authors observed a high correlation with an in vitro genotoxicity assay performed in parallel. Similar results were obtained by Wei et al., who investigated the relative toxicity of haloacetonitriles using mammalian cell cytotoxicity, genotoxicity, and a thiol reactivity assay.201 Boehme et al. used a kinetic glutathione assay to determine the thiol reactivity of α,β-unsaturated ketones, acrylates, and propiolates.202 The authors observed a wide range of reaction rates spanning over 5 orders of magnitude. In addition, a high correlation (r2 = 0.91) was observed between the measured rate constants and the toxicity toward the ciliates Tetrahymena pyriformis, thus demonstrating that the toxicity of these compounds is driven by their electrophilic reactivity. A similar approach was used by Roberts et al. to assess the thiol reactivity of 60 haloaliphatic compounds.71 The highest correlation (r2 = 0.889) with acute aquatic toxicity (IG50) to Tetrahymena pyriformis was observed for compounds in which a primary halogen is α to a carbonyl or other electronegative unsaturated group (SN2 electrophiles).
In addition to the assessment of individual (drinking) water contaminants, recent efforts have also focused on the assessment of the presence of electrophiles in different environmental matrices. Dong et al. used a plate reader-based assay with N-acetyl-cysteine as a nucleophilic probe to evaluate the thiol reactivity of different water samples, including tap water and wastewater.203,204 Thiol reactivity differed significantly between the different samples and was highest in treated wastewater and lowest in tap water and water from a direct potable reuse system.204 Furthermore, the authors demonstrated a high correlation between thiol reactivity and mammalian genotoxicity as observed using Chinese hamster ovary cells, which indicated the suitability of this method to assess the toxicity of environmental mixtures. Using the same approach, Dong et al. also determined the toxicity of wastewater amended with Br− and I− during subsequent treatment with ozone, chlorine, or chloramine.205 Significantly higher thiol reactivity was observed in chlorinated and chloraminated wastewater, while the ozonated wastewater showed a similar reactivity to the untreated control. According to the authors, this can be explained by the formation of ozonation products that are less toxic than the DBPs formed in chlorination and chloramination. However, no attempts were made to elucidate the electrophiles responsible for the observed thiol reactivity. In addition, all previously mentioned studies used an extraction step based on XAD resins to concentrate the electrophiles prior to their toxicity assessment. As such, the lower thiol reactivity observed for some of the samples, in particular ozonated wastewater, could potentially be attributed to the loss of low molecular weight and/or highly polar compounds such as aldehydes during the extraction step. Preconcentration using XAD resins was also used by Cheh et al. for the detection of alkyl halides and epoxides in drinking water via their reaction with the nucleophilic probe compounds 4-nitrothiophenol and 4-nitrobenzylpyridine.206 The authors further investigated the impacts of the addition of different amounts of these nucleophiles on the mutagenicity of the extracted drinking water sample. They observed a decrease in toxicity with increasing amounts of nucleophiles, which again demonstrated the importance of electrophiles as contributors to the toxic effects of these environmental mixtures.
Another promising application of RDA is the identification of toxic electrophiles formed during the oxidative treatment of individual drinking water contaminants in simulated laboratory studies. Prasse et al. investigated the formation of electrophilic transformation products during the degradation of phenolic compounds by UV light, hydroxyl radicals, and HOCl.93,108 Formation of electrophiles was investigated using N-α-acetyl lysine and N-acetyl cysteine as nucleophilic probes. The identity of electrophile adducts with both amino acids was elucidated using high-resolution mass spectrometry. The results of experiments with phenol revealed the formation of the highly toxic dialdehyde 2-butene-1,4-dial. The toxicity of this compound was further studied using chemoproteomics, which revealed the reaction of 2-butene-1,4-dial with several proteins using mouse liver proteome.108 Experiments with other substituted phenolic compounds revealed that dicarbonyl compounds are common transformation products of hydroxyl radicals with this class of chemicals. Most importantly, this was the first time that these compounds were detected in aqueous environments, which can be attributed to the inadequacy of conventional approaches like GC-MS and LC-MS. As such, RDA offers an opportunity to identify electrophiles that are intrinsically challenging to detect. In this context, nucleophilic biomolecules or their analogues function as derivatization agents, thus enabling the indirect identification of electrophiles via analysis of nucleophile adducts.
F Future research needs
RDA represents a novel approach with the potential to substantially expand our knowledge about the presence and formation of toxic contaminants in drinking water and other aqueous matrices. As discussed in this review, electrophiles are commonly detected in drinking water, and their relevance will rise in the future due to the increased application of oxidative treatment technologies. This increase is contrasted by the limited availability of analytical methods to comprehensively assess the presence of electrophiles in drinking water. This is particularly true for electrophiles characterized by low molecular weight, high polarity, and low stability, as they are likely to be lost during current sample work-up procedures and difficult to directly detect by conventional analytical approaches such as LC- and GC-high-resolution mass spectrometry. Even though the environmental applications of RDA are still limited, the escalating importance of this approach for chemical risk assessment will likely contribute to the expansion of this technology toward the analysis of complex mixtures and assessment of environmental exposure.207–209 In addition to electrophile detection in drinking water and reactive organic electrophile identification in (oxidative) drinking water treatment processes, RDA also offers new approaches to advance our understanding of the impacts of drinking water contaminants on human health. It provides a new tool to identify chemicals that are of particular health concern and that should be prioritized in future toxicity testing efforts that use in vitro and in vivo assays.
In addition, RDA can also support epidemiological studies that focus on the health impacts of drinking water exposure. Existing data strongly suggest an association between the presence of specific drinking water contaminants, in particular THMs, and health outcomes such as bladder cancer.11,210 It is currently unknown, however, whether this association is indeed attributable to THMs or whether other co-occurring compounds are responsible, considering that the vast majority of drinking water contaminants (including DBPs) are not (or not widely) monitored. For skin cancer, exposure to drinking water contaminants has been associated with the presence of THMs, which are metabolized by skin enzymes to toxic intermediates.10 Thus, RDA could provide novel insights into the formation of electrophilic intermediates that lead to adverse health outcomes, e.g., in controlled laboratory experiments simulating these metabolic processes, and help to elucidate the toxic mode of action. RDA can also help identify the chemicals responsible for dermal and respiratory diseases associated with drinking water exposure.13 As discussed before, electrophiles represent the majority of (skin) sensitizers that are responsible for chemically induced allergic reactions. Known electrophilic sensitizers are characterized by molecular weights <500 Da,211,212 which is also likely to be the case for electrophiles formed during oxidative drinking water treatment. As such, organic electrophiles are likely candidates responsible for these effects, and RDA represents an ideal platform to identify the relevant toxicants.
Despite the advantages of RDA as a novel tool to determine the presence and formation of organic electrophiles in drinking water, a number of challenges need to be addressed before the approach can be more widely applied. These include the detection of electrophiles at concentrations relevant for drinking water, the stability of nucleophilic probes—in particular thiol-containing compounds—and the stability of specific nucleophile adducts. Similar to other analytical approaches, the low concentrations of most drinking water contaminants represent a major challenge for their analysis. Existing strategies that use additional pre-concentration steps like SPE or resins like XAD are not suitable for the extraction of most electrophiles, as they are not sufficiently retained and/or reactive with the sorbents. For RDA, this issue could be addressed by direct addition of nucleophilic probe compounds to the (drinking) water samples (Fig. 1). This step would then be followed by extraction of the nucleophile adducts using conventional approaches such as SPE. Modified nucleophilic probes containing hydrophobic moieties, similar to those used to enhance their detection by UV or fluorescence, could be utilized to enhance the extraction efficacies. On the other hand, this approach would require the addition of large amounts of nucleophiles to reach sufficient nucleophile concentrations in the solutions. For the subsequent analysis of adducts, the low concentrations of electrophiles in drinking water might also be challenging due to the high concentrations of unmodified nucleophilic probes in the sample extracts. As such, an additional sample pre-treatment step might be required to separate the modified nucleophiles from the unmodified nucleophiles. Another challenge is the potential auto-oxidation of nucleophiles, in particular thiols, by residual chemical oxidants and trace metals in drinking water samples. Thiols present in cysteines are easily oxidized and form disulfides, which can significantly impact the availability of nucleophiles as well as their analysis.213,214 To address this issue, additives such as ethylenediaminetetraacetic acid can be added to complex trace metals and reducing agents such as tris(2-carboxyethyl)phosphine can be added to reduce oxidized thiol species back to their reduced monomeric form.182 Similarly, sodium cyanoborohyride can be used to stabilize Schiff base adducts.68
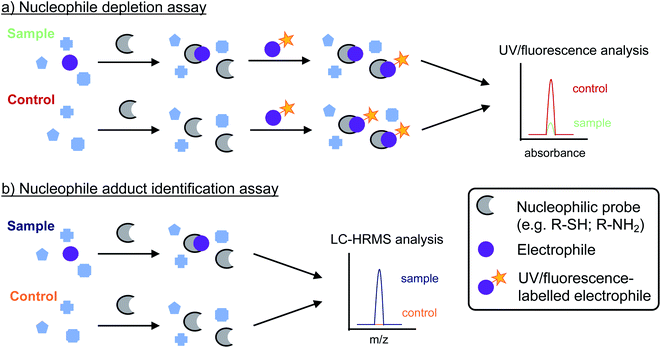 |
| Fig. 1 Strategies for the identification of electrophiles in drinking water using RDA. The nucleophile depletion assay (a) allows for the general assessment of the presence of electrophiles in drinking water, while the nucleophile adduct identification assay (b) can be used to detect individual nucleophile adducts which can then be used to identify the relevant electrophiles responsible for the adduct formation. | |
Finally, the exposure time of the reaction between nucleophilic probe compounds and organic electrophiles needs to be considered. The reaction kinetics of electrophiles with nucleophiles vary substantially and span several orders of magnitude.215,216 While nucleophiles are generally added in a large excess to prevent their complete depletion, shorter exposure times will result in bias toward highly reactive electrophiles, while longer exposure times would be required for slower reacting compounds. However, this could potentially be used to prioritize electrophiles likely to be of the highest concern as the electrophilic reactivity is highly correlated with toxicity.217
Conflicts of interest
There are no conflicts to declare.
Acknowledgements
I want to thank Daisy Grace, Veronica Wallace, Casey Smith and Jessica Goddard for valuable comments and edits on the draft and Daisy Grace for the design of the TOC art. I am grateful to all the incredible researchers who have inspired and influenced my research. I further acknowledge financial support by Johns Hopkins University.
References
- E. Drakvik, R. Altenburger, Y. Aoki, T. Backhaus, T. Bahadori, R. Barouki, W. Brack, M. T. D. Cronin, B. Demeneix, S. Hougaard Bennekou, J. van Klaveren, C. Kneuer, M. Kolossa-Gehring, E. Lebret, L. Posthuma, L. Reiber, C. Rider, J. Rüegg, G. Testa, B. van der Burg, H. van der Voet, A. M. Warhurst, B. van de Water, K. Yamazaki, M. Öberg and Å. Bergman, Statement on advancing the assessment of chemical mixtures and their risks for human health and the environment, Environ. Int., 2020, 134, 105267 CrossRef CAS.
- B. I. Escher, H. M. Stapleton and E. L. Schymanski, Tracking Complex Mixtures in Our Changing Environment, Science, 2020, 367, 388–392 CrossRef CAS.
-
National Cancer Institute, Report on the Carcinogenesis Bioassay of Chloroform (CAS No. 67-66-3), TR-000 NTIS Rpt No. PB264018, Bethesda, MD, Bethesda, MD, 1976 Search PubMed.
- S. D. Richardson, M. J. Plewa, E. D. Wagner, R. Schoeny and D. M. Demarini, Occurrence, genotoxicity, and carcinogenicity of regulated and emerging disinfection by-products in drinking water: a review and roadmap for research, Mutat. Res., 2007, 636, 178–242 CAS.
- U. von Gunten, Oxidation Processes in Water Treatment: Are We on Track?, Environ. Sci. Technol., 2018, 52, 5062–5075 CrossRef CAS.
- C. Postigo and S. D. Richardson, Transformation of pharmaceuticals during oxidation/disinfection processes in drinking water treatment, J. Hazard. Mater., 2014, 279, 461–475 CrossRef CAS.
- U. Hübner, B. Seiwert, T. Reemtsma and M. Jekel, Ozonation products of carbamazepine and their removal from secondary effluents by soil aquifer treatment – indications from column experiments, Water Res., 2014, 49, 34–43 CrossRef.
- S. W. Krasner, H. S. Weinberg, S. D. Richardson, S. J. Pastor, R. Chinn, M. J. Sclimenti, G. D. Onstad and A. D. Thruston, Occurrence of a new generation of disinfection byproducts, Environ. Sci. Technol., 2006, 40, 7175–7185 CrossRef CAS.
- S. D. Richardson and M. J. Plewa, To regulate or not to regulate? What to do with more toxic disinfection by-products?, J. Environ. Chem. Eng., 2020, 8, 103939 CrossRef CAS.
- M. R. Karagas, C. M. Villanueva, M. Nieuwenhuijsen, C. P. Weisel, K. P. Cantor and M. Kogevinas, Disinfection byproducts in drinking water and skin cancer? A hypothesis, Cancer Causes Control, 2008, 19, 547–548 CrossRef.
- C. M. Villanueva, S. Cordier, L. Font-Ribera, L. A. Salas and P. Levallois, Overview of disinfection by-products and associated health effects, Curr. Environ. Health Rep., 2015, 2, 107–115 CrossRef CAS.
- C. M. Villanueva, K. P. Cantor, J. O. Grimalt, N. Malats, D. Silverman, A. Tardon, R. Garcia-Closas, C. Serra, A. Carrato, G. Castaño-Vinyals, R. Marcos, N. Rothman, F. X. Real, M. Dosemeci and M. Kogevinas, Bladder cancer and exposure to water disinfection by-products through ingestion, bathing, showering, and swimming in pools, Am. J. Epidemiol., 2007, 165, 148–156 CrossRef.
- J. M. Weintraub, M. Berger and R. Bhatia, Heterogeneous dermatitis complaints after change in drinking water treatment: A case report, Environ. Health: Global Access Sci. Source, 2006, 5, 4–6 CrossRef.
- A. Lazarov, K. Nevo, A. Pardo and P. Froom, Self-reported skin disease in hydrotherapists working in swimming pools, Contact Dermatitis, 2005, 53, 327–331 CrossRef.
- K. M. Thickett, J. S. McCoach, J. M. Gerber, S. Sadhra and P. S. Burge, Occupational asthma caused by chloramines in indoor swimming-pool air, Eur. Respir. J., 2002, 19, 827–832 CrossRef CAS.
- R. S. Judson, K. A. Houck, R. J. Kavlock, T. B. Knudsen, M. T. Martin, H. M. Mortensen, D. M. Reif, D. M. Rotroff, I. Shah, A. M. Richard and D. J. Dix, In vitro screening of environmental chemicals for targeted testing prioritization: the ToxCast project, Environ. Health Perspect., 2010, 118, 485–492 CrossRef CAS.
- R. Kavlock, K. Chandler, K. Houck, S. Hunter, R. Judson, N. Kleinstreuer, T. Knudsen, M. Martin, S. Padilla, D. Reif, A. Richard, D. Rotroff, N. Sipes and D. Dix, Update on EPA's ToxCast program: providing high throughput decision support tools for chemical risk management, Chem. Res. Toxicol., 2012, 25, 1287–1302 Search PubMed.
- C. Rivetti, T. E. H. Allen, J. B. Brown, E. Butler, P. L. Carmichael, J. K. Colbourne, M. Dent, F. Falciani, L. Gunnarsson, S. Gutsell, J. A. Harrill, G. Hodges, P. Jennings, R. Judson, A. Kienzler, L. Margiotta-Casaluci, I. Muller, S. F. Owen, C. Rendal, P. J. Russell, S. Scott, F. Sewell, I. Shah, I. Sorrel, M. R. Viant, C. Westmoreland, A. White and B. Campos, Vision of a near future: Bridging the human health–environment divide. Toward an integrated strategy to understand mechanisms across species for chemical safety assessment, Toxicol. In Vitro, 2020, 62, 104692 CrossRef.
- B. I. Escher, L. Glauch, M. König, P. Mayer and R. Schlichting, Baseline toxicity and volatility cutoff in reporter gene assays used for high-throughput screening, Chem. Res. Toxicol., 2019, 32, 1646–1655 Search PubMed.
- G. L. Ginsberg, K. P. Fedinick, G. M. Solomon, K. C. Elliott, J. J. Vandenberg, S. Barone and J. R. Bucher, New toxicology tools and the emerging paradigm shift in environmental health decision-making, Environ. Health Perspect., 2019, 127, 1–7 CrossRef.
- T. A. Ternes, C. Prasse, C. Lu, G. Knopp, P. Cornel, U. Schulte-Oehlmann, T. Schwartz, J. Alexander, W. Seitz, A. Coors and J. Oehlmann, Integrated evaluation concept to assess the efficacy of advanced wastewater treatment processes for the elimination of micropollutants and pathogens, Environ. Sci. Technol., 2017, 51, 308–319 CrossRef CAS.
- A. M. Brunner, C. Bertelkamp, M. M. L. Dingemans, A. Kolkman, B. Wols, D. Harmsen, W. Siegers, B. J. Martijn, W. A. Oorthuizen and T. L. ter Laak, Integration of target analyses, non-target screening and effect-based monitoring to assess OMP related water quality changes in drinking water treatment, Sci. Total Environ., 2020, 705, 135779 CrossRef CAS.
- R. Altenburger, W. Brack, R. M. Burgess, W. Busch, B. I. Escher, A. Focks, L. Mark Hewitt, B. N. Jacobsen, M. L. de Alda, S. Ait-Aissa, T. Backhaus, A. Ginebreda, K. Hilscherová, J. Hollender, H. Hollert, P. A. Neale, T. Schulze, E. L. Schymanski, I. Teodorovic, A. J. Tindall, G. de Aragão Umbuzeiro, B. Vrana, B. Zonja and M. Krauss, Future water quality monitoring: improving the balance between exposure and toxicity assessments of real-world pollutant mixtures, Environ. Sci. Eur., 2019, 31, 1–17 CrossRef.
- C. Prasse, D. Stalter, U. Schulte-Oehlmann, J. Oehlmann and T. A. Ternes, Spoilt for choice: A critical review on the chemical and biological assessment of current wastewater treatment technologies, Water Res., 2015, 87, 237–270 CrossRef CAS.
- A. Abbas, I. Schneider, A. Bollmann, J. Funke, C. Prasse, U. Schulte-Oehlmann, W. Seitz, T. Ternes, M. Weber, H. Wesely and M. Wagner, What you extract is what you see: Optimising the preparation of water and wastewater samples for in vitro bioassays, Water Res., 2019, 152, 47–60 CrossRef CAS.
- J. Han and X. Zhang, Evaluating the comparative toxicity of DBP mixtures from different disinfection scenarios: a new approach by combining freeze-drying or rotoevaporation with a marine polychaete bioassay, Environ. Sci. Technol., 2018, 52, 10552–10561 CrossRef CAS.
- W. Brack, Effect-directed analysis: A promising tool for the identification of organic toxicants in complex mixtures?, Anal. Bioanal. Chem., 2003, 377, 397–407 CrossRef CAS.
- W. Brack, S. Ait-Aissa, R. M. Burgess, W. Busch, N. Creusot, C. Di Paolo, B. I. Escher, L. Mark Hewitt, K. Hilscherova, J. Hollender, H. Hollert, W. Jonker, J. Kool, M. Lamoree, M. Muschket, S. Neumann, P. Rostkowski, C. Ruttkies, J. Schollee, E. L. Schymanski, T. Schulze, T. B. Seiler, A. J. Tindall, G. De Aragão Umbuzeiro, B. Vrana and M. Krauss, Effect-directed analysis supporting monitoring of aquatic environments – an in-depth overview, Sci. Total Environ., 2016, 544, 1073–1118 CrossRef CAS.
- F. Hernández, J. Bakker, L. Bijlsma, J. de Boer, A. M. Botero-Coy, Y. Bruinen de Bruin, S. Fischer, J. Hollender, B. Kasprzyk-Hordern, M. Lamoree, F. J. López, T. L. te. Laak, J. A. van Leerdam, J. V. Sancho, E. L. Schymanski, P. de Voogt and E. A. Hogendoorn, The role of analytical chemistry in exposure science: Focus on the aquatic environment, Chemosphere, 2019, 222, 564–583 CrossRef.
- N. Zwart, S. L. Nio, C. J. Houtman, J. De Boer, J. Kool, T. Hamers and M. H. Lamoree, High-throughput effect-directed analysis using downscaled in vitro reporter gene assays to identify endocrine disruptors in surface water, Environ. Sci. Technol., 2018, 52, 4367–4377 CrossRef CAS.
- W. H. Glaze, Drinking-water treatment with ozone. Ozone is a powerful disinfectant and oxidant, but its chemical byproducts need to be better understood, Environ. Sci. Technol., 1987, 21, 224–230 CrossRef CAS.
- D. C. Liebler, Protein damage by reactive electrophiles: Targets and consequences, Chem. Res. Toxicol., 2008, 21, 117–128 Search PubMed.
- S. M. Rappaport and M. T. Smith, Environment and disease risks, Science, 2010, 330, 460–461 CrossRef CAS.
- M. T. D. Cronin, F. Bajot, S. J. Enoch, J. C. Madden, D. W. Roberts and J. Schwöbel, The in chemico-in silico interface: Challenges for integrating experimental and computational chemistry to identify toxicity, ATLA, Altern. Lab. Anim., 2009, 37, 513–521 CrossRef CAS.
- R. M. Lopachin and T. Gavin, Molecular mechanisms of aldehyde toxicity: A chemical perspective, Chem. Res. Toxicol., 2014, 27, 1081–1091 Search PubMed.
- S. J. Enoch and M. T. D. Cronin, A review of the electrophilic reaction chemistry involved in covalent DNA binding, Crit. Rev. Toxicol., 2010, 40, 728–748 CrossRef CAS.
- G. T. Ankley, R. S. Bennett, R. J. Erickson, D. J. Hoff, M. W. Hornung, R. D. Johnson, D. R. Mount, J. W. Nichols, C. L. Russom, P. K. Schmieder, J. A. Serrrano, J. E. Tietge and D. L. Villeneuve, Adverse outcome pathways: A conceptual framework to support ecotoxicology research and risk assessment, Environ. Toxicol. Chem., 2010, 29, 730–741 CrossRef CAS.
- T. E. H. Allen, J. M. Goodman, S. Gutsell and P. J. Russell, Defining molecular initiating events in the adverse outcome pathway framework for risk assessment, Chem. Res. Toxicol., 2014, 27, 2100–2112 Search PubMed.
- T. E. H. Allen, J. M. Goodman, S. Gutsell and P. J. Russell, Using 2D structural alerts to define chemical categories for molecular initiating events, Toxicol. Sci., 2018, 165, 213–223 CrossRef CAS.
- S. Balbo, R. J. Turesky and P. W. Villalta, DNA adductomics, Chem. Res. Toxicol., 2014, 27, 356–366 Search PubMed.
- S. M. Rappaport, H. Li, H. Grigoryan, W. E. Funk and E. R. Williams, Adductomics: Characterizing exposures to reactive electrophiles, Toxicol. Lett., 2012, 213, 83–90 CrossRef CAS.
- S. J. Enoch and M. T. D. Cronin, Development of new structural alerts suitable for chemical category formation for assigning covalent and non-covalent mechanisms relevant to DNA binding, Mutat. Res., Genet. Toxicol. Environ. Mutagen., 2012, 743, 10–19 CrossRef CAS.
- E. C. Miller and J. A. Miller, Searches for ultimate chemical carcinogens and their reactions with cellular macromolecules, Cancer, 1981, 47, 2327–2345 CrossRef CAS.
- R. Benigni and C. Bossa, Mechanisms of chemical carcinogenicity and mutagenicity: a review with implications for predictive toxicology, Chem. Rev., 2011, 111, 2507–2536 CrossRef CAS.
- M. T. Smith, K. Z. Guyton, N. Kleinstreuer, A. Borrel, A. Cardenas, W. A. Chiu, D. W. Felsher, C. F. Gibbons, W. H. Goodson, K. A. Houck, A. Kane, M. A. La Merrill, H. Lebrec, L. Lowe, C. M. McHale, S. Minocherhomji, L. Rieswijk, M. S. Sandy, H. Sone, A. Wang, L. Zhang, L. Zeise and M. Fielden, The Key Characteristics of Carcinogens: Relationship to the Hallmarks of Cancer, Relevant Biomarkers, and Assays to Measure Them, Cancer Epidemiol., Biomarkers Prev., 2020, 29, 1887–1903 CrossRef CAS.
- R. M. LoPachin and A. P. DeCaprio, Protein adduct formation as a molecular mechanism in neurotoxicity, Toxicol. Sci., 2005, 86, 214–225 CrossRef CAS.
- J. Gan, H. Zhang and W. G. Humphreys, Drug-protein adducts: chemistry, mechanisms of toxicity, and methods of characterization, Chem. Res. Toxicol., 2016, 29, 2040–2057 Search PubMed.
- J. Limón-Pacheco and M. E. Gonsebatt, The role of antioxidants and antioxidant-related enzymes in protective responses to environmentally induced oxidative stress, Mutat. Res., Genet. Toxicol. Environ. Mutagen., 2009, 674, 137–147 CrossRef.
- R. Franco, R. Sánchez-Olea, E. M. Reyes-Reyes and M. I. Panayiotidis, Environmental toxicity, oxidative stress and apoptosis: Ménage à Trois, Mutat. Res., Genet. Toxicol. Environ. Mutagen., 2009, 674, 3–22 CrossRef CAS.
- Y. Kumagai and Y. Abiko, Environmental Electrophiles: Protein Adducts, Modulation of Redox Signaling, and Interaction with Persulfides/Polysulfides, Chem. Res. Toxicol., 2017, 30, 203–219 Search PubMed.
- R. M. LoPachin, B. C. Geohagen and L. U. Nordstroem, Mechanisms of soft and hard electrophile toxicities, Toxicology, 2019, 418, 62–69 CrossRef CAS.
- M. D. Nelms, M. T. D. Cronin, T. W. Schultz and S. J. Enoch, Experimental verification, and domain definition, of structural alerts for protein binding: epoxides, lactones, nitroso, nitros, aldehydes and ketones, SAR QSAR Environ. Res., 2013, 24, 695–709 CrossRef CAS.
- J. A. H. Schwöbel, Y. K. Koleva, S. J. Enoch, F. Bajot, M. Hewitt, J. C. Madden, D. W. Roberts, T. W. Schultz and M. T. D. Cronin, Measurement and estimation of electrophilic reactivity for predictive toxicology, Chem. Rev., 2011, 111, 2562–2596 CrossRef.
- R. G. Pearson, Hard and Soft Acids and Bases, J. Am. Chem. Soc., 1963, 85, 3533–3539 CrossRef CAS.
- R. M. LoPachin, T. Gavin, A. DeCaprio and D. S. Barber, Application of the Hard and Soft, Acids and Bases (HSAB) theory to toxicant – target interactions, Chem. Res. Toxicol., 2012, 25, 239–251 Search PubMed.
- A. Srivastava, J. L. Maggs, D. J. Antoine, D. P. Williams, D. A. Smith and B. K. Park, Role of reactive metabolites in drug-induced hepatotoxicity, Handb. Exp. Pharmacol., 2010, 196, 165–194 Search PubMed.
- P. A. Jackson, J. C. Widen, D. A. Harki and K. M. Brummond, Covalent modifiers: a chemical perspective on the reactivity of α,β-unsaturated carbonyls with thiols via hetero-Michael addition reactions, J. Med. Chem., 2017, 60, 839–885 CrossRef CAS.
- F. Melnikov, B. C. Geohagen, T. Gavin, R. M. LoPachin, P. T. Anastas, P. Coish and D. W. Herr, Application of the hard and soft, acids and bases (HSAB) theory as a method to predict cumulative neurotoxicity, Neurotoxicology, 2020, 79, 95–103 CrossRef CAS.
- A. O. Aptula and D. W. Roberts, Mechanistic applicability domains for nonanimal-based prediction of toxicological end points: General principles and application to reactive toxicity, Chem. Res. Toxicol., 2006, 19, 1097–1105 Search PubMed.
- S. J. Enoch, D. W. Roberts and M. T. D. Cronin, Electrophilic reaction chemistry of low molecular weight respiratory sensitizers, Chem. Res. Toxicol., 2009, 22, 1447–1453 Search PubMed.
- D. W. Roberts, G. Patlewicz, S. D. Dimitrov, L. K. Low, A. O. Aptula, P. S. Kern, G. D. Dimitrova, M. I. H. Comber, R. D. Phillips, J. Niemelä, C. Madsen, E. B. Wedebye, P. T. Bailey and O. G. Mekenyan, TIMES-SS – a mechanistic evaluation of an external validation study using reaction chemistry principles, Chem. Res. Toxicol., 2007, 20, 1321–1330 Search PubMed.
- D. W. Roberts, G. Patlewicz, P. S. Kern, F. Gerberick, I. Kimber, R. J. Dearman, C. A. Ryan, D. A. Basketter and A. O. Aptula, Mechanistic applicability domain classification of a local lymph node assay dataset for skin sensitization, Chem. Res. Toxicol., 2007, 20, 1019–1030 Search PubMed.
- D. A. Dawson, J. L. Allen, T. W. Schultz and G. Pöch, Time-dependence in mixture toxicity with soft-electrophiles: 2. Effects of relative reactivity level on time-dependent toxicity and combined effects for selected Michael acceptors, J. Environ. Sci. Health, Part A: Toxic/Hazard. Subst. Environ. Eng., 2008, 43, 43–52 Search PubMed.
- A. O. Aptula, G. Patlewicz and D. W. Roberts, Skin sensitization: Reaction mechanistic applicability domains for structure-activity relationships, Chem. Res. Toxicol., 2005, 18, 1420–1426 Search PubMed.
- D. Urbisch, M. Becker, N. Honarvar, S. N. Kolle, A. Mehling, W. Teubner, B. Wareing and R. Landsiedel, Assessment of pre- and pro-haptens using nonanimal test methods for skin sensitization, Chem. Res. Toxicol., 2016, 29, 901–913 Search PubMed.
- D. Urbisch, A. Mehling, K. Guth, T. Ramirez, N. Honarvar, S. Kolle, R. Landsiedel, J. Jaworska, P. S. Kern, F. Gerberick, A. Natsch, R. Emter, T. Ashikaga, M. Miyazawa and H. Sakaguchi, Assessing skin sensitization hazard in mice and men using non-animal test methods, Regul. Toxicol. Pharmacol., 2015, 71, 337–351 CrossRef CAS.
- A. O. Aptula, D. W. Roberts and C. K. Pease, Haptens, prohaptens and prehaptens, or electrophiles and proelectrophiles, Contact Dermatitis, 2007, 56, 54–56 CrossRef.
- R. L. Levine, D. Garland, C. N. Oliver, A. Amici, I. Climent, A.-G. Lenz, B.-W. Ahn, S. Shaltiel and E. R. Stadtman, Determination of carbonyl content in oxidatively modified proteins, Methods Enzymol., 1990, 186, 464–478 CAS.
- D. W. Roberts, Linear Free Energy Relationships for reactions of electrophilic halo- and pseudohalobenzenes, and their application in prediction of skin sensitization potential for SNAR electrophiles, Chem. Res. Toxicol., 1995, 8, 545–551 Search PubMed.
- J. F. Bunnett, Mechanism and Reactivity in Aromatic Nucleophilic Substitution Reactions, Quaterly Rev. Chem. Soc., 1958, 12, 1–16 Search PubMed.
- D. W. Roberts, T. W. Schultz, E. M. Wolf and A. O. Aptula, Experimental reactivity parameters for toxicity modeling: Application to the acute aquatic toxicity of SN2 electrophiles to Tetrahymena pyriformis, Chem. Res. Toxicol., 2010, 23, 228–234 Search PubMed.
- J. A. Pals, E. D. Wagner and M. J. Plewa, Energy of the lowest unoccupied molecular orbital, thiol reactivity, and toxicity of three monobrominated water disinfection byproducts, Environ. Sci. Technol., 2016, 50, 3215–3221 CrossRef CAS.
- D. A. Dawson, J. Jeyaratnam, T. Mooneyham, G. Pöch and T. W. Schultz, Mixture toxicity of SN2-reactive soft electrophiles: 1. Evaluation of mixtures containing α-halogenated acetonitriles, Arch. Environ. Contam. Toxicol., 2010, 59, 532–541 CrossRef CAS.
- D. A. Dawson, T. Mooneyham, J. Jeyaratnam, T. W. Schultz and G. Pöch, Mixture toxicity of SN2-reactive soft electrophiles: 2-evaluation of mixtures containing ethyl α-halogenated acetates, Arch. Environ. Contam. Toxicol., 2011, 61, 547–557 CrossRef CAS.
- J. Hemming, B. Holmbom, M. Reunanen and L. Kronberg, Determination of the strong mutagen 3-chloro-4-(dichloromethyl)-5-hydroxy-2(5H)-furanone in chlorinated drinking and humic waters, Chemosphere, 1986, 15, 549–556 CrossRef.
- J. R. Meier, R. B. Knohl, W. E. Coleman, H. P. Rinhand, J. W. Munch, W. H. Kaylor, R. P. Streicher and F. C. Kopfler, Studies on the potent bacterial mutagen 3-chloro-4-dichloromethyl-5-hydroxy-2(5H)furanone: aqueous stability, XAD recovery and analytical determination in drinking water and in chlorinated humic acid solutions, Mutat. Res., 1987, 189, 363–373 CAS.
- G. D. Onstad, H. S. Weinberg and S. W. Krasner, Occurrence of halogenated furanones in U.S. drinking waters, Environ. Sci. Technol., 2008, 42, 3341–3348 CrossRef CAS.
- P. Backlund, L. Kronberg and L. Tikkanen, Formation of Ames mutagenicity and of the strong bacterial mutagen 3-chloro-4-(dichloromethyl)-5-hydroxy-2(5H)-furanone and other halogenated compounds during disinfection of humic water, Chemosphere, 1988, 17, 1329–1336 CrossRef CAS.
- N. M. Peleato, M. McKie, L. Taylor-Edmonds, S. A. Andrews, R. L. Legge and R. C. Andrews, Fluorescence spectroscopy for monitoring reduction of natural organic matter and halogenated furanone precursors by biofiltration, Chemosphere, 2016, 153, 155–161 CrossRef CAS.
- T. Bond, O. Henriet, E. H. Goslan, S. A. Parsons and B. Jefferson, Disinfection byproduct formation and fractionation behavior of natural organic matter surrogates, Environ. Sci. Technol., 2009, 43, 5982–5989 CrossRef CAS.
- T. Bond, E. H. Goslan, S. A. Parsons and B. Jefferson, A critical review of trihalomethane and haloacetic acid formation from natural organic matter surrogates, Environ. Technol. Rev., 2012, 1, 93–113 CrossRef CAS.
- C. M. M. Bougeard, E. H. Goslan, B. Jefferson and S. A. Parsons, Comparison of the disinfection by-product formation potential of treated waters exposed to chlorine and monochloramine, Water Res., 2010, 44, 729–740 CrossRef CAS.
- G. Hua and D. A. Reckhow, Characterization of disinfection byproduct precursors based on hydrophobicity and molecular size, Environ. Sci. Technol., 2007, 41, 3309–3315 CrossRef CAS.
- T. Bond, J. Huang, M. R. Templeton and N. Graham, Occurrence and control of nitrogenous disinfection by-products in drinking water - A review, Water Res., 2011, 45, 4341–4354 CrossRef CAS.
- T. Bond, N. H. Mokhtar Kamal, T. Bonnisseau and M. R. Templeton, Disinfection by-product formation from the chlorination and chloramination of amines, J. Hazard. Mater., 2014, 278, 288–296 CrossRef CAS.
- W. Wang, Y. Qian, L. K. Jmaiff, S. W. Krasner, S. E. Hrudey and X. F. Li, Precursors of halobenzoquinones and their removal during drinking water treatment processes, Environ. Sci. Technol., 2015, 49, 9898–9904 CrossRef CAS.
- Y. Zhao, F. Qin, J. M. Boyd, J. Anichina and X. F. Li, Characterization and determination of chloro- and bromo-benzoquinones as new chlorination disinfection byproducts in drinking water, Anal. Chem., 2010, 82, 4599–4605 CrossRef CAS.
- M. Bedner and W. A. MacCrehan, Transformation of acetaminophen by chlorination produces the toxicants 1,4-benzoquinone and N-acetyl-p-benzoquinone imine, Environ. Sci. Technol., 2006, 40, 516–522 CrossRef CAS.
- W. A. Mitch and I. M. Schreiber, Degradation of tertiary alkylamines during chlorination/chloramination: Implications for formation of aldehydes, nitriles, halonitroalkanes, and nitrosamines, Environ. Sci. Technol., 2008, 42, 4811–4817 CrossRef CAS.
- Z. T. How, K. L. Linge, F. Busetti and C. A. Joll, Chlorination of amino acids: reaction pathways and reaction rates, Environ. Sci. Technol., 2017, 51, 4870–4876 CrossRef CAS.
- S. H. E. E. Joo and W. A. Mitch, Halonitroalkane formation during chlorination/chloramination of primary amines, Environ. Sci. Technol., 2007, 41, 1288–1296 CrossRef CAS.
- A. Dabrowska, J. Świetlik and J. Nawrocki, Formation of aldehydes upon ClO2 disinfection, Water Res., 2003, 37, 1161–1169 CrossRef CAS.
- C. Prasse, U. Von Gunten and D. L. Sedlak, Chlorination of phenols revisited: unexpected formation of α,β-unsaturated C4-dicarbonyl ring cleavage products, Environ. Sci. Technol., 2020, 54, 826–834 CrossRef CAS.
- S. D. Richardson, A. D. Thruston, T. V. Caughran, P. H. Chen, T. W. Collette, T. L. Floyd, K. M. Schenck, B. W. Lykins, G. R. Sun and G. Majetich, Identification of new ozone disinfection byproducts in drinking water, Environ. Sci. Technol., 1999, 33, 3368–3377 CrossRef CAS.
- J. Nawrocki, J. Świetlik, U. Raczyk-Stanisławiak, A. Dąbrowska, S. Biłozor and W. Ilecki, Influence of ozonation conditions on aldehyde and carboxylic acid formation, Ozone: Sci. Eng., 2003, 25, 53–62 CrossRef CAS.
- H. S. Weinberg, W. H. Glaze, S. W. Krasner and M. J. Sclimenti, Formation and removal of aldehydes in plants that use ozonation, J. - Am. Water Works Assoc., 1993, 85, 72–85 CrossRef CAS.
- C. Prasse, M. Wagner, R. Schulz and T. A. Ternes, Oxidation of the antiviral drug acyclovir and its biodegradation product carboxy-acyclovir with ozone: Kinetics and identification of oxidation products, Environ. Sci. Technol., 2012, 46, 2169–2178 CrossRef CAS.
- L. Hureiki, J. P. Croué, B. Legube and M. Doré, Ozonation of amino acids: Ozone demand and aldehyde formation, Ozone: Sci. Eng., 1998, 20, 381–402 CrossRef CAS.
- A. Matilainen and M. Sillanpää, Removal of natural organic matter from drinking water by advanced oxidation processes, Chemosphere, 2010, 80, 351–365 CrossRef CAS.
- M. Pimentel, N. Oturan, M. Dezotti and M. A. Oturan, Phenol degradation by advanced electrochemical oxidation process electro-Fenton using a carbon felt cathode, Appl. Catal., B, 2008, 83, 140–149 CrossRef CAS.
- P. R. Tentscher, M. Bourgin and U. von Gunten, Ozonation of para-substituted phenolic compounds yields p-benzoquinones, other cyclic α,β-unsaturated ketones, and substituted catechols, Environ. Sci. Technol., 2018, 52, 4763–4773 CrossRef CAS.
- P. Mazellier, C. Busset, A. Delmont and J. De Laat, A comparison of fenuron degradation by hydroxyl and carbonate radicals in aqueous solution, Water Res., 2007, 41, 4585–4594 CrossRef CAS.
- L. A. Pérez-Estrada, S. Malato, W. Gernjak, A. Agüera, E. M. Thurman, I. Ferrer and A. R. Fernández-Alba, Photo-fenton degradation of diclofenac: Identification of main intermediates and degradation pathway, Environ. Sci. Technol., 2005, 39, 8300–8306 CrossRef.
- M. M. Sein, M. Zedda, J. Tuerk, T. C. Schmidt, A. Golloch and C. Von Sonntag, Oxidation of diclofenac with ozone in aqueous solution, Environ. Sci. Technol., 2008, 42, 6656–6662 CrossRef CAS.
- S. Gäb, W. V. Turner, S. Wolff, K. H. Becker, L. Ruppert and K. J. Brockmann, Formation of alkyl and hydroxyalkyl hydroperoxides on ozonolysis in water and in air, Atmos. Environ., 1995, 29, 2401–2407 CrossRef.
- W. A. Pryor, B. Das and D. F. Church, The ozonation of unsaturated fatty acids: aldehydes and hydrogen peroxide as products and possible mediators of ozone toxicity, Chem. Res. Toxicol., 1991, 4, 341–348 Search PubMed.
- R. Flyunt, J. A. Theruvathu, A. Leitzke and C. Von Sonntag, The reactions of thymine and thymidine with ozone, J. Chem. Soc., Perkin Trans. 2, 2002, 2, 1572–1582 RSC.
- C. Prasse, B. Ford, D. K. Nomura and D. L. Sedlak, Unexpected transformation of dissolved phenols to toxic dicarbonyls by hydroxyl radicals and UV light, Proc. Natl. Acad. Sci. U. S. A., 2018, 115, 2311–2316 CrossRef CAS.
- J. Van Buren, C. Prasse, E. L. Marron, B. Skeel and D. L. Sedlak, Ring-cleavage products produced during the initial phase of oxidative treatment of alkyl-substituted aromatic compounds, Environ. Sci. Technol., 2020, 54, 8352–8361 CrossRef CAS.
-
C. Von Sonntag and U. Von Gunten, Chemistry of Ozone in Water and Wastewater Treatment, IWA Publishing, 2012 Search PubMed.
- D. S. Schechter and P. C. Singer, Formation of aldehydes during ozonation, Ozone: Sci. Eng., 1995, 17, 53–69 CrossRef CAS.
- S. Liu, M. Lim, R. Fabris, C. Chow, M. Drikas and R. Amal, TiO2 photocatalysis of natural organic matter in surface water: Impact on trihalomethane and haloacetic acid formation potential, Environ. Sci. Technol., 2008, 42, 6218–6223 CrossRef CAS.
- J. Agbaba, J. M. Jazić, A. Tubić, M. Watson, S. Maletić, M. K. Isakovski and B. Dalmacija, Oxidation of natural organic matter with processes involving O3, H2O2 and UV light: Formation of oxidation and disinfection by-products, RSC Adv., 2016, 6, 86212–86219 RSC.
- R. Medina-Navarro, E. Mercado-Pichardo, O. Hernández-Perez and J. J. Hicks, Identification of acrolein from the ozone oxidation of unsaturated fatty acids, Hum. Exp. Toxicol., 1999, 18, 677–682 CrossRef CAS.
- C. H. Jeong, C. Postigo, S. D. Richardson, J. E. Simmons, S. Y. Kimura, B. J. Mariñas, D. Barcelo, P. Liang, E. D. Wagner and M. J. Plewa, Occurrence and comparative toxicity of haloacetaldehyde disinfection byproducts in drinking water, Environ. Sci. Technol., 2015, 49, 13749–13759 CrossRef CAS.
- K. P. Kringstad, P. O. Ljungquist, F. de Sousa and L. M. Strmberg, On the formation of mutagens in the chlorination of humic acid, Environ. Sci. Technol., 1983, 17, 553–555 CrossRef CAS.
- Y. H. Chuang, D. L. McCurry, H. H. Tung and W. A. Mitch, Formation pathways and trade-offs between haloacetamides and haloacetaldehydes during combined chlorination and chloramination of lignin phenols and natural waters, Environ. Sci. Technol., 2015, 49, 14432–14440 CrossRef CAS.
- Y. qin Mao, X. mao Wang, X. fen Guo, H. wei Yang and Y. F. Xie, Characterization of haloacetaldehyde and trihalomethane formation potentials during drinking water treatment, Chemosphere, 2016, 159, 378–384 CrossRef.
- L. Kronberg and R. Franzén, Determination of chlorinated furanones, hydroxyfuranones, and butenedioic acids in chlorine-treated water and in pulp bleaching liquor, Environ. Sci. Technol., 1993, 27, 1811–1818 CrossRef CAS.
- P. Backlund, E. Wondergem, K. Voogd and A. De Jong, Mutagenic activity and presence of the strong mutagen 3-chloro-4-(dichloromethyl-5-hydroxy-2(5H)-furanone
(MX) in chlorinated raw and drinking waters in the Netherlands, Sci. Total Environ., 1989, 84, 273–282 CrossRef CAS.
- B. Holmbom, R. H. Voss, R. D. Mortimer and A. Wong, Fractionation, isolation, and characterization of Ames mutagenic compounds in Kraft chlorination effluents, Environ. Sci. Technol., 1984, 18, 333–337 CrossRef CAS.
- M. Nihemaiti, J. Le Roux, C. Hoppe-Jones, D. A. Reckhow and J. P. Croué, Formation of haloacetonitriles, haloacetamides, and nitrogenous heterocyclic byproducts by chloramination of phenolic compounds, Environ. Sci. Technol., 2017, 51, 655–663 CrossRef CAS.
- K. L. Linge, I. Kristiana, D. Liew, A. Holman and C. A. Joll, Halogenated semivolatile acetonitriles as chloramination disinfection by-products in water treatment: A new formation pathway from activated aromatic compounds, Environ. Sci.: Processes Impacts, 2020, 22, 653–662 RSC.
- W. Lee, P. Westerhoff and J. P. Croué, Dissolved organic nitrogen as a precursor for chloroform, dichloroacetonitrile, N-nitrosodimethylamine, and trichloronitromethane, Environ. Sci. Technol., 2007, 41, 5485–5490 CrossRef CAS.
- A. D. Nikolaou and T. D. Lekkas, The role of natural organic matter during formation of chlorination by-products: a review, Acta Hydrochim. Hydrobiol., 2001, 29, 63–77 CrossRef CAS.
- S. Y. Kimura, Y. Komaki, M. J. Plewa and B. J. Mariñas, Chloroacetonitrile and N,2-dichloroacetamide formation from the reaction of chloroacetaldehyde and monochloramine in water, Environ. Sci. Technol., 2013, 47, 12382–12390 CrossRef CAS.
- H. Huang, Q. Y. Wu, H. Y. Hu and W. A. Mitch, Dichloroacetonitrile and dichloroacetamide can form independently during chlorination and chloramination of drinking waters, model organic matters, and wastewater effluents, Environ. Sci. Technol., 2012, 46, 10624–10631 CrossRef CAS.
- K. L. Simpson and K. P. Hayes, Drinking water disinfection by-products: an Australian perspective, Water Res., 1998, 32, 1522–1528 CrossRef CAS.
- V. Kanokkantapong, T. F. Marhaba, P. Pavasant and B. Panyapinyophol, Characterization of haloacetic acid precursors in source water, J. Environ. Manage., 2006, 80, 214–221 CrossRef CAS.
- W. Chu, X. Li, T. Bond, N. Gao and D. Yin, The formation of haloacetamides and other disinfection by-products from non-nitrogenous low-molecular weight organic acids during chloramination, Chem. Eng. J., 2016, 285, 164–171 CrossRef CAS.
- H. Liu, T. A. Bruton, W. Li, J. Van Buren, C. Prasse, F. M. Doyle and D. L. Sedlak, Oxidation of benzene by persulfate in the presence of Fe(III)- and Mn(IV)-containing oxides: Stoichiometric efficiency and transformation products, Environ. Sci. Technol., 2016, 50, 890–898 CrossRef CAS.
- K. Kosaka, T. Nakai, Y. Hishida, M. Asami, K. Ohkubo and M. Akiba, Formation of 2,6-dichloro-1,4-benzoquinone from aromatic compounds after chlorination, Water Res., 2017, 110, 48–55 CrossRef CAS.
- N. Boonrattanakij, M. C. Lu and J. Anotai, Kinetics and mechanism of 2,6-dimethyl-aniline degradation by hydroxyl radicals, J. Hazard. Mater., 2009, 172, 952–957 CrossRef CAS.
- R. G. Rice and M. Gomez-Taylor, Occurrence of by-products of strong oxidants reacting with drinking water contaminants - Scope of the problem, Environ. Health Perspect., 1986, 69, 31–44 CAS.
- P. S. Bailey, The reactions of ozone with organic compounds, Chem. Rev., 1958, 58, 925–1010 CrossRef CAS.
- P. S. Bailey, H. H. Hwang and C. Y. Chiang, Mechanisms of epoxidation during ozonation of carbon-carbon double bonds, J. Org. Chem., 1985, 50, 231–234 CrossRef CAS.
- P. Dowideit and C. Von Sonntag, Reaction of ozone with ethene and its methyl- and chlorine-substituted derivatives in aqueous solution, Environ. Sci. Technol., 1998, 32, 1112–1119 CrossRef CAS.
- S. J. Khan, H. S. Weinberg and E. C. Bedford, Aqueous-phase aminolysis: approach for the analysis of epoxides in water, Anal. Chem., 2006, 78, 2608–2616 CrossRef CAS.
- M. Soufan, M. Deborde, A. Delmont and B. Legube, Aqueous chlorination of carbamazepine: kinetic study and transformation product identification, Water Res., 2013, 47, 5076–5087 CrossRef CAS.
- M. Stylidi, D. I. Kondarides and X. E. Verykios, Pathways of solar light-induced photocatalytic degradation of azo dyes in aqueous TiO2 suspensions, Appl. Catal., B, 2003, 40, 271–286 CrossRef CAS.
- X. Wang, C. Chen, J. Li and X. Wang, Ozone degradation of 1-naphthol on multiwalled carbon nanotubes/iron oxides and recycling of the adsorbent, Chem. Eng. J., 2015, 262, 1303–1310 CrossRef CAS.
- S. Hammami, N. Bellakhal, N. Oturan, M. A. Oturan and M. Dachraoui, Degradation of Acid Orange 7 by electrochemically generated •OH radicals in acidic aqueous medium using a boron-doped diamond or platinum anode: a mechanistic study, Chemosphere, 2008, 73, 678–684 CrossRef CAS.
- N. Zhu, L. Gu, H. Yuan, Z. Lou, L. Wang and X. Zhang, Degradation pathway of the naphthalene azo dye intermediate 1-diazo-2-naphthol-4-sulfonic acid using Fenton’s reagent, Water Res., 2012, 46, 3859–3867 CrossRef CAS.
- J. Theurich, D. W. Bahnemann, R. Vogel, F. E. Ehamed, G. Alhakimi and I. Rajab, Photocatalytic degradation of naphthalene and anthracene: GC-MS analysis of the degradation pathway, Res. Chem. Intermed., 1997, 23, 247–274 CrossRef CAS.
- S. E. Duirk and T. W. Collette, Degradation of chlorpyrifos in aqueous chlorine solutions: Pathways, kinetics, and modelling, Environ. Sci. Technol., 2006, 40, 546–551 CrossRef CAS.
- J. Wu, C. Lan and G. Y. S. Chan, Organophosphorus pesticide ozonation and formation of oxon intermediates, Chemosphere, 2009, 76, 1308–1314 CrossRef CAS.
- M. Bavcon Kralj, M. Franko and P. Trebše, Photodegradation of organophosphorus insecticides – investigations of products and their toxicity using gas chromatography-mass spectrometry and AChE-thermal lens spectrometric bioassay, Chemosphere, 2007, 67, 99–107 CrossRef CAS.
- M. Bavcon, P. Trebše and L. Zupančič-Kralj, Investigations of the determination and transformations of diazinon and malathion under environmental conditions using gas chromatography coupled with a flame ionisation detector, Chemosphere, 2003, 50, 595–601 CrossRef CAS.
- E. J. Pedersen, E. T. Urbansky, B. J. Mariñas and D. W. Margerum, Formation of cyanogen chloride from the reaction of monochloramine with formaldehyde, Environ. Sci. Technol., 1999, 33, 4239–4249 CrossRef CAS.
- Y. Hirose, N. Maeda, T. Ohya, K. Nojima and S. Kanno, Formation of cyanogen chloride by the reaction of amino acids with hypochlorous acid in the persence of ammonium ion, Chemosphere, 1988, 17, 865–873 CrossRef CAS.
- Y. Xie and D. A. Reckhow, A rapid and simple analytical method for cyanogen chloride and cyanogen bromide in drinking water, Water Res., 1993, 27, 507–511 CrossRef CAS.
- L. A. Allen, N. Blezard and A. B. Wheatland, Formation of cyanogen chloride during chlorination of certain liquids toxicity of such liquids to fish, J. Hyg., 1948, 46, 184–193 CrossRef CAS.
- A. Leitzke, R. Flyunt, J. A. Theruvathu and C. Von Sonntag, Ozonolysis of vinyl compounds, CH2=CH-X, in aqueous solution - The chemistries of the ensuing formyl compounds and hydroperoxides, Org. Biomol. Chem., 2003, 1, 1012–1019 RSC.
- T. W. Schultz, R. E. Carlson, M. T. D. Cronin, J. L. M. Hermens, R. Johnson, P. J. O'Brien, D. W. Roberts, A. Siraki, K. B. Wallace and G. D. Veith, A conceptual framework for predicting the toxicity of reactive chemicals: Modeling soft electrophilicity, SAR QSAR Environ. Res., 2006, 17, 413–428 CrossRef CAS.
- A. S. Kalgutkar, Liabilities associated with the formation of “hard” electrophiles in reactive metabolite trapping screens, Chem. Res. Toxicol., 2017, 30, 220–238 Search PubMed.
- A. F. Stepan, D. P. Walker, J. Bauman, D. A. Price, T. A. Baillie, A. S. Kalgutkar and M. D. Aleo, Structural alert/reactive metabolite concept as applied in medicinal chemistry to mitigate the risk of idiosyncratic drug toxicity: a perspective based on the critical examination of trends in the top 200 drugs marketed in the United States, Chem. Res. Toxicol., 2011, 24, 1345–1410 Search PubMed.
- J. Y. M. Tang, E. Glenn, H. Thoen and B. I. Escher, In vitro bioassay for reactive toxicity towards proteins implemented for water quality monitoring, J. Environ. Monit., 2012, 14, 1073–1081 RSC.
- Y. Chen, M. Monshouwer and W. L. Fitch, Analytical tools and approaches for metabolite identification in early drug discovery, Pharm. Res., 2007, 24, 248–257 CrossRef CAS.
- O. Pelkonen, M. Pasanen, A. Tolonen, M. Koskinen, J. Hakkola, K. Abass, J. Laine, M. Hakkinen, R. Juvonen, S. Auriola, M. Storvik, P. Huuskonen, T. Rousu and M. Rahikkala, Reactive metabolites in early drug development: predictive in vitro tools, Curr. Med. Chem., 2015, 22, 538–550 CrossRef CAS.
- J. E. Laine, M. R. Häkkinen, S. Auriola, R. O. Juvonen and M. Pasanen, Comparison of trapping profiles between d-peptides and glutathione in the identification of reactive metabolites, Toxicol. Rep., 2015, 2, 1024–1032 CrossRef CAS.
- J. E. Laine, S. Auriola, M. Pasanen and R. O. Juvonen, D-Isomer of gly-tyr-pro-cys-pro-his-pro peptide: A novel and sensitive in vitro trapping agent to detect reactive metabolites by electrospray mass spectrometry, Toxicol. In Vitro, 2011, 25, 411–425 CrossRef CAS.
- C. Avonto, A. G. Chittiboyina, M. Wang, Y. Vasquez, D. Rua and I. A. Khan, In chemico evaluation of tea tree essential oils as skin sensitizers: impact of the chemical composition on aging and generation of reactive species, Chem. Res. Toxicol., 2016, 29, 1108–1117 Search PubMed.
- G. F. Gerberick, The use of peptide reactivity assays for skin sensitisation hazard identification and risk assessment, ATLA, Altern. Lab. Anim., 2016, 44, 437–442 Search PubMed.
- G. F. Gerberick, J. A. Troutman, L. M. Foertsch, J. D. Vassallo, M. Quijano, R. L. M. Dobson, C. Goebel and J. P. Lepoittevin, Investigation of peptide reactivity of pro-hapten skin sensitizers using a peroxidase-peroxide oxidation system, Toxicol. Sci., 2009, 112, 164–174 CrossRef CAS.
- K. M. Sullivan, S. J. Enoch, J. Ezendam, K. Sewald, E. L. Roggen and S. Cochrane, An adverse outcome pathway for sensitization of the respiratory tract by low-molecular-weight chemicals: Building evidence to support the utility of in vitro and in silico methods in a regulatory context, Appl. In Vitro Toxicol., 2017, 3, 213–226 CrossRef CAS.
- A. Chary, J. Hennen, S. G. Klein, T. Serchi, A. C. Gutleb and B. Blömeke, Respiratory sensitization: toxicological point of view on the available assays, Arch. Toxicol., 2018, 92, 803–822 CrossRef CAS.
- J. Arts, How to assess respiratory sensitization of low molecular weight chemicals?, Int. J. Hyg. Environ. Health, 2020, 225, 113469 CrossRef CAS.
- J. F. Lalko, I. Kimber, G. Frank Gerberick, L. M. Foertsch, A. M. Api and R. J. Dearman, The direct peptide reactivity assay: selectivity of chemical respiratory allergens, Toxicol. Sci., 2012, 129, 421–431 CrossRef CAS.
- L. Ndreu, L. N. Erber, M. Törnqvist, N. Y. Tretyakova and I. Karlsson, Characterizing adduct formation of electrophilic skin allergens with human serum albumin and hemoglobin, Chem. Res. Toxicol., 2020, 33, 2623–2636 Search PubMed.
- H. Carlsson, S. M. Rappaport and M. Törnqvist, Protein adductomics: methodologies for untargeted screening of adducts to serum albumin and hemoglobin in human blood samples, High-Throughput, 2019, 8, 6 Search PubMed.
- R. J. Preston, DNA reactivity as a mode of action and its relevance to cancer risk assessment, Toxicol. Pathol., 2013, 41, 322–325 CrossRef CAS.
- R. Benigni and C. Bossa, Structural alerts of mutagens and carcinogens, Curr. Comput.-Aided Drug Des., 2006, 2, 169–176 CrossRef CAS.
- A. B. Bailey, R. Chanderbhan, N. Collazo-Braier, M. A. Cheeseman and M. L. Twaroski, The use of structure-activity relationship analysis in the food contact notification program, Regul. Toxicol. Pharmacol., 2005, 42, 225–235 CrossRef CAS.
- C. L. Wong, S. Ghassabian, M. T. Smith and A. L. Lam, In vitro methods for hazard assessment of industrial chemicals - Opportunities and challenges, Front. Pharmacol., 2015, 6, 1–13 CAS.
-
J. Cahill, J. D. L. Williams, M. C. Matheson, A. M. Palmer, J. A. Burgess, S. C. Dharmage and R. L. Nixon, Occupational Contact Dermatitis: A Review of 18 Years of Data from an Occupational Dermatology Clinic in Australia, Occupational Dermatology Research and Education Centre, Skin and Cancer Foundation Inc., Victoria, Australia, 2012 Search PubMed.
- G. F. Gerberick, J. D. Vassallo, R. E. Bailey, J. G. Chaney, S. W. Morrall and J. P. Lepoittevin, Development of a peptide reactivity assay for screening contact allergens, Toxicol. Sci., 2004, 81, 332–343 CrossRef CAS.
-
OECD, Test No. 442C: in Chemico Skin Sensitisation: Assays Addressing the Adverse Outcome Pathway Key Event on Covalent Binding to Proteins, OECD Guidelines for the Testing of Chemicals, Section 4, OECD Publishing Paris, 2020 Search PubMed.
- D. W. Roberts and A. Natsch, High throughput kinetic profiling approach for covalent binding to peptides: Application to skin sensitization potency of Michael acceptor electrophiles, Chem. Res. Toxicol., 2009, 22, 592–603 Search PubMed.
- I. Chipinda, R. O. Ajibola, M. K. Morakinyo, T. B. Ruwona, R. H. Simoyi and P. D. Siegel, Rapid and simple kinetics screening assay for electrophilic dermal sensitizers using nitrobenzenethiol, Chem. Res. Toxicol., 2010, 23, 918–925 Search PubMed.
- I. Chipinda, W. Mbiya, R. A. Adigun, M. K. Morakinyo, B. F. Law, R. H. Simoyi and P. D. Siegel, Pyridoxylamine reactivity kinetics as an amine based nucleophile for screening electrophilic dermal sensitizers, Toxicology, 2014, 315, 102–109 CrossRef CAS.
- M. Fujita, Y. Yamamoto, H. Tahara, T. Kasahara, Y. Jimbo and T. Hioki, Development of a prediction method for skin sensitization using novel cysteine and lysine derivatives, J. Pharmacol. Toxicol. Methods, 2014, 70, 94–105 CrossRef CAS.
- M. Fujita, Y. Yamamoto, S. Watanabe, T. Sugawara, K. Wakabayashi, Y. Tahara, N. Horie, K. Fujimoto, K. Kusakari, Y. Kurokawa, T. Kawakami, K. Kojima, H. Kojima, A. Ono, Y. Katsuoka, H. Tanabe, H. Yokoyama and T. Kasahara, Cause of and countermeasures for oxidation of the cysteine-derived reagent used in the amino acid derivative reactivity assay, J. Appl. Toxicol., 2019, 39, 191–208 CrossRef CAS.
- S. Wanibuchi, Y. Yamamoto, A. Sato, T. Kasahara and M. Fujita, The amino acid derivative reactivity assay with fluorescence detection and its application to multi-constituent substances, J. Toxicol. Sci., 2019, 44, 821–832 CrossRef CAS.
- C. Avonto, A. G. Chittiboyina, D. Rua and I. A. Khan, A fluorescence high throughput screening method for the detection of reactive electrophiles as potential skin sensitizers, Toxicol. Appl. Pharmacol., 2015, 289, 177–184 CrossRef CAS.
- A. G. Chittiboyina, C. Avonto, D. Rua and I. A. Khan, Alternative testing methods for skin sensitization: NMR spectroscopy for probing the reactivity and classification of potential skin sensitizers, Chem. Res. Toxicol., 2015, 28, 1704–1714 Search PubMed.
- A. Natsch and H. Gfeller, LC-MS-based characterization of the peptide reactivity of chemicals to improve the in vitro prediction of the skin sensitization potential, Toxicol. Sci., 2008, 106, 464–478 CrossRef CAS.
- S. R. Ahlfors, O. Sterner and C. Hansson, Reactivity of contact allergenic haptens to amino acid residues in a model carrier peptide, and characterization of formed peptide-hapten adducts, Skin Pharmacol. Appl. Skin Physiol., 2003, 16, 59–68 CrossRef CAS.
- Y. Yamamoto, H. Tahara, R. Usami, T. Kasahara, Y. Jimbo, T. Hioki and M. Fujita, A novel in chemico method to detect skin sensitizers in highly diluted reaction conditions, J. Appl. Toxicol., 2015, 35, 1348–1360 CrossRef CAS.
- A. Natsch, R. Emter, H. Gfeller, T. Haupt and G. Ellis, Predicting skin sensitizer potency based on in vitro data from keratinosens and kinetic peptide binding: Global versus domain-based assessment, Toxicol. Sci., 2015, 143, 319–332 CrossRef CAS.
- C. L. Wong, A. L. Lam, M. T. Smith and S. Ghassabian, Evaluation of a high-throughput peptide reactivity format assay for assessment of the skin sensitization potential of chemicals, Front. Pharmacol., 2016, 7, 53 CrossRef.
- M. K. Dennehy, K. A. M. Richards, G. R. Wernke, Y. Shyr and D. C. Liebler, Cytosolic and nuclear protein targets of thiol-reactive electrophiles, Chem. Res. Toxicol., 2006, 19, 20–29 Search PubMed.
- J. A. Troutman, L. M. Foertsch, P. S. Kern, H. J. Dai, M. Quijano, R. L. M. Dobson, J. F. Lalko, J. P. Lepoittevin and G. F. Gerberick, The incorporation of lysine into the peroxidase peptide reactivity assay for skin sensitization assessments, Toxicol. Sci., 2011, 122, 422–436 CrossRef CAS.
- J. F. Lalko, R. J. Dearman, G. F. Gerberick, J. A. Troutman, A. M. Api and I. Kimber, Reactivity of chemical respiratory allergens in the Peroxidase Peptide Reactivity Assay, Toxicol. In Vitro, 2013, 27, 651–661 CrossRef CAS.
- M. H. Reihmann and H. Ritter, Regioselective HRP-catalyzed polymerization of 4-amino-phenol, J. Macromol. Sci., Part A: Pure Appl.Chem., 2002, 39, 1369–1382 CrossRef.
- Y. Yamamoto, M. Fujita, S. Wanibuchi, A. Sato, Y. Katsuoka and T. Kasahara, Development of photo-amino acid derivative reactivity assay: a novel in chemico alternative method for predicting photoallergy, J. Appl. Toxicol., 2020, 40, 655–678 CrossRef CAS.
- N. H. Patel, P. K. Mishra, R. Nagane, A. Deshpande, I. Y. Tamboli and R. Date, Comparison of in chemico skin sensitization methods and development of in chemico skin photosensitization assays, ALTEX, 2019, 36, 373–387 Search PubMed.
- C. Stiefel and W. Schwack, Rapid screening method to study the reactivity of UV filter substances towards skin proteins by high-performance thin-layer chromatography, Int. J. Cosmet. Sci., 2013, 35, 588–599 CrossRef CAS.
- C. Stiefel and W. Schwack, Reactivity of cosmetic UV filters towards skin proteins: Model studies with Boc-lysine, Boc-Gly-Phe-Gly-Lys-OH, BSA and gelatin, Int. J. Cosmet. Sci., 2014, 36, 561–570 CrossRef CAS.
- R. I. de Ávila, G. C. Teixeira, D. F. M. C. Veloso, L. C. Moreira, E. M. Lima and M. C. Valadares, In vitro assessment of skin sensitization, photosensitization and phototoxicity potential of commercial glyphosate-containing formulations, Toxicol. In Vitro, 2017, 45, 386–392 CrossRef.
- J. A. Pals, E. D. Wagner, M. J. Plewa, M. Xia and M. S. Attene-Ramos, Monohalogenated acetamide-induced cellular stress and genotoxicity are related to electrophilic softness and thiol/thiolate reactivity, J. Environ. Sci., 2017, 58, 224–230 CrossRef CAS.
- X. Wei, M. Yang, Q. Zhu, E. D. Wagner and M. J. Plewa, Comparative quantitative toxicology and QSAR modeling of the haloacetonitriles: Forcing agents of water disinfection by-product toxicity, Environ. Sci. Technol., 2020, 54, 8909–8918 CrossRef CAS.
- A. Böhme, D. Thaens, A. Paschke and G. Schüürmann, Kinetic glutathione chemoassay to quantify thiol reactivity of organic electrophiless - Application to α,β-unsaturated ketones, acrylates, and propiolates, Chem. Res. Toxicol., 2009, 22, 742–750 Search PubMed.
- S. Dong, M. A. Page, E. D. Wagner and M. J. Plewa, Thiol reactivity analyses to predict mammalian cell cytotoxicity of water samples, Environ. Sci. Technol., 2018, 52, 8822–8829 CrossRef CAS.
- S. Dong, M. A. Page, N. Massalha, A. Hur, K. Hur, K. Bokenkamp, E. D. Wagner and M. J. Plewa, Toxicological comparison of water, wastewaters, and processed wastewaters, Environ. Sci. Technol., 2019, 53, 9139–9147 CrossRef CAS.
- S. Dong, N. Masalha, M. J. Plewa and T. H. Nguyen, Toxicity of wastewater with elevated bromide and iodide after chlorination, chloramination, or ozonation disinfection, Environ. Sci. Technol., 2017, 51, 9297–9304 CrossRef CAS.
- A. M. Cheh and R. E. Carlson, Determination of potentially mutagenic and carcinogenic electrophiles in environmental samples, Anal. Chem., 1981, 53, 1001–1006 CrossRef CAS.
- E. Giménez-Arnau, Chemical compounds responsible for skin allergy to complex mixtures: How to identify them?, Cosmetics, 2019, 6, 71 CrossRef.
- Y. Yamamoto, M. Fujita, S. Wanibuchi, Y. Katsuoka, A. Ono and T. Kasahara, Expanding the applicability of the amino acid derivative reactivity assay: determining a weight for preparation of test chemical solutions that yield a predictive capacity identical to the conventional method using molar concentration and demonstrating the capacity to detect sensitizers in liquid mixtures, J. Pharmacol. Toxicol. Methods, 2019, 97, 67–79 CrossRef CAS.
- A. Freidig, M. Hofhuis, I. Van Holstijn and J. Hermens, Glutathione depletion in rat hepatocytes: a mixture toxicity study with α,β-unsaturated esters, Xenobiotica, 2001, 31, 295–307 CrossRef CAS.
- C. M. Villanueva, M. Kogevinas, S. Cordier, M. R. Templeton, R. Vermeulen, J. R. Nuckols, M. J. Nieuwenhuijsen and P. Levallois, Assessing exposure and health consequences of chemicals in drinking water: current state of knowledge and research needs, Environ. Health Perspect., 2014, 122, 213–221 CrossRef CAS.
- J. D. Bos and M. M. H. M. Meinardi, The 500 Dalton rule for the skin penetration of chemical compounds and drugs, Exp. Dermatol., 2000, 9, 165–169 CrossRef CAS.
- J. M. Fitzpatrick, D. W. Roberts and G. Patlewicz, What determines skin sensitization potency: Myths, maybes and realities. The 500 molecular weight cut-off: An updated analysis, J. Appl. Toxicol., 2017, 37, 105–116 CrossRef CAS.
- H. Hsu-Kim, Stability of metal-glutathione complexes during oxidation by hydrogen peroxide and Cu(II)-catalysis, Environ. Sci. Technol., 2007, 41, 2338–2342 CrossRef CAS.
- M. Moingt, M. Bressac, D. Bélanger and M. Amyot, Role of ultra-violet radiation, mercury and copper on the stability of dissolved glutathione in natural and artificial freshwater and saltwater, Chemosphere, 2010, 80, 1314–1320 CrossRef CAS.
- A. Böhme, D. Thaens, A. Paschke and G. Schüürmann, Kinetic glutathione chemoassay to quantify thiol reactivity of organic electrophiless - application to α,β-unsaturated ketones, acrylates, and propiolates, Chem. Res. Toxicol., 2009, 22, 742–750 Search PubMed.
- A. Böhme, D. Thaens, F. Schramm, A. Paschke and G. Schüürmann, Thiol reactivity and its impact on the ciliate toxicity of α,β-unsaturated aldehydes, ketones, and esters, Chem. Res. Toxicol., 2010, 23, 1905–1912 Search PubMed.
- A. Natsch, T. Haupt, B. Wareing, R. Landsiedel and S. N. Kolle, Predictivity of the kinetic direct peptide reactivity assay (kDPRA) for sensitizer potency assessment and subclassification, ALTEX, 2020, 37, 652–664 Search PubMed.
Footnote |
† Electronic supplementary information (ESI) available. See DOI: 10.1039/d0em00471e |
|
This journal is © The Royal Society of Chemistry 2021 |