Ammonia fluxes and emission factors under an intensively managed wetland rice ecosystem†
Received
26th August 2020
, Accepted 25th November 2020
First published on 10th December 2020
Abstract
Nitrogen (N) loss from rice production systems in the form of ammonia (NH3) can be a significant N loss pathway causing significant economic and environmental costs. Yet, data on NH3 fluxes in wetland rice ecosystems are still very scarce which limits the accuracy of national and global NH3 budgets. We measured the NH3 fluxes in situ in a wetland rice field and estimated emission factors (EF) under two soil management systems (i.e. conventional tillage, CT and strip tillage, ST); two residue retention levels (i.e. 15%, LR and 40% crop residue by height, HR); and three N fertilization rates (i.e. 108, 144 and 180 kg N ha−1) in two consecutive years (2019 and 2020). The highest NH3 peaks were observed within the first 3 days after urea application. The mean and cumulative NH3 fluxes significantly increased with the increases in N fertilization rates and were 18.5% and 18.6% higher in ST than in CT in 2020 but not in 2019. Overall, the highest mean NH3 fluxes were in 180 kg N ha−1 coupled with either HR or LR and ST or CT. In 2019, the NH3 EF was unchanged by any treatments. In 2020, the lower EF was in CT coupled with LR (15%) than all other treatment combinations, where ST with HR showed the highest EF (20%). Likewise, the lowest N rate (108 kg N ha−1) in ST had the highest NH3 EF (20%) that was similar to higher N rates (144 and 180 kg N ha−1) in the same tillage treatment and to 180 kg N ha−1 in CT. Our results highlight that NH3 fluxes in rice field particularly the effects of ST correlated with higher soil pH and NH4+ content and lower redox potential. Our results highlight that NH3 fluxes are a potentially large N loss pathway in wetland rice under conventional and decreased soil disturbance regimes.
Environmental significance
Nitrogen losses from rice field cause huge economic and environmental costs. The IPPC set a default value for N loss as NH3 from soils which has huge uncertainty. The mechanistic understanding of N loss as NH3 in diversified managements in rice ecosystems is required to improve N use efficiency and to reduce reactive N loss to the environment. In situ field measurement of NH3 is very limited due to difficulties in the measurement leading to uncertainty in global N budget. We have measured in situ NH3 volatilization loss, NH3 emissions and emission factors under diverse management practices: different tillage systems, crop residue retention and N fertilizer rates. These data will not only provide new information on our understanding of N loss via volatilization, but also adoptions of better management towards lower N loss. The NH3 emissions factor will add up new information that will help modelling and estimating N loss, N balance and ecosystem level N cycling, particularly in rice ecosystems. The quantified NH3 emission factor will help revising the IPCC emission factor for national inventory. Because the IPCC emission factors has been used in Bangladesh since the beginning of such estimation which was not a quantified value but an assumed value.
|
1. Introduction
With the rapid growth of the human population, the decrease in arable land and the associated economic expansion are expected to cause a considerable increase of nitrogen (N) fertilizer use in rice production systems in Asia. Average N application is about 150 kg N ha−1 season−1 in rice fields in Bangladesh which is about two times higher than the rate in Japan (80 kg N per ha per season) and a little higher than in the USA (140 kg N per ha per season).1,2 Nitrogen use efficiency in wetland rice (Oryza sativa L.) is very low because of high N losses to the environment.3 The worldwide drive for higher yield through the application of greater amounts of N fertilizer to paddy soils may exacerbate gaseous N losses.4,5 Among the gaseous N emissions, ammonia (NH3) fluxes are considered to be an important N loss pathway.6,7 Agricultural gaseous N losses as NH3 are predicted to increase in Asia from 13.8 Tg N per year in 2000 to 18.8 Tg N per year in 2030.8 However, this estimate is associated with large uncertainties, due to the high spatial and temporal variabilities of NH3 fluxes.9 Fan et al. (2006) reported that the gaseous N loss through volatilization during the rice growing season varied between 9% and 40% of the N applied.10 Such a wide range of volatilization loss makes estimates of agricultural NH3 loss uncertain leading to inaccuracies in the calculations of the N balance.
Significant NH3 fluxes not only cause economic loss but can also have negative impacts on agricultural soil by acidification, on aquatic ecosystems by eutrophication and contribute to indirect nitrous oxide (N2O) emissions.11 Similarly, it creates serious problems to human health as ammonia reacts with other air pollutants to create tiny particles that can lodge deep in the lungs, causing asthma attacks, bronchitis, and heart attacks.12 Due to its potential impacts on the sustainability of food production, it is critical that the data on NH3 emissions are accurate and precise, and lead to improved mitigation approaches and management strategies.13 Currently, in the Indo-Gangetic Plain NH3 volatilization is estimated by the IPCC Tier 1 method (using a single default emission factor expressed as a fraction of the N applied to the soil), based on Bouwman (1996).14 The limitations of such an approach are that the same Emission Factor (EF) is used irrespective of the fertilizer sources, soil types, climatic conditions or management strategies. The synthesis of regionally validated EFs would provide a much-needed improvement over the current IPCC Tier 1 approach, leading to an inventory that reflects the region's fertilizer management practices, soils and climate. While the Indo Gangetic Plain was reported as one of the hot spots for NH3 fluxes in the world,6 no reliable data set are available for this region preventing reliable EF calculations.
As one of the tools of sustainable agriculture, minimum soil disturbance (e.g., minimum tillage, MT; strip tillage, ST) or no-tillage (NT) is practiced worldwide due to its advantages in conserving soil and water and improving crop productivity.15–17 These soil management practices along with increased crop residue retention are getting more attention in Bangladesh in the recent years.18 It has been demonstrated that NT could enhance NH3 volatilization compared with CT because of the increase in soil urease activity and the presence of crop residues under NT, while CT causes penetration of a fraction of the fertilizer N into soil shallow cracks.19,20 In addition, crop residue retention was reported to facilitate NH3 fluxes from corn fields.21 Biomass burning (wildfires, agricultural burns etc.) is the second largest contributor to atmospheric NH3, after agricultural management.6,22 Similarly, agricultural residue (rice and wheat straw) burning contributes to reactive N emissions (including NH3) in the Indo Gangetic Plain which is associated with effects on regional air quality.23 On the contrary, crop residue retention can help sequester reactive N minimizing the atmospheric N loss and can act as a source of plant nutrients.24 However, there is little information on the effect of ST and crop residue on NH3 fluxes in rice fields in the Eastern Gangetic Plains or how NH3 fluxes vary across this region with soil types, climate conditions, seasons and agricultural management practices. Furthermore, few experiments have reported NH3 volatilization losses in response to increased in N fertilization rates.25,26 Most of the data on NH3 volatilization originate from central China where soils are sandy loam and classified as Gleysol (FAO classification) and in Japan where soils are Gray Lowland soils i.e. Fulvisols.28 There is a great lack of NH3 volatilisation data from floodplain soils that are common across the Indo-Gangetic Plain. Thus, we hypothesized that reduced tillage with higher residue retention coupled with increased N fertilization will enhance NH3 fluxes and the EF. The specific objectives of this study were to evaluate the effects of tillage systems along with different residue levels and urea-N rates on NH3 fluxes and to estimate the NH3 EF in a typical wetland rice ecosystem.
2. Material and methods
2.1 Description of experimental site
The study was conducted at the Bangladesh Agricultural University (BAU) farm, Mymensingh (24° 71.60′ N, 90° 42.51′ E), Bangladesh. The BAU farm (ca. 200 ha) hosts the research fields of the Department of Soil Science in the south-western part of the farm. The experimental field has been under conventional tillage practices for a long time with negligible aboveground crop residue retention. Since January 2019 an annual rice-rice-rice pattern, a common cropping sequence on the floodplain soils of Bangladesh, has been followed. The region has a subtropical monsoon climate with a mean annual temperature of 26 °C, average annual rainfall of 1800 mm, and relative humidity of 85% (Weather Yard, BAU). The field site with a Non-calcareous Dark Grey Floodplain soil (Aeric Haplaquept in the U.S. Soil Taxonomy) is situated in Agro-ecological zone 9 (AEZ-9; Old Brahmaputra Floodplain soil)27 and is moderately drained with silt loam texture and near neutral pH (6.5). Mean monthly rainfall and temperature during the rice cropping season were 127.4 mm and 25.4 °C in 2019 and 171.2 mm and 26.8 °C in 2020, respectively (Fig. 1).
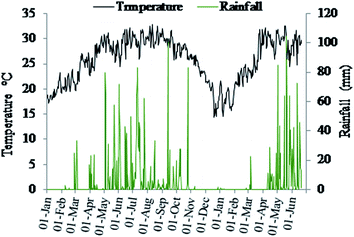 |
| Fig. 1 Daily weather data (rainfall and temperature) of the research field at Bangladesh Agricultural University in Mymensingh, Bangladesh from January 2019 to June 2020. | |
2.2 Soil characteristics
The soil (0–15 cm) was silt loam having 6.5 pH (water), 11.3 g soil organic C per kg,29 12 g N per kg,30 3.2 mg P per kg,31 34.8 mg NH4OAc extractable K per kg,32 and 10.5 mg CaCl2 extractable S per kg.33
2.3 Experimental design and management
In July 2018, the experiment was initiated with two soil disturbance levels (strip tillage, ST and conventional tillage, CT); two crop residue retention levels (low residue (LR): 15% and high residue (HR): 40%, by height) as well as three N fertilization rates (108, 144 and 180 kg N ha−1), with 144 kg N ha−1 being the recommended N fertilizer rate (FRG, 2018) as urea under an annual sequence of three rice crops (Oryza sativa L), called hereafter Boro rice-Transplanted Aus rice-Transplanted Aman rice. Boro rice was grown during January to May (mid-winter to pre-monsoon season), followed by Aus rice as a rainfed crop from June to August (monsoon), and then Aman rice from August to December (late monsoon to winter). The ST has been accomplished by a Versatile Multi-crop planter making 3 cm furrows for seeding or transplanting separated by 20 cm of undisturbed soil between rows.34 In the ST system, 15 and 40% residues (by height) of the previous crop were left on soil surface while in CT system the same amount of residue was incorporated into soil by repeated ploughing using a rotary tiller. The 15% retention was comparable to current practice of farmers. A split–split plot experiment was established with three replications for each treatment combination. Tillage treatment was assigned to the main plots with residue to the sub-plots and fertilizer to sub–sub plots. The size of each sub–sub plot was 10 m × 4.2 m with a separating bund between plots. Total number of plots was 36, including two tillage × two residue levels × three N rates × three replications.
Land preparation began in the last week of January for transplanting Boro rice seedlings, followed by transplanting of Aus rice in the last week of May and the transplanting of Aman rice seedlings in late August. Three seedlings were transplanted per hill with a 20 cm × 20 cm spacing. The varieties were BRRI dhan63, BINA dhan19 and BRRI dhan46, respectively. The recommended dose (RD) of fertilizers were 144 kg N, 21 kg P, 60 kg K, 8 kg S, 1.5 kg Zn per ha for Boro rice; 72 kg N, 7 kg P, 40 kg K, and 3 kg S per ha for Aus rice; and 90 kg N, 8.5 kg P, 50 kg K, 4 kg S, and 1 kg Zn per ha for Aman rice. The sources of N, P, K, S and Zn were urea, triple super phosphate, muriate of potash, gypsum and zinc sulphate, respectively.
Glyphosate (Round up®), a non-selective herbicide, was sprayed over the field at a rate of 1.85 kg ha−1 3 days before final land preparation. In addition, Pretilachlor (Superhit®, post emergence herbicide) was used at a rate of 450 g ha−1 at 7 days after transplanting of rice seedlings. Brifer 5G and Cidial 5G (ACI Bangladesh Ltd.) were applied as required to control rice insects. The rice fields were irrigated a day before the final land preparation and then when necessary to maintain standing water at about 3 cm above soil surface throughout the growing season.
2.4 Measurement of NH3 fluxes
The field measurement of NH3 was conducted during February to May 2019 in the first year of conversion of CT to ST and again in 2020 in the second year. In paddy rice, different techniques have been used to measure NH3 fluxes e.g., dynamic chamber method by Hayashi et al. (2006),35 continuous airflow enclosure method by Ye et al. (2011)36 and closed chamber method by Cheng et al. (2012).26 However, all the methods used the same principle of trapping the volatilized NH3 into a gas washing bottle containing an acid trap25 or cellulose filter impregnated with H3PO4.35 The NH3 trapped solution is then titrated with a standard acid to determine NH3 fluxes. In the dynamic flow-through chamber method, a vacuum pump is attached to draw fresh air through the acid trap which can add NH3 from outside the chamber resulting an over estimation of NH3 fluxes. To avoid over estimation of NH3 fluxes in the current study, an improved chamber-based technique was designed as follows. A closed chamber (PVC pipe, 20 cm diameter and 40 cm height) was inserted into the centre of each plot (10 m × 4.2 m) to a depth of 5 cm. After each urea application to each chamber, the airtight lid was placed on top of the base and connected, through a side inlet tube attached to another PVC pipe (2.5 m long and 2 cm diameter) which had an opening to the atmosphere. This inlet tube provides NH3 free air (2.5 m above the ground surface is deemed to be free of NH3 emitted from the field) from the atmosphere to the NH3 measurement chamber. Another side of the chamber was connected to an especially designed air tight flask containing the boric acid indicator solution (100 ml) to trap the NH3. This was designed in such a way to trap the NH3 entering from the chamber and let the additional air pass through an outlet pipe connected to the vacuum pump (Suppl. 1). The vacuum pump was used to pump air from the NH3 measurement chamber at 100 ml min−1 to mimic the natural air current. Due to trapping of NH3 in the boric acid indicator solution, the color changed from pink to green. After every 24 h, the indicator solution, when it turned pink to green, was titrated with 0.01 N H2SO4. Measurements were made from continuously flooded soil beginning from the first split application of urea followed by the second and third split application. In each case measurements continued until the fluxes were below detection limit.
2.5 NH3 fluxes and EF calculation
The NH3 fluxes were calculated following eqn (1). |  | (1) |
where, NH3 flux was measured as mg per m2 per day (titration was done after every 24 h); FBR is Final Burette Reading; IBR is Initial Burette Reading; 14.01 is molecular weight of N; 0.01 is normality of H2SO4; 1000 is unit conversion factor. The cumulative NH3 fluxes were calculated as the sum of NH3 fluxes on sampling days during the total sampling period.
We derived the EF (%) according to eqn (2).13,37
| 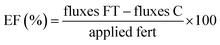 | (2) |
where EF (%) = emission factor, in %; fluxes FT = fluxes from fertilizer treatment (in kg N per ha per year); fluxes C = fluxes from control treatment (in kg N per ha per year); applied fert = amount of fertilizer applied (in kg N per ha per year). From a previous experimentation in this site, without fertilizer application (true control) no NH
3 fluxes were observed. In the current experiment, NH
3 fluxes from the sampling before urea application as well as 7–12 days after urea application was below the detection limit indicating that from a true control, NH
3 fluxes should be negligible.
2.6 Measurement of soil pH and redox potential
Soil pH was measured in the field every seven days during NH3 loss measurement using a portable pH meter (Direct Soil pH Portable Meter, HI12923; Hanna Instruments). The pH meter is designed to measure soil pH directly by inserting it into the soil (https://hanna-worldwide.com/ph-electrode-for-direct-soil-measurement-hi12923.html). Soil redox potential was measured at 3 and 7 cm below soil surface at every seven days using a water proof ORP meter (RE300, Extech Instruments).
2.7 Statistical analysis
A split–split plot three-way analysis of variance (ANOVA) was performed using tillage, residues and N rate as fixed variables. The distribution of data for normality was checked before ANOVA. Data were statistically analyzed to ascertain the significant differences for main effects and interactions among tillage, residue and N rate treatments. Post-hoc tests were performed to separate differences among N rates using the Tukey–Kramer multiple comparison Test. All statistical analyses were considered significant at p ≤ 0.05, unless otherwise mentioned. All the statistical analyses were performed on Statistics 10 and Jamovi1.0.0.0. (R Package).
3. Results
3.1 Time course of NH3 fluxes after urea application
During the sampling period in 2020, the NH3 emission peaks were observed on day 2–3, day 2 and day 1 after the urea application in first, second, and third split applications, respectively (Fig. 2). The timing and magnitude of NH3 emission peaks were similar in 2019 and 2020. The highest peak appeared to have been much greater in ST than that in CT in all the three urea splits, being 113.4, 131.1 and 140.6 mg N m−2 d−1 in ST and 85.7, 78.4, and 100.5 mg N m−2 d−1 in CT in the first, second and third splits, respectively in 2020. In 2019, the corresponding values were similar for ST and CT, namely: 104.4, 108.6 and 119.6 mg N m−2 d−1 in ST and 105.4, 115.2, and 100.5 mg N m−2 d−1 in CT in the first, second and third split, respectively. The NH3 fluxes were higher in the third split than in the first and second splits across tillage systems, residue retentions and fertilizer rates (Fig. 2). While, NH3 emission peaks appeared on the same days in all splits for ST and CT, values declined to below detection earlier in CT than in ST causing shorter emission intervals in CT than in ST. Across tillage systems, residue retentions and fertilizer rates, the NH3 emissions declined to below the detection limit on day 12 after the first split, day 7 after the second split and day 8 after the third split of urea application. The highest peak in the third split was observed on day 1 following the order: 180 kg N ha−1 > 144 kg N ha−1 > 108 kg N ha−1. Values were 79.4, 103.0 and 122.9 mg N m−2 d−1 in the first split, 73.1, 118.2 and 129.6 mg N m−2 d−1 in the second split and 94.3, 117.4 and 155.6 mg N m−2 d−1 in the third split in 108 kg N ha−1, 144 kg N ha−1 and 180 kg N ha−1, respectively in 2020 while they were 65.9, 87.4 and 117.2 mg N m−2 d−1 in the first split, 69.0, 89.3 and 124.4 mg N m−2 d−1 in the second split and 117.2, 124.4 and 149.9 mg N m−2 d−1 in the third split in 108 kg N ha−1, 144 kg N ha−1 and 180 kg N ha−1, respectively in 2019.
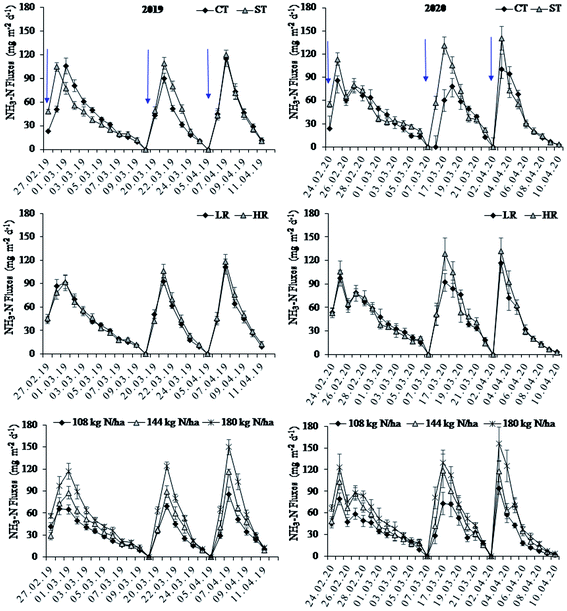 |
| Fig. 2 Daily NH3 fluxes (mean ± SE; n = 3) in conventional (CT) and strip (ST) tillage systems; low residue (LR) and high residue (HR) retention; and three N application rates (108 kg N ha−1, 144 kg N ha−1, 180 kg N ha−1) in 2019 and 2020; arrow shows the day of split application of urea. | |
3.2 Mean and cumulative NH3 fluxes after urea application
The mean NH3 fluxes were 18.5% higher in ST than in CT (p < 0.05) in 2020, but there was no significant difference in 2019 (Table 1). The NH3 fluxes significantly increased with N fertilization rates in both 2019 and 2020 (p < 0.001). More specifically, in 2019 NH3 fluxes were increased by 32.6% and 77.4.8% with increase in N fertilization rate from 108 to 144 and 180 kg ha−1, respectively. In 2020, increasing N fertilizer to 180 kg N ha−1 significantly increased the NH3 fluxes by 62.4% and 32.5% compared with the 108 and 144 kg N ha−1 treatment, respectively. However, the mean NH3 fluxes was significantly affected by the three way interaction of tillage × residue × N rate in both years (P < 0.05, Table 1). In 2019, increasing N rate from 108 to 144 kg ha−1 did not significantly affect the mean NH3 fluxes in the treatment of CT with LR, but significantly increased it by 32–41% in other treatments, while increasing the N rate from 144 to 180 kg ha−1 significantly increased the mean NH3 fluxes by 24–46% for all the treatments. In contrast, in 2020, the mean NH3 fluxes were only significantly increased with the increasing N rate from 108 to 180 kg ha−1 in all the treatments, with the exception of the treatment of ST with LR which had no significant difference among N rates.
Table 1 The effect of tillage systems (conventional tillage, CT and strip tillage, ST), residue levels (low residue, LR and high residue, HR) and N application rates (108, 144, and 180 kg ha−1) on mean and cumulative NH3 fluxes and emission factor for wetland rice in 2019 and 2020 (n = 3; mean ± SE)a
Tillage |
Residue |
N rate (kg N ha−1) |
2019 |
2020 |
Mean NH3 fluxes (mg m−2 d−1) |
Cumulative NH3 emissions (mg m−2) |
Mean emission factor (EF) (%) |
Mean NH3 emission (mg m−2 d−1) |
Cumulative NH3 emission (mg m−2) |
Mean emission factor (EF) (%) |
NS, *, **, *** indicate P > 0.05, P < 0.05, P < 0.01, P < 0.001, respectively.
|
CT |
LR |
108 |
33.67 ± 7.28 |
1582 ± 17.40 |
15 ± 0.03 |
32.64 ± 3.20 |
1534 ± 10.62 |
14 ± 0.02 |
144 |
42.05 ± 1.14 |
1976 ± 17.10 |
14 ± 0.01 |
46.81 ± 0.44 |
2200 ± 9.72 |
15 ± 0.01 |
180 |
61.27 ± 1.89 |
2879 ± 21.50 |
16 ± 0.01 |
60.60 ± 10.6 |
2848 ± 15.31 |
16 ± 0.03 |
HR |
108 |
35.82 ± 3.38 |
1684 ± 11.88 |
16 ± 0.01 |
37.80 ± 3.49 |
1777 ± 10.08 |
16 ± 0.02 |
144 |
47.49 ± 2.22 |
2232 ± 8.37 |
15 ± 0.01 |
46.11 ± 9.74 |
2167 ± 11.80 |
15 ± 0.03 |
180 |
63.76 ± 1.69 |
2997 ± 25.32 |
17 ± 0.01 |
63.90 ± 14.7 |
3002 ± 19.86 |
17 ± 0.04 |
ST |
LR |
108 |
35.63 ± 1.27 |
1675 ± 9.37 |
15 ± 0.01 |
45.03 ± 2.94 |
2117 ± 8.80 |
19 ± 0.01 |
144 |
50.08 ± 3.85 |
2354 ± 17.89 |
16 ± 0.01 |
52.46 ± 3.09 |
2466 ± 11.94 |
17 ± 0.01 |
180 |
62.13 ± 0.85 |
2920 ± 21.41 |
16 ± 0.01 |
66.07 ± 7.25 |
3105 ± 10.18 |
17 ± 0.02 |
HR |
108 |
38.77 ± 3.51 |
1822 ± 14.80 |
17 ± 0.02 |
47.95 ± 5.51 |
2254 ± 11.32 |
21 ± 0.02 |
144 |
51.16 ± 2.11 |
2405 ± 15.30 |
17 ± 0.01 |
54.93 ± 8.00 |
2582 ± 14.83 |
18 ± 0.03 |
180 |
66.76 ± 1.30 |
3138 ± 17.89 |
18 ± 0.01 |
74.86 ± 8.19 |
3518 ± 17.69 |
20 ± 0.02 |
LSD |
9.51 |
448.9 |
4.1 |
22.81 |
1072.4 |
4.6 |
Statistical analysis |
Tillage |
NS |
NS |
NS |
* |
* |
* |
Residue |
NS |
NS |
NS |
NS |
NS |
NS |
N rate |
** |
** |
NS |
** |
** |
NS |
Tillage × residue |
* |
* |
NS |
NS |
NS |
* |
Tillage × N rate |
* |
* |
NS |
NS |
NS |
* |
Residue × N rate |
* |
* |
NS |
* |
* |
NS |
Tillage × residue × N rate |
* |
* |
NS |
* |
* |
NS |
Similar to mean NH3 fluxes, cumulative NH3 fluxes over the entire sampling period were significantly higher in ST than in CT in 2020, but the effect was non-significant in 2019 (Fig. 3). In both years, cumulative NH3 fluxes were similar in HR and LR (p > 0.05). By contrast, the cumulative NH3 fluxes were significantly higher in 180 kg N ha−1 and 144 kg N ha−1 than in 108 kg N ha−1, while the former two were also different (p < 0.001) in both years. Like the mean NH3 fluxes, interaction effects on cumulative NH3 fluxes of tillage vs. residue vs. N rate were significant (Table 1). There were higher cumulative NH3 fluxes in 180 kg N ha−1 coupled with either HR or LR and ST or CT than any other treatment combinations. The lowest N rate (108 kg N ha−1) had lower cumulative NH3 fluxes than all other treatment combinations.
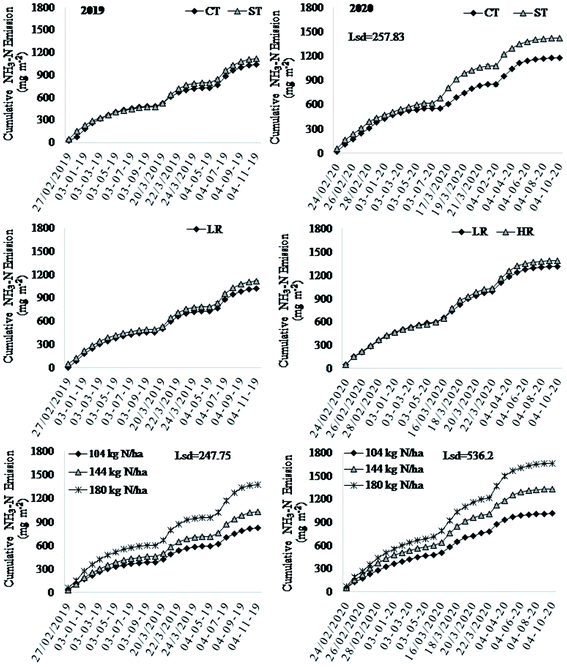 |
| Fig. 3 Cumulative NH3 fluxes in conventional tillage (CT) and strip tillage (ST) systems; low residue (LR) and high residue (HR) levels; three N application rates (108 kg N ha−1, 144 kg N ha−1, 180 kg N ha−1) in 2019 and 2020. | |
In CT, cumulative NH3 fluxes ranged from 1582 in 108 kg N ha−1 to 2879 mg N m−2 in 180 kg N ha−1 under LR, and 1777 in 108 kg N ha−1 to 3002 mg N m−2 in 180 kg N ha−1 under HR. In ST, cumulative NH3 fluxes ranged from 2117 in 108 kg N ha−1 to 3105 mg N m−2 in 180 kg N ha−1 under LR, and 2254 in 108 kg N ha−1 to 3518 mg N m−2 in 180 kg N ha−1 under HR (Table 1). In 2020, cumulative NH3 fluxes were significantly higher in 180 kg N ha−1 than in 108 kg N ha−1 and 144 kg N ha−1, where the latter two were alike (Fig. 3).
3.3 Effect of management on NH3 emission factors
In 2019, no treatment combinations affected NH3 EF (Table 1). In 2020, mean NH3 EF of 19% was significantly higher in ST than 16% in CT (Table 1). Unlike, the tillage effect, NH3 EF was statistically similar between residue levels and between N fertilizer rates in 2020. However, interaction effects of tillage vs. residue on mean NH3 EF were significant, since there was a lower EF in CT coupled with LR (15%) than all other treatment combinations (Table 1), while ST with HR showed the highest EF (20%). Likewise, the interaction effects of tillage vs. N fertilizer rate were significant, where 108 and 144 kg N ha−1 in CT had the lower NH3 EF than all other treatment combinations. The lowest N rate (108 kg N ha−1) in ST had the highest NH3 EF (20%) which was similar to 144 and 180 kg N ha−1 in the same tillage and to 180 kg N ha−1 in CT (Table 1).
3.4 Management effects on soil pH, redox potential and mineral N content and their relationships with NH3 fluxes
Conversion of CT soil management to ST increased soil pH with HR (p < 0.05) (Table 2). That is, there was higher soil pH in ST coupled with HR (6.69) than the CT with HR (6.59; p < 0.05). Interaction effects of tillage and fertilization rates were also significant since there was higher pH in ST coupled with 180 kg N ha−1 than ST coupled with 144 kg N ha−1, which was similar to CT coupled with 180 kg N ha−1 and 144 kg N ha−1. The ST coupled with 108 kg N ha−1 had the lower pH than all other combinations of tillage and N fertilizer rates but equal to CT coupled with 108 kg N ha−1. Likewise, crop residue vs. fertilizer rate had significant effects on soil pH showing the higher pH in 180 kg N ha−1 with either HR or LR and lower pH in 108 kg N ha−1 with either HR or LR.
Table 2 Soil pH, redox potential and mineral N content in different treatments at 0–15 cm soil depth in 2020, measured while field measurement of NH3 fluxes were done; mean ± SE (n = 3)a
Tillage |
Residue |
N fertilizer rate (kg N ha−1) |
pH |
Redox potential (mV) |
Available soil NH4+ concentration (mg N kg−1) |
Available soil NO3− concentration (mg N kg−1) |
3 cm below soil surface |
7 cm below soil surface |
NS and * indicate P > 0.05, P < 0.05, respectively.
|
CT |
LR |
108 |
6.50 ± 0.05 |
44.1 ± 7.53 |
3.77 ± 10.40 |
7.66 ± 1.06 |
3.58 ± 0.16 |
144 |
6.67 ± 0.01 |
46.9 ± 3.66 |
1.56 ± 6.20 |
7.97 ± 0.67 |
3.86 ± 0.22 |
180 |
6.68 ± 0.05 |
55.4 ± 1.88 |
0.56 ± 0.23 |
9.59 ± 0.51 |
4.36 ± 0.37 |
HR |
108 |
6.52 ± 0.03 |
42.7 ± 5.52 |
−3.54 ± 2.85 |
7.10 ± 1.38 |
3.49 ± 0.58 |
144 |
6.60 ± 0.02 |
51.9 ± 0.08 |
11.26 ± 2.64 |
8.06 ± 0.93 |
5.42 ± 1.51 |
180 |
6.65 ± 0.08 |
48.1 ± 4.84 |
6.36 ± 8.78 |
8.72 ± 1.98 |
5.45 ± 1.51 |
ST |
LR |
108 |
6.48 ± 0.06 |
−39.0 ± 12.37 |
−32.18 ± 3.49 |
10.18 ± 1.08 |
3.61 ± 0.22 |
144 |
6.64 ± 0.05 |
−37.2 ± 1.75 |
−31.33 ± 5.41 |
10.27 ± 0.57 |
4.20 ± 0.67 |
180 |
6.80 ± 0.07 |
−27.5 ± 11.25 |
−30.97 ± 4.01 |
10.65 ± 0.33 |
4.30 ± 0.11 |
HR |
108 |
6.56 ± 0.02 |
−38.9 ± 3.58 |
−33.49 ± 2.20 |
5.76 ± 0.97 |
3.83 ± 0.38 |
144 |
6.63 ± 0.06 |
−33.4 ± 3.42 |
−28.67 ± 3.64 |
9.87 ± 0.57 |
4.48 ± 0.35 |
180 |
6.86 ± 0.04 |
−22.0 ± 14.08 |
−27.74 ± 3.44 |
11.61 ± 1.46 |
5.23 ± 0.39 |
LSD |
0.04 |
15.03 |
8.43 |
2.93 |
1.52 |
Statistical analysis |
Tillage |
* |
* |
* |
NS |
NS |
Residue |
NS |
NS |
NS |
NS |
NS |
Fertilizer |
* |
NS |
NS |
* |
* |
Tillage × residue |
* |
* |
* |
NS |
NS |
Tillage × fertilizer |
* |
* |
* |
* |
NS |
Residue × fertilizer |
* |
NS |
NS |
* |
* |
Tillage × residue × fertilizer |
NS |
* |
* |
* |
NS |
Soil redox potentials at both soil depths (3 and 7 cm below soil surface) were significantly influenced by the interactions between tillage × residue × N fertilization rates (Table 2). Soil redox potential at both depths were significantly higher in CT and residue combinations than in ST with fertilizer or residue combinations (p < 0.05). Soil NH4+ concentration was the highest in 180 kg N ha−1 with either HR or LR in ST and the lowest in 108 kg N ha−1 in HR with either ST or CT. The 144 kg N ha−1 or 180 kg N ha−1 in ST with either residue was similar to 180 kg N ha−1 in CT with HR or LR, which was higher than the 108 kg N ha−1 in ST or CT (p < 0.05). Tillage and residue management effects on soil NO3−–N content were non-significant (p > 0.05), while N fertilization rate significantly increased soil NO3−–N content (p < 0.05). The interaction effects of residue vs. N fertilization rate resulted from higher NO3−–N content in 144 kg N ha−1 and 180 kg N ha−1 with either residue levels than in 108 kg N ha−1 in LR or HR. The relationships between the NH3 fluxes and measured soil properties were positive and significant; soil pH (R2 = 0.61; p < 0.01), NH4+–N (R2 = 0.37; p < 0.05) and NO3−–N (R2 = 0.35; p < 0.05). The NH3 fluxes were not correlated with the redox potential (p > 0.05).
4. Discussion
4.1 NH3 fluxes under different tillage systems
The highest NH3 emission peaks occurred within 1–3 days after urea application because the saturated soil supported rapid hydrolysis of the applied urea. Immediately after urea application, if soil pH rose near the urea granules this would create favourable conditions for NH4+ conversion to NH3.38 In this study, we found that NH3 fluxes were significantly higher in ST compared to CT in 2020 which is in agreement with Mkhabela et al., (2008) who observed higher NH3 fluxes from soil after ST or no tillage than after CT.19 Liu et al. (2018) who measured NH3 fluxes in the whole rice growing period found that only no tillage and ST markedly increased NH3 fluxes from basal fertilizer by 10–14% compared to CT, but had no obvious effects on total volatilization during the whole season.39 The discrepancy may be attributed to the duration of ST practice in the field.40 In our study, cumulative NH3 fluxes were higher in ST by 7.2% and 18.5% over the CT in 2019 and 2020, respectively. The higher fluxes in ST can be attributable to greater urease activities in soil surface resulting in higher concentration of NH4+ in the soil and floodwater from hydrolyzed N fertilizer which diffused to the water–atmosphere interface.41,42 Moreover, the contact of fertilizer particles and the soil may be reduced by the residues left on soil surface after ST, which means reduced adsorption of NH4+ from hydrolyzed fertilizer N by soil particles under ST. Part of the fertilizer N may diffuse into superficial soil fractures under the puddled soil condition in CT, which may result in lower NH3 fluxes at the early stage of crop growth under CT than under ST.20 The NH3 flux peaks were observed on 1–3 days after each split of N fertilizer application (Fig. 2) may be related to the rate of enzymatic hydrolysis of urea in the applied N.39,41,43
4.2 NH3 fluxes under different residue levels
The effect of residue retention level was not significant on net NH3 fluxes in our study. The results indicated that the effect of residue retention may take several years to develop, in line with the duration it takes for increases in soil organic matter. Alam et al. (2018) reported 65% increase in SOM and Alam and Bell (2020) reported a similar increase in TN in soils after 5 years under ST and increased residue.24,44 It would be useful to test effects of these soil management practices on NH3 fluxes over a longer period of time than the 2 years of the current study. In both the present study and that reported by Alam et al. (2018) the maximum residue retention was 50% or less, indicating that the impact of residue on NH3 may be underestimated.44 The in situ incorporation of rice straw in the soil has been reported to increase recycling of nutrients and increasing soil organic carbon and contribute to yields of the subsequent crop.45,46 The production of three rice crops annually results in the production of large quantities of straw with a short turn over period between the consecutive crops which results in limited decomposition. Generally, straw mass reduction and nutrient release of rice straw is faster in early stage of decomposition (0–14 days after decomposition), where available carbohydrates and amines are the preferential substrates for decomposers involved.47 Gram-positive bacteria are the quantitatively predominant microorganisms in early stage of decomposition. On the other hand, fungi and actinomycetes play a major role in decomposition of relatively resistant substances at the late decomposition stage. The straw retained acts both as a source of phosphorus and potassium in short term and as a source of N and carbon in the long term during the rice growing season.48 The effect of residue level on the available NH4+ concentration as well as NH3 fluxes was not large in the present study, possibly due to slow decomposition of rice straw at high C
:
N ratio, the readily available fertilizer N for the associated microbes, the short turn over periods of the residue between the crops, and the partial retention of crop residue from the previous crop even in the HR treatment.
4.3 NH3 fluxes under different fertilizer rates
A stimulation of NH3 fluxes resulting from N fertilization in submerged soils has been reported before.25,41,49 The response of urea hydrolysis and corresponding NH3 fluxes after urea application were very rapid because of the microbial stimulation from enhanced mineral N which was very low before N application. The low mineral N content is attributable to the loss of mineral N during land preparation and seedling establishment until urea application after day 7. In our study we found that NH3 losses increased significantly with enhanced N fertilizer supply in both the tillage systems and residues which is in line with previous studies.25,26 The potential for NH3 fluxes following urea application is high because of its rapid hydrolysis to ammoniacal N and of the increased pH in the vicinity of the dissolved urea granules.50 The ratio of NH3 fluxes to the N application level increased with the N application rate.38 This was mainly because of the pronounced increase in the NH4+–N concentration in surface water as reported before.51 Flood water has a limited ability to buffer solution NH4+ or pH which rises due to diurnal photosynthesis of algae and together these two water properties result in higher NH3 fluxes loss in rice field.50 Urea application shortly after transplanting when uptake is still small (i.e. first split urea application) leaves surplus ammoniacal N that is prone to volatilization.52,53 Furthermore, a history of excessive N fertilizer application creates a situation conducive for NH3 fluxes by reducing acidification.54 Besides, SOC and soil TN are closely associated and most N taken up originates from soil organic matter (SOM) mineralization.55 High soil TN improves the capacity of soil to supply N to the crop and reduces the N fertilizer requirement, thus leading to more fertilizer N loss if N application supply remains unchanged.52 Plant residue incorporated from previous crops is also a source of N and can enhance soil TN and at the same time decrease the uptake of fertilizer N resulting in greater NH3 fluxes with higher N fertilizer supply.
4.4 Management effects on NH3 emission factors
In the present study, the estimated mean NH3 EF was 15.9 and 17.2% in 2019 and 2020, irrespective of tillage, which is about 45 and 56% higher than the IPCC default Tier 1 FracGASF value of 11%.56 But the present result corresponds to meta-analyses which reported that the average proportion of N lost through NH3 fluxes from applied N fertilizer was 17% (n = 78) and 16% (n = 265), respectively.57,58 Generally estimates range from 11–22% which agrees well with our results.13,26,37 The discrepancy in results may be attributed to the differences in agricultural management practices, climatic conditions, soil properties and regions.40 The mean NH3 EF was significantly higher in ST (17%) than in CT (15%) which is a bit higher than the Chinese report of 13%.39–41 The causal factor may be greater urease activity in surface soil under ST or no tillage than in CT because SOM, microbial biomass and urease activities are higher in reduced or no tillage than conventional tillage.59 In addition, more NH4+ may be adsorbed on clay surfaces after CT as there is a greater chance of adsorption NH4+ from the broadcasted urea in CT while after ST, urea contact with soil is hindered by the straw retained on the soil surface. The lack of effect of residue levels on EF of NH3 fluxes can be attributed to the slow decomposition of rice straw. However, only 40% residue was retained in HR which means the potential residue effect was probably underestimated. The NH3 EF significantly increased with the increment in fertilizer N rate which was reported earlier.26 But in our study the highest EF was estimated in low fertilizer rate compared to the recommended and higher N dose which is opposite to the previous result. It may be due to separation of the amount of NH3 by the greater amount of N fertilizer applied which lowers the value of EF. The highest cumulative fluxes and EF were observed during the seedling stage (first split urea application) followed by booting stage and maximum tillering stage. This may be attributed to the very limited root expansion of the rice seedlings during the first split urea application. As a result, plant uptake of NH4+ from the applied urea fertilizer was very low which increases the cumulative NH3 fluxes and EF as well as the duration to about 12 days. During the booting stage, highest NH3 peaks were observed and attributed to high temperature (36 °C) (Fig. 3) that result in rapid hydrolysis of urea. However, since plant demand and uptake of N is high during the booting stage,60 the cumulative NH3 emission did not exceed the amount lost in the first split. The lowest EF and cumulative NH3 during the maximum tillering stage was a further indication of the impact of rapid NH4+ uptake by plants on NH3 emissions.
4.5 Relationships between soil pH, redox potential and mineral N content with NH3 fluxes
The NH3 fluxes were positively correlated with soil pH (r = 0.61; p < 0.05) and NH4+–N content (r = 0.54; p < 0.05) (Fig. 4), which is in agreement with other studies.38,50 Higher NH3 fluxes from soils with higher N fertilization rate was mainly driven by the reduced soil conditions and corresponding higher soil pH, because these soil conditions support a higher NH4+–N production. Likewise, soils in ST were more reduced and had higher soil pH than in CT causing higher NH3 fluxes. The NH3 fluxes were also positively correlated with the NO3−–N content, which is not surprising because the higher NO3−–N in soil was associated with higher N fertilization rate, as was NH4+–N content. It suggests that after urea application hydrolysis produces NH4+–N that is simultaneously transformed to both NH3, by volatilization, and NO3−–N, by nitrification, which is reported by other studies.61 The NO3−–N production in rice root rhizosphere have been reported previously and attributed to radial oxygen loss from rice aerenchyma to the rhizosphere where it oxidises NH4+ to NO3−–N, which rice roots absorb and alleviate NH4+–N toxicity effects that can occur under anaerobic conditions.62 Our results show that higher NH3 fluxes under diversified management practices are associated with possibly local higher soil pH coupled with reduced soils conditions and higher N applications. In the case of ST with either residue level greater NH3 EF was associated with higher soil pH, NH4+–N and more reduced soil conditions than CT (Table 2).
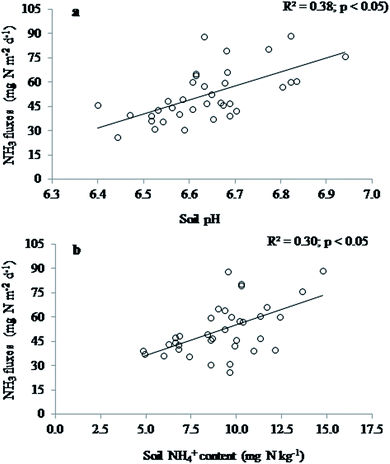 |
| Fig. 4 Relationship between NH3 fluxes and soil pH (a) and soil NH4+ content (b); n = 36. | |
5. Conclusions
The estimated NH3 EFs in this study were 16% in 2019 and 17% in 2020, which are 45 and 56% higher than the default value reported by the IPCC for wetland rice. Clearly, for the Eastern Gangetic plain, NH3 fluxes loss from floodplain soils is a more significant loss pathway than previously assumed with significant implications for N use efficiency and N balance in rice-based cropping systems. Our results also show that minimum soil disturbance caused a further small increase in NH3 fluxes in the second year of the study (2020), but not in the first year (2019). The difference was most pronounced at 108 kg N ha−1 where ST tillage had the highest EF (21%) whereas CT had the lowest (14%). Highest net NH3 fluxes was observed at seedling establishment stage of urea application compared to the booting and maximum tillering stage. Fertilizer N use is trending upwards in the last few decades due to declining organic matter as well as fertility of the soil. About 2.37 million tonnes of N fertilizer as urea was applied in 2017–18 in Bangladesh which costs about 50.7 million US$. Considering the emission factor, NH3 fluxes is consuming 8.12 million US$ every year and the loss may be higher in the future. It clearly highlights the urgent need for mitigation strategies for N losses via NH3 fluxes in wetland rice ecosystems.
Conflicts of interest
There are no conflicts to declare.
Acknowledgements
The research was funded by a Krishi Goveshona Foundation (KGF) project administered by Bangladesh Agricultural Research Council (BARC) in association with the Australian Centre for International Agricultural Research (ACIAR: Project LWR 2016/136).
References
- B. A. Linquist, M. M. Anders, M. A. Adviento-Borbe, R. L. Chaney, L. L. Nalley, E. F. Da Rosa and C. Kessel, Reducing greenhouse gas emissions, water use, and grain arsenic levels in rice systems, Glob. Chang. Biol., 2015, 21, 407–417, DOI:10.1111/gcb.12701
.
- L. Xia, C. Ti, B. Li, Y. Xia and X. Yan, Greenhouse gas emissions and reactive nitrogen releases during the life-cycles of staple food production in China and their mitigation potential, Sci. Total Environ., 2016, 556, 116–125, DOI:10.1016/j.scitotenv.2016.02.204
.
-
IFDC, Mitigating poverty and environmental degradation through nutrient management in south asia, IFDC report (March 2007), 2007 Search PubMed
.
- Z. L. Zhu, G. X. Cai, J. R. Simpson, S. L. Zhang, D. L. Chen, A. V. Jackson and J. R. Freney, Processes of nitrogen loss from fertilizers applied to flooded rice fields on a calcareous soil in north-central China, Fert. Res., 1989, 18, 101–115, DOI:10.1007/bf01049507
.
- X. Zhao, Y. Zhou, S. Wang, G. Xing, W. Shi, R. Xu and Z. Zhu, Nitrogen balance in a highly fertilized rice-wheat double-cropping system in southern China, Soil Sci. Soc. Am. J., 2012, 76, 1068–1078, DOI:10.2136/sssaj2011.0236
.
- J. Kuttippurath, A. Singh, S. P. Dash, N. Mallick, C. Clerbaux, M. Van Damme, L. Clarisse, P. F. Coheur, S. Raj, K. Abbhishek and H. Varikoden, Record high levels of atmospheric ammonia over India: Spatial and temporal analyses, Sci. Total Environ., 2020, 740, 139986, DOI:10.1016/j.scitotenv.2020.139986
.
- S. B. Peng, J. L. Huang, X. H. Zhong, J. C. Yang, G. H. Wang, Y. B. Zhou, F. S. Zhang, Q. S. Zhu, R. Buresh and C. Witt, Research strategy in improving fertilizer-nitrogen use efficiency of irrigated rice in China, Sci. Agric. Sin., 2002, 35(9), 1095–1103 Search PubMed
.
- X. Zheng, C. Fu, X. Xu, X. Yan, Y. Huang, S. Han, F. Hu and G. Chen, The Asian nitrogen cycle case study, Ambio, 2002, 31, 79–87 CrossRef
.
- G. M. Tian, Z. C. Cai, J. H. Cao and X. P. Li, Ammonia volatilization from paddy field and its affecting factors in Zhengjiang hilly region, Acta Pedol. Sin., 2001, 38(3), 321–324, DOI:10.1155/2014/417605
.
- X. H. Fan, Y. S. Song, D. X. Lin, L. Z. Yang and J. F. Luo, Ammonia volatilization losses and 15N balance from urea applied to rice on a paddy soil, J. Environ. Sci., 2006, 18, 299–303 CAS
.
-
IPCC, International Panel for Climate Change, in Guidelines for National Greenhouse Gas Inventories, 2006, vol. 4, p. 23, http://www.ipcc-nggip.iges.or.jp/public/2006gl/index.htm Search PubMed
.
- E. Stokstad, Ammonia pollution from farming may exact hefty health costs, Science, 2014, 343, 238, DOI:10.1126/science.343.6168.238
.
- A. M. Mazzetto, D. Styles, J. Gibbons, C. Arndt, T. Misselbrook and D. Chadwick, Region-specific emission factors for Brazil increase the estimate of nitrous oxide emissions from nitrogen fertiliser application by 21%, Atmos. Environ., 2020, 230, 117506, DOI:10.1016/j.atmosenv.2020.117506
.
- A. F. Bouwman, Direct emission of nitrous oxide from agricultural soils, Nutr. Cycling Agroecosyst., 1996, 46, 53–70, DOI:10.1007/bf00210224
.
- H. L. Zhang, R. Lal, X. Zhao, J. F. Xue and F. Chen, Opportunities and challenges of soil carbon sequestration by conservation agriculture in China, Adv. Agron., 2014, 124, 1–36, DOI:10.1016/B978-0-12-800138-7.00001-2
.
- C. M. Pittelkow, X. Liang, B. A. Linquist, K. J. Van Groenigen, J. Lee, M. E. Lundy, N. Van Gestel, J. Six, R. T. Venterea and C. V. Kessel, Productivity limits and potentials of the principles of conservation agriculture, Nature, 2015, 517, 365–368, DOI:10.1038/nature13809
.
- C. M. Pittelkow, B. A. Linquist, M. E. Lundy, X. Q. Liang, K. J. van Groenigen, J. Lee, N. Van Gestel, J. Six, R. T. Venterea and C. V. Kessel, When does no-till yield more? A global meta-analysis, Field Crops Res., 2015, 183, 156–168, DOI:10.1016/j.fcr.2015.07.020
.
- R. W. Bell, M. E. Haque, M. Jahiruddin, M. M. Rahman, M. Begum, M. A. M. Miah, M. A. Islam, M. A. Hossen, N. Salahin, T. Zahan, M. M. Hossain, M. K. Alam and M. N. H. Mahmud, Conservation Agriculture for rice-based intensive cropping by smallholders in the Eastern Gangetic Plain, Agriculture, 2019, 9, 5, DOI:10.3390/agriculture9010005
.
- M. S. Mkhabela, A. Madani, R. Gordon, D. Burton, D. Cudmore, A. Elmi and W. Hart, Gaseous and leaching nitrogen losses from no-tillage and conventional tillage systems following surface application of cattle manure, Soil Tillage Res., 2008, 98, 187–199, DOI:10.1016/j.still.2007.12.005
.
- P. Rochette, D. A. Angers, M. H. Chantigny, J. D. MacDonald, N. Bissonnette and N. Bertrand, Ammonia volatilization following surface application of urea to tilled and no-till soils: a laboratory comparison, Soil Tillage Res., 2009, 103, 310–315, DOI:10.1016/j.still.2008.10.028
.
- T. Al-Kanani and A. F. MacKenzie, Effect of tillage practices and hay straw on ammonia volatilization from nitrogen fertilizer solutions, Can. J. Soil Sci., 1992, 72, 145–157, DOI:10.4141/cjss92-014
.
- A. F. Bouwman, D. S. Lee, W. A. H. Asman, F. J. Dentener, K. W. Van Der Hoek and J. G. J. Olivier, A global high-resolution emission inventory for ammonia, Global Biogeochem. Cycles, 1997, 11, 561–587, DOI:10.1029/97GB02266
.
- C. D. Bray, W. H. Battye and V. P. Aneja, The role of biomass burning agricultural emissions in the Indo-Gangetic Plains on the air quality in New Delhi, India, Atmos. Environ., 2019, 218, 116983, DOI:10.1016/j.atmosenv.2019.116983
.
- M. K. Alam, R. W. Bell, M. E. Haque, M. A. Islam and M. A. Kader, Soil nitrogen storage and availability to crops are increased by conservation agriculture practices in rice-based cropping systems in the Eastern Gangetic Plains, Field Crops Res., 2020, 250, 107764, DOI:10.1016/j.fcr.2020.107764
.
- S. Huang, W. Lv, S. Bloszies, Q. Shi, X. Pan and Y. Zeng, Effects of fertilizer management practices on yield-scaled ammonia emissions from croplands in China: a meta-analysis, Field Crops Res., 2016, 192, 118–125, DOI:10.1016/j.fcr.2016.04.023
.
- L. Z. Cheng, D. Q. Gen, Y. S. Chao, W. U. Fu-guan, J. Yu-shu, C. Jing-dou, X. U. Lu-sheng, Z. Hong-cheng, H. Zhong-yang, X. U. Ke and W. Hai-yan, Effects of Nitrogen Application Levels on Ammonia Volatilization and Nitrogen Utilization during Rice Growing Season, Ric. Sci., 2012, 19(2), 125–134, DOI:10.1016/S1672-6308(12)60031-6
.
-
JSP, Unified Soil Classification System of Japan, 2nd approximation, Japanese Society of Pedology (JSP), Hakuyu-sya, Tokyo, 2002 Search PubMed
.
-
FAO, Land Resources Appraisal of Bangladesh for Agricultural Development Report 2, Agro-ecological Regions of Bangladesh UNDP, FAO, 1988, pp. 212–221 Search PubMed
.
-
D. W. Nelson and L. E. Sommer, Total Carbon, Organic Carbon and Organic Matter, in Methods of soil Analysis, Part 2, ed. A. L. Page, R. H. Miller and D. R. Keeney, ASA and SSSA, Madison, WI, USA, 1982, pp. 539–579 Search PubMed
.
-
J. M. Bremner and C. S. Mulvaney, Nitrogen-Total, in Methods of Soil Analysis, Part 2, ed. A. L. Page, R. H. Miller and D. R. Keeney, ASA and SSSA, Madison, WI, USA, 1982, pp. 595–624 Search PubMed
.
-
S. R. Olsen and L. E. Sommer, Phosphorus, in Methods of Soil Analysis, Part 2, ed. A. L. Page, R. H. Miller and D. R. Keeney, ASA and SSSA, Madison, WI, USA, 1982, pp. 403–430 Search PubMed
.
-
D. Knudsen, G. A. Peterson and P. F. Pratt, Lithium, sodium and potassium, in Methods of Soil Analysis, Part 2, ed. A. L. Page, R. H. Miller and D. R. Keeney, ASA and SSSA, Madison, WI, USA, 1982, pp. 225–245 Search PubMed
.
- R. L. Fox, R. A. Olson and H. F. Rhoades, Evaluating the sulfur status of soils by plants and soil tests, Soil Sci. Soc. Am. Proc., 1964, 28, 243–246, DOI:10.2136/sssaj1964.03615995002800020034x
.
- M. E. Haque, R. W. Bell, A. K. M. S. Islam, K. D. Sayre and M. M. Hossain, An innovative Versatile Multi-crop Planter for crop establishment using two-wheel tractors, Agril. Mech. Asia, Africa Latin. Am., 2017, 48, 33–37 Search PubMed
.
- K. Hayashi, S. Nishimura and K. Yagi, Ammonia volatilization from the surface of a Japanese paddy field during rice cultivation, Soil Sci. Plant Nutr., 2006, 52, 545–555, DOI:10.1111/j.1747-0765.2006.00053.x
.
- S. C. Ye, Z. C. Lin, Q. G. Dai, Y. S. Jia, H. Y. Gu, J. D. Chen, L. S. Xu, F. G. Wu, H. C. Zhang, Z. Y. Hu, K. Xu and H. Y. Wei, Effects of nitrogen application rate on ammonia volatilization and nitrogen utilization in rice growing season, Chin. J. Rice Sci., 2011, 25(1), 71–78, DOI:10.1016/S1672-6308(13)60117-1
.
- H. Wang, D. Zhang, Y. Zhang, L. Zhai, B. Yin, F. Zhou, Y. Geng, J. Pan, J. Luo, B. Gu and H. Liu, Ammonia emissions from paddy fields are underestimated in China, Environ. Pollut., 2018, 235, 482–488, DOI:10.1016/j.envpol.2017.12.103
.
- P. Rochette, D. A. Angers, M. H. Chantigny, M. O. Gasser, J. D. MacDonald, D. E. Pelster and N. Bertrand, NH3 volatilization, soil NH4+ concentration and soil pH, Can. J. Soil Sci., 2013, 93, 261–268, DOI:10.4141/CJSS2012-095
.
- T. Liu, J. Huang, K. Chai, C. Cao and C. Li, Effect of N Fertilizer Sources and Tillage Practices on NH3 volatilization, Grain Yield and N Use Efficiency of Rice Fields in Central China, Front. Plant Sci., 2018, 9(385), 1–10, DOI:10.3389/fpls.2018.00385
.
- X. Liang, H. Zhang, M. He, J. Yuan, L. Xu and G. Tian, No-tillage effects on grain yield, N use efficiency and nutrient runoff losses in paddy fields, Environ. Sci. Pollut. Res., 2016, 23, 21451–21459, DOI:10.1007/s11356-016-7338-1
.
- J. S. Zhang, F. P. Zhang, J. H. Yang, J. P. Wang, M. L. Cai, C. F. Li and C. G. Cao, Emissions of N2O and NH3, and nitrogen leaching from direct seeded rice under different tillage practices in central China, Agric., Ecosyst. Environ., 2011, 140, 164–173, DOI:10.1016/j.agee.2010.11.023
.
- M. M. R. Jahangir, R. Begum, M. Jahiruddin, K. Dawar, M. Zaman, R. W. Bell, K. G. Richards and C. Müller, Reduced Tillage with Residue Retention and Nitrogen Application Rate Increase N2O Fluxes from Irrigated Wheat in a Subtropical Floodplain Soil, Agric., Ecosyst. Environ., 2020, 306, 107194, DOI:10.1016/j.agee.2020.107194
.
- Q. Y. Shang, C. M. Gao, X. X. Yang, P. P. Wu, N. Ling, Q. R. Shen and S. Guo, Ammonia volatilization in Chinese double rice-cropping systems: a 3-year field measurement in long-term fertilizer experiments, Biol. Fertil. Soils, 2014, 50, 715–725, DOI:10.1007/s00374-013-0891-6
.
- M. K. Alam, R. W. Bell, M. E. Haque and M. A. Kader, Minimal soil disturbance and increased residue retention increase soil carbon in rice-based cropping system on the Eastern Gangetic Plains, Soil Tillage Res., 2018, 183, 28–41, DOI:10.1016/j.still.2018.05.009
.
- Y. S. Bijay-Singh, J. K. Ladha, C. S. Khind, T. S. Khera and C. S. Bueno, Effects od residue decomposition on productivity and soil fertility in rice-wheat rotation, Soil Sci. Soc. Am. J., 2004, 68, 854–864, DOI:10.2136/sssaj2004.8540
.
- R. Gupta, J. Ladha, J. Singh, G. Singh and H. Pathak, Yield and phosphorus transformations in a rice-wheat system with crop residue and phosphorus management, Soil Sci. Soc. Am. J., 2007, 71, 1500–1507, DOI:10.2136/sssaj2006.0325
.
- T. Guo, Q. Zhang, C. Ai, G. Liang, P. He and W. Zhou, Nitrogen enrichment regulates straw decomposition and its associated microbial community in a double-rice cropping systems, Nature, 2018, 8, 1847, DOI:10.1038/s41598-018-20293-5
.
- C. Yan, S. S. Yan, T. Y. Jia, S. K. Dong, C. M. Ma and Z. P. Gong, Decomposition characteristics of rice straw returned to the soil in northeast China, Nutr. Cycling Agroecosyst., 2019, 114, 211–224, DOI:10.1007/s10705-019-09999-8
.
- T. Q. Liu, D. J. Fan, X. X. Zhang, J. Chen, C. F. Li and C. G. Cao, Deep placement of nitrogen fertilizers reduces ammonia volatilization and increases nitrogen utilization efficiency in no-tillage paddy fields in central China, Field Crops Res., 2015, 184, 80–90, DOI:10.1016/j.fcr.2015.09.011
.
- S. G. Sommer, J. K. Schjoerring and O. T. Denmead, Ammonia emission from mineral fertilizers and fertilized crops, Adv. Agron., 2004, 82, 557–622, DOI:10.1016/S0065-2113(03)82008-4
.
- T. W. Andraski, L. G. Bundy and K. R. Brye, Corn management and nitrogen rate effects on nitrate leaching, J. Environ. Qual., 2000, 29, 1095–1103, DOI:10.2134/jeq2000.0047242
.
- S. Peng, R. J. Buresh, J. Huang, J. Yang, Y. Zou, X. Zhong, G. Wang and F. Zhang, Strategies for overcoming low agronomic nitrogen use efficiency in irrigated rice system in China, Field Crops Res., 2006, 96, 37–47, DOI:10.1016/j.fcr.2005.05.004
.
- S. Peng, R. J. Buresh, J. Huang, X. Zhong, Y. Zou, J. Yang, G. Wang, Y. Liu, R. Hu, Q. Tang, K. Cui, F. Zhang and A. Dobermann, Improving nitrogen fertilization in rice by site-specific N management, A review, Agron. Sustainable Dev., 2010, 30, 649–656, DOI:10.1051/agro/2010002
.
- J. H. Guo, X. J. Liu, Y. Zhang, J. L. Shen, W. X. Han, W. F. Zhang, P. Christie, K. W. T. Goulding, P. M. Vitousek and F. S. Zhang, Significant acidification in major chinese croplands, Science, 2010, 327, 1008–1010, DOI:10.1126/science.1182570
.
- J. B. Garden and L. E. Drinkwater, The fate of nitrogen in grain cropping systems: a meta-analysis for 15N field experiments, Ecol. Appl., 2009, 19, 2167–2184, DOI:10.1890/08-1122.1
.
-
IPCC, 2019 Refinement to the 2006 IPCC Guidelines for National Greenhouse Gas Inventories, 2019, vol. 4, p. 11 Search PubMed
.
- L. Xia, Y. Xia, S. Ma, J. Wang, S. Wang, W. Zhou and X. Yan, Greenhouse gas emissions and reactive nitrogen releases from rice production with simultaneous incorporation of wheat straw and nitrogen fertilizer, Biogeosciences, 2016, 13, 4569–4579, DOI:10.5194/bg-13-4569-2016
.
- X. Chen, Z. Cui, M. Fan, P. Vitousek, M. Zhao, W. Ma, Z. Wang, W. Zhang, X. Yan, J. Yang, X. Deng, Q. Gao, Q. Zhang, S. Guo, J. Ren, S. Li, Y. Ye, Z. Wang, J. Huang, Q. Tang, Y. Sun, X. Peng, J. Zhanng, M. He, Y. Zhu, J. Xue, G. Wang, L. Wu, N. An, L. Wu, L. Ma, W. Zhang and F. Zhang, Producing more grain with lower environmental costs, Nature, 2014, 514, 486–489, DOI:10.1038/nature13609
.
- R. Roscoe, A. E. Furtini-Neto, G. A. A. Guedes and L. A. Fernandes, Urease activity and its relation to soil organic matter, microbial biomass nitrogen and urea-nitrogen assimilation by maize in a Brazilian Oxisol under no-tillage and tillage systems, Biol. Fertil. Soils, 2000, 32, 52–59 CrossRef CAS
.
- M. M. Hashim, M. K. Yusop, R. Othman and A. W. Wahid, Characterization of Nitrogen Uptake Pattern in Malaysian Rice MR219 at Different Growth Stages Using 15N Isotope, Ric. Sci., 2015, 22(5), 250–254, DOI:10.1016/S1672-6308(14)60305-X
.
- H. K. Kludze, R. D. DeLaune and W. H. Patrick, Aerenchyma formation and methane and oxygen exchange in rice, Soil Sci. Soc. Am. J., 1993, 57, 386–391, DOI:10.2136/sssaj1993.03615995005700020017x
.
- Y. L. Li, X. R. Fan and Q. R. Shen, The relationship between rhizosphere nitrification and nitrogen-use efficiency in rice plants, Plant, Cell Environ., 2008, 31, 73–85, DOI:10.1111/j.1365-3040.2007.01737.x
.
Footnote |
† Electronic supplementary information (ESI) available. See DOI: 10.1039/d0em00374c |
|
This journal is © The Royal Society of Chemistry 2021 |
Click here to see how this site uses Cookies. View our privacy policy here.