Advances in research on petroleum biodegradability in soil
Received
25th August 2020
, Accepted 25th November 2020
First published on 28th November 2020
Abstract
With the increased demand for petroleum and petroleum products from all parts of the society, environmental pollution caused by petroleum development and production processes is becoming increasingly serious. Soil pollution caused by petroleum seriously affects environmental quality in addition to human lives and productivity. At present, petroleum in soil is mainly degraded by biological methods. In their natural state, native bacteria in the soil spontaneously degrade petroleum pollutants that enter the soil; however, when the pollution levels increase, the degradation rates decrease, and it is necessary to add nutrients, dissolved oxygen, biosurfactants and other additives to improve the degradation ability of the native bacteria in the soil. The degradation process can also be enhanced by adding exogenous petroleum-degrading bacteria, microbial immobilization technologies, and microbial fuel cell technologies.
Environmental significance
Much research has been performed on the environmental behavior of oil-contaminated soil remediation by biological methods. However, objects, methods and purposes of early research are fairly diverse. In this paper, the development and research status of biodegradation of petroleum were reviewed, and the literature was studied from three aspects: nutrients, oxygen and surfactants can promote the growth of petroleum-degrading bacteria; immobilized exogenous petroleum degrade bacteria to promote petroleum degradation; and soil microbial fuel cell technology was used to degrade petroleum. The conclusions obtained were summarized, and some suggestions for future research were proposed. The information provided in this review is considerably significant to improve the understanding of the environmental behavior of petroleum in soil environments.
|
1. Introduction
With rapid economic development, the demand for petroleum is continually increasing. During the exploitation, transportation, production and processing, oil spills have caused heavy pollution of soil surroundings; the phenomenon of oil pollution has not just occurred in recent years.
As early as 1980s, there were serious oil spills in the United States. In 1984, the National Underground Oil Storage Tank Plan was implemented; however, currently, 20% of these underground oil tanks still leak.1,2 On March 24, 1989, the Exxon Valdez ran aground on Blei Reef, north of Prince William Sound, which led to the death of a large number of Marine lives, and caused serious damage to the nearby aquaculture industry.3,4 On April 20, 2010, the explosion and sinking of the Deepwater Horizon, an offshore oil rig about 80 kilometers off the coast of Louisiana, caused a daily influx of oil into the Gulf of Mexico.5,6 Russia is a major world oil producer. About 60
000 oil spills occur every year, and nearly 1/8 of soil has been damaged by pollution. The pollution levels of soil near oil banks in northern Mexico are 10 times higher than that of the local standard. There is serious oil pollution around nearly all refineries in UK.7–9
At present, there are more than 400 explored and developed oil and gas fields in China; these fields cover an area of 320
000 km2, in which approximately 4.8 million hm2 of soil has been polluted to varying degrees.10 Oil pollution of soils has become a widespread and worldwide concern. In some countries and regions, pollution levels are quite serious. Currently, oil pollution has not been controlled, and countries worldwide are paying great attention to it.
Petroleum is composed of a mixture of many different substances, of which alkanes, naphthenes and aromatic hydrocarbons are the main focus of attention. After oil is discharged into the soil, the soil ecosystem can degrade oil due to the self-purification capacity of the soil. However, if a large amount of oil enters the soil that is beyond the self-purification capacity over a short period, it will cause soil pollution.11 Soil pollution caused by petroleum is not only due to its high toxicity but can also change the physical and chemical properties of soil and damage the structures of soil biomes and living environment. After entering the soil, petroleum substances infiltrate and erode the soil layer and cause soil salinization, while polluted soils are also prone to compaction.12 The pH of soils contaminated by petroleum decreases and the ratios of soil C and N are out of balance, which affects the chemical properties of the soil enzymes.13,14 Long-term accumulation of oil substances damages soil structures and affects soil permeabilities. Oil adheres to the root surfaces of plants, forms mucous membranes and damages plant roots, which harm both plants and microbial ecosystems and seriously affect soil productivity and crop yields.15 The amount of residual oil in rice produced in the Shenfu Sewage Tank area exceeded the standard and caused an unpleasant smell. The nutritional composition of the rice changed, which seriously affected its yield and quality.16 Polycyclic aromatic hydrocarbons in petroleum are difficult to degrade and can accumulate in soil for long periods; they eventually enter the human body through the food chain. They have strong mutagenic, carcinogenic and teratogenic effects and threaten human lives and health.17 It is clear that petroleum pollution poses a serious threat to the environment and human health. As petroleum commonly contains hazardous chemicals such as benzene, ethylbenzene, toluene, and xylenes (BETX), it threatens the health of humans and the safety of the soil ecological system.18–20 The molecular structures of benzene, toluene, ethylbenzene, and xylene, commonly known as BTEX materials, and their physicochemical properties are listed in Table 1.
Table 1 Compositions of petrochemical hydrocarbon pollutants (BTEX)21
|
Benzene |
Toluene |
m-Xylene |
o-Xylene |
p-Xylene |
Ethylbenzene |
Chemical structure |
|
|
|
|
|
|
Formula |
C6H6 |
C7H8 |
C8H10 |
C8H10 |
C8H10 |
C8H10 |
Molecular weight (g mol−1) |
78 |
92 |
106 |
106 |
106 |
106 |
Solubility in water (mg L−1) |
1700 |
515 |
— |
175 |
198 |
152 |
Steam pressure (mm Hg) at 20 °C |
95.2 |
28.4 |
— |
6.6 |
— |
9.5 |
Special density (at 20 °C) |
0.8787 |
0.8669 |
0.8642 |
0.8802 |
0.8610 |
0.8670 |
Octane coefficient (at 20 °C) |
2.13 |
2.69 |
3.15 |
3.15 |
2.77 |
3.20 |
Fixed art law (in 25 °C) (kPam3 mol−1) |
0.55 |
0.67 |
0.7 |
0.5 |
0.71 |
0.8 |
Maximum amount of contaminants (MCL) (mg L−1) |
0.005 |
1 |
10 |
10 |
10 |
0.7 |
Aromatic hydrocarbons (aromatics) are compounds that contain only carbon and hydrogen in their structures. Aromatic hydrocarbons can also cause bone marrow suppression and musculoskeletal malformations.22 This group of compounds contains benzene and its derivatives as well as several condensed benzene rings. When hydrogen atoms in the benzene rings are replaced by methyl or ethyl, are new compounds are obtained, such as toluene (methylbenzene), xylene (dimethyl benzene), and ethylbenzene. In the case of xylene, there are three types of topical isomers, called o-, m-, and p-xylene.23 The aromatic hydrocarbons have molecular rings. Especially, the difficult-to-degrade components in oil (PAHs-polycyclic aromatic hydrocarbons) will continue to cause damage to the soil environment. They have varying physicochemical and toxicological characteristics according to their molecular weights (Table 2). PAHs are composed of fused benzene rings consisting of carbon and hydrogen arranged in a simple to complex structural configuration, i.e., in linear, angular, or cluster arrangements. PAHs are classified according to their aromatic benzene ring number, i.e., those consisting of two or three benzene rings are classified as low molecular weight (LMW) PAHs, such as naphthalene, anthracene, and phenanthrene, and PAHs consisting of more than three benzene rings are classified as high molecular weight (HMW) PAHs (pyrene, chrysene, benzo(a)pyrene, coronene, etc.). Vieira et al.25 identified five hydrocarbons by chromatographic analysis: paraffins, isoparaffins, olefins, naphthenics, and aromatics. Naphthenes do not degrade as readily as other hydrocarbons that have been identified. A study by Mohammadi et al.21 showed that benzene can stick to soil particles and that BTEX compounds can be broken down slowly in the presence of sufficient oxygen. Also, they are very volatile and affect the human respiratory system. Sakshi et al.24 found that the hard-to-degrade components of petroleum (PAHs) continue to cause damage to the soil environment. Therefore, treatment and repair of petroleum pollution has become an urgent environmental concern.
Table 2 Physicochemical properties of major PAHs24
S. no. |
Name |
Mol. formula |
Chemical structure |
1 |
Naphthalene |
C10H8 |
|
2 |
Fluorene |
C13H10 |
|
3 |
Anthracene |
C14H10 |
|
4 |
Phenanthrene |
C14H10 |
|
5 |
Fluoranthene |
C16H10 |
|
6 |
Pyrene |
C16H10 |
|
7 |
Benzo[a]anthracene |
C18H12 |
|
8 |
Benzo[a]pyrene |
C20H12 |
|
9 |
Benzo[ghi]perylene |
C22H12 |
|
Over the years, many scholars have devoted much effort to researching and developing oil pollution remediation technologies. At present, there is relatively little systematic research on this topic, and the main remediation methods include physical, chemical and biological remediation.26,27 Before the 1980s, physical and chemical methods were the main methods for controlling oil pollution. Traditional physical methods include incineration, isolation and alien soils. Most of these methods change the form of the pollutants or isolate them from soil; however, they cannot fundamentally resolve the actual pollution. The costs are high and the project volumes are large.
After many years of improvement and development, thermal desorption, electrical repair and other new technologies have been invented, such as the thermal desorption method and the electrical repair method. However, the thermal desorption method requires more equipment and large amounts of energy consumption. The electrical repair method requires rigorous reaction conditions. These disadvantages restrict their application.28,29
Compared with physical methods, chemical remediation is usually simple, efficient and mature. Commonly used chemical methods include chemical leaching, chemical oxidation and cement curing. Although these methods can achieve good oil removal effects, the introduction of chemicals (permanganate, persulfate, titanium dioxide, acetone)30 causes secondary pollution, which increases the complexity and cost of restoration.31–33 Due to the limitations of physical and chemical approaches, bioremediation technology has been widely studied by scholars since the 1970s.34–40
Biological methods apply inoculated microorganisms and indigenous microorganisms to degrade petroleum. After microbial activity assimilation, metabolism and cell decomposition, petroleum is converted into carbon dioxide and water, which are harmless for soil.41,42 This process does not introduce foreign substances and enriches biological populations in soil. Furthermore, the biological methods promote material circulation and have low treatment costs and high efficiency. Domestic and foreign scholars have adopted biological methods to repair oil-contaminated soil and have achieved promising results.43–45
On March 24, in 1989, the famous Exxon Valdez spill occurred, which is one of the worst petroleum-caused environmental disasters in history. Dispersants, controlled burns, skimming, siphoning from the wellhead, containment booms, shoreline scavenging/berms, and beach sand mixing were used extensively to mitigate the impact of the spill. Although numerous physical means were used to remove or disperse the oil, ultimately it was microbes that played the major role in mitigating the environmental impacts of the two worst oil spills in U.S. history.46
During the Exxon Valdez spill, water washing and bioremediation (biostimulation using fertilizers containing N nutrients) were the major strategies. This involved the application of nitrogen-containing fertilizers to stimulate growth of indigenous hydrocarbon-degrading microorganisms within the intertidal zones; shorelines were treated with Inipol EAP 22 (7.4% N, 0.7% P), an oleophilic liquid fertilizer, and Customblen (28% N, 3.5% P), a slow-release granulated fertilizer. Treated areas were significantly cleaner than adjacent untreated areas within a few weeks of fertilizer application.4,47–51 The efficiency of biosurfactants in remediation of petroleum hydrocarbon pollution has been used in the removal of Exxon Valdez oil from gravel.52 Bioremediation was used extensively after the Exxon Valdez spill. At present, biological remediation of oil pollution has become one of the most dynamic treatment technologies.53–55
This paper describes the treatment methods for petroleum pollution, its development history, and the research status of biological methods. The environmental conditions of microbial degradation are explored. This study can provide assistance for screening and optimizing microbial degradation agents and making better use of biological methods to degrade petroleum in soil.
2. Characteristics of petroleum-degrading bacteria in soils
Soil refers to loose mineral particles that are formed by various weathering processes. These weathering products of rocks are the foundation material for soil formation. The soil environment contains many kinds of native microorganisms, the number of which is as high as 4000–7000 species; this provides the possibility for the microbial degradation of petroleum.56,57
2.1 Types of soil microorganisms in the natural environment
As an important part of soil ecosystems, soil microorganisms are mainly involved in soil material and energy cycles. There are many kinds of soil microorganisms, which mainly include prokaryotes such as bacteria and actinomycetes and eukaryotes such as fungi, algae and protozoa. Bacteria, as the largest proportion of soil microorganisms, mainly use heterotrophic nutrition; they are distributed in surface soil and are the most active component of soil microorganisms. Actinomycetes are widely distributed in soils with high contents of alkaline and organic matter. Fungi are aerobic microorganisms that exist in the tillage layer. As opposed to bacteria and actinomycetes, fungi can grow and reproduce in acidic soil. Algae and protozoa are not widely distributed in soils. The growth of algae is greatly affected by light and water, and protozoa mainly feed on organic matter and can consume bacteria and algae.58
Soil microorganisms play an important role in the soil material cycle and can improve the soil environment through their metabolic activities. For example, bacteria produce extracellular polysaccharides to form complexes with soil particles, which can improve soil fertility.59 In addition, microbial activities are affected by the soil environment. Microorganisms are sensitive to environmental changes and can use their physiological and biochemical characteristics to reflect the environmental conditions of the soil and indicate changes in the soil environment.60
2.2 Types of soil petroleum-degrading bacteria
Under normal circumstances, the number of petroleum-degrading bacteria is relatively small in soils compared with petroleum-contaminated soil. When soils are polluted by petroleum, the numbers of petroleum-degrading bacteria show a rapid increase.34,61,62 Kostka et al.62 showed that the native microbial communities in beach sands respond fairly quickly to oil contamination, metabolizing oil to support growth at a rate which is within a factor of 2 to 4 in comparison to pure cultures. Vieira et al.25 simulated the continuous culture of degrading bacteria in the presence of petroleum pollutants in the laboratory. The results showed that a gradual increase in biomass concentration was verified at the end of each successive cultivation. The initial inoculum concentration was 0.3 ± 0.05 g L−1, and after the sixth cultivation (24 days) it was 3.5 ± 0.16 g L−1.
In the 1970s, people mainly focused on studies of microbial degradation mechanisms and metabolites. In the late 1980s, the United States successfully remediated petroleum contaminated soil in Alaskan waters for the first time by using petroleum-degrading bacteria in the soil.51 The community type of petroleum-degrading bacteria in the soil is greatly affected by the pollutant types and pollution degree. In oil-contaminated soils, bacteria and fungi form the dominant population and show sharp increases in their numbers. At the same time, the numbers of species declined, and the relative abundance increased. Moreover, the stability of microbial communities decreases with aggravation of pollution.63,64 The degradation efficiency of bacteria for petroleum is much higher than that of fungi and algae, and the number of bacteria is the largest.65
Petroleum-degrading bacteria have special cellular structures and usually contain more ribosomes in their cells. Petroleum can accumulate on the cytoplasmic membrane, and the ability of bacteria to synthesize phospholipids is strong.66 According to the statistics, more than 100 genera and more than 200 species of petroleum-degrading bacteria have been found. These mainly include bacteria, molds and actinomycetes.67,68 The classification of petroleum-degrading bacteria is shown in Table 3. However, not all species in these genera will be capable of hydrocarbon degradation. For example, some bacteria can metabolize specific alkanes, while others can decompose aromatic or hydrocarbon resins. Almost no bacteria can degrade the entire petroleum hydrocarbon fraction.71
Table 3 Genera of petroleum-degrading bacteria30,54,69,70
Classification |
Hydrocarbon degrading genera |
Bacteria |
Achromobacter, Acinetobacter, Alcaligenes, Arthrobacter, Actinomycetes, Bacillus, Brevibacterium, Brevibacillus, Citrobacter freundii, Chromobacterium, Corynebacterium, Enterobacter cloacae, Micrococcus, Mycobacterium, Micromonospora, Nocardia, Serratia marcescens, Sphingomonas, Staphylococcus, Stenotrophomonas, Streptomyces parvus, Sphingomonas, Spirillum, Ochrobactrum, Pseudomonas, Rhodococcus, Vibrio |
Fungus |
Trichoderma, Penicillium, Aspergillus, Mortierella, Rhodotorula, Selenotila, Trichosporon, Cladosporium, Cunninghamella, Fusarium, Gliocladium, Mucor, Verticillium, Phanerochaete |
Algae |
Anabaena, Oscillatoria, Nostoc |
2.3 Factors influencing the metabolism of petroleum-degrading bacteria in soils
Petroleum-degrading bacteria can effectively degrade petroleum in suitable environments and carry out effective bioremediation of contaminated soil. The metabolic activity of petroleum-degrading bacteria is not only affected by internal factors (microbial species and activity), but is also affected by many external factors (petroleum concentration, temperature, water, oxygen and nutrients). Therefore, it is significant to explore the suitable growth environment of petroleum-degrading bacteria.
Soil petroleum concentrations have a large influence on petroleum-degrading bacteria. High petroleum concentrations exert toxic effects on soil and petroleum-degrading bacteria, which significantly reduces the activity of petroleum-degrading bacteria and can even lead to their death.72 Hentati et al.73 found that as the oil concentration increased from 31 mg kg−1 to 1000 mg kg−1, the survival rate of earthworms decreased from 80% to 33% after 14 days. After oil enters the soil, if the concentration is not high, the self-purification ability of the soil can degrade the oil; however, if the concentration is too high, the oil will have toxic effects on the soil. In addition, the structures of petroleum molecules have a great influence on their biodegradability. In general, saturated hydrocarbons are most easily degraded under the same environmental conditions, followed by aromatic hydrocarbons; meanwhile, colloids and asphaltenes are the most difficult to biodegrade.74,75 As previously discussed, due to the complex physicochemical properties of petroleum hydrocarbons, lighter fractions volatilize readily following spill/release, fractions with hydrophilic and amphipathic natures dissolve in water, and lipophilic fractions are more likely to be bound to soil particles, organic matter, and stream sediment.76 Usually, the toxicity of the pH increases with increasing molecular weight. Similarly, cyclic alkanes with lower molecular weights are more toxic to aquatic organisms than aliphatic and aromatic compounds with similar molecular weights.77,78 In terrestrial environments, aromatic hydrocarbons have been proven to be more toxic than aliphatics.79 Additionally, it is established that in petroleum oil composition, in addition to polycyclic aromatic compounds, the lower aromatic compounds are also highly toxic.80 Lighter crudes, such as the oil released from the BP Deepwater Horizon spill, contain a higher proportion of simpler lower molecular weight hydrocarbons that are more readily biodegraded than heavy crudes, such as the oil released from the Exxon Valdez.46
The ambient temperature has a strong influence on the activity of soil petroleum-degrading bacteria. Normally, as the temperature rises, the growth and metabolism of bacteria accelerates. Different petroleum-degrading bacteria have different suitable temperatures. Generally, the degradation efficiency is higher when the temperature is between 30 °C and 40 °C. Low temperatures reduce the transport capacity of nutrients in soil and weaken the metabolic activity of petroleum-degrading bacteria.81–83
In addition, soil water can provide nutrient conditions for the metabolisms of petroleum-degrading bacteria because water dissolves organic matter with small molecules. Low content of water inhibits cell growth and metabolism.
Petroleum biodegradation is an oxidation process. The degradation rates of aerobic reactions are usually much higher than that of anaerobic reactions. Moreover, aerobic microorganisms can convert 20–40% of soil organic carbon into cellular materials, which is beneficial to improve soil organic quality.66 Therefore, petroleum-degrading bacteria need an aerobic environment.
As petroleum is mostly a hydrophobic substance, it forms oil films at interfaces, which prevents oxygen transfer and inhibits aerobic reactions. Therefore, tillage or proper use of leavening agents can increase soil porosity and oxygen supplementation. At the same time, growth and reproduction of petroleum-degrading bacteria require a variety of inorganic compounds and mineral elements. Generally, high content of carbon and low content of nitrogen and phosphorus leads to nutrient imbalance and affects the biosynthesis of petroleum-degrading bacteria. Therefore, appropriately increasing the nitrogen and phosphorus ratios in soil will promote the biodegradation of petroleum-degrading bacteria.84–86
2.4 The petroleum biodegradation process
The biodegradation process of petroleum includes adsorption, transfer and biological oxidation. First, microorganisms are adsorbed on the surface of petroleum pollutants. Then, petroleum is transferred to the cell membrane surfaces. Finally, petroleum provides metabolic substrates for microorganisms and is converted by bacteria. Additionally, intracellular biological oxidation can release energy.87
Petroleum-contaminated soil mainly uses the metabolism of aerobic bacteria to remove pollutants. The main principle of the microbial degradation of petroleum is shown in Fig. 1.
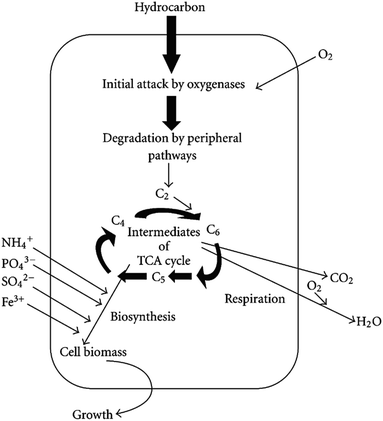 |
| Fig. 1 Main principle of aerobic degradation of hydrocarbons by microorganisms.88 | |
From Fig. 1, it can be seen that hydrocarbons undergo enzymatic reactions under the catalysis of oxygenase and catalase in the presence of oxygen. Hydrocarbons are converted in the tricarboxylic acid cycle through the peripheral degradation pathway. Finally, bacteria assimilate nutrients to synthesize new cells and exhibit growth and reproduction. In addition, hydrocarbons are oxidized to CO2 and H2O by respiration and generate energy.89
Hydrocarbons in petroleum can be divided into alkanes, cycloalkanes, aromatic hydrocarbons and unsaturated hydrocarbons according to their structures. In general, the biodegradation of alkanes occurs as a result of aerobic reactions.90 The biodegradation mechanism of alkanes is shown in Fig. 2.
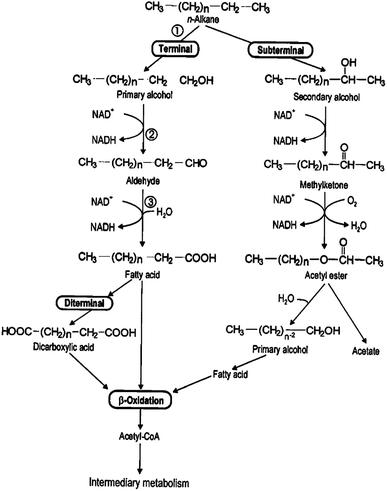 |
| Fig. 2 Biodegradation mechanism of alkane hydrocarbons.91 | |
As shown in Fig. 2, the initial degradation process occurs under aerobic conditions by adding an oxygen atom to the terminal or sub-terminal carbon. This process will convert aliphatic compounds to a few central intermediates, such as primary and secondary alcohol, through convergent pathways.92 The alcohols are then converted into aldehydes with the aid of alcohol dehydrogenase. The next reaction produces fatty acids from the reaction between the alcohol dehydrogenase enzyme and aldehydes. These fatty acids will react with coenzyme A (CoA) to produce acetyl CoA after undergoing the oxidation process. The whole process of alkane conversion is also called the carboxylation reaction. The degradation mechanism of cycloalkanes is generally considered to be a common metabolic action, as shown in Fig. 3.
 |
| Fig. 3 Cyclohexane biometabolic pathways. | |
Fig. 3 shows that the cycloalkanes are first converted into corresponding alcohols and ketones, and the latter are further oxidized to obtain esters or directly opened to generate corresponding fatty acids. The final products of microbial degradation are carbon dioxide and water.93–95Fig. 4 is an example of the biodegradation mechanism of aromatic hydrocarbons.
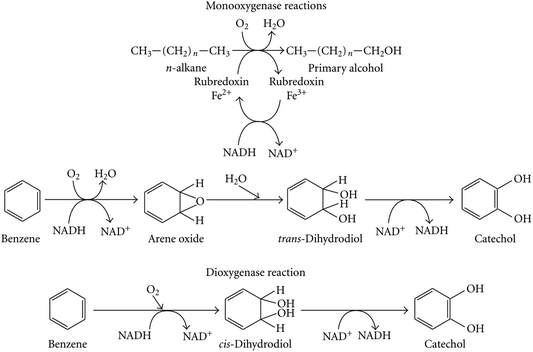 |
| Fig. 4 Enzymatic reactions involved in the processes of aromatic hydrocarbon degradation.88 | |
In the presence of dehydrogenase and redox enzymes, benzene and short-chain alkyl benzene are metabolized to catechol (see Fig. 4). Then, hydrogenation and dehydration effects cause the C–C key to break. Intermediate metabolites include dibasic alcohols, phenols, and epoxides. The end products are water and carbon dioxide, and the entire process depends on the activity of the microorganisms.94,95
At present, the degradation mechanism of alkynes and alkenes is not well understood. Some bacteria can metabolize them into unsaturated fatty acids and produce some double bond displacement or methylation. The formation of branched fatty acids is thought to be easily degraded by microorganisms.93
2.5 Effects of weathering on the biodegradation process
Petroleum hydrocarbons in the environment undergo weathering, which may involve physical (dispersion), physiochemical (evaporation, dissolution, sorption), chemical (photo-oxidation, auto-oxidation), and biological (plant and microbial catabolism of hydrocarbons) influences. Weathering may strongly impact the degradation of oil, and the degree and type of weathering will be different from site to site.96,97 Under the influence of weathering, the properties of petroleum, such as its adsorption/desorption, diffusion, distribution and chemical structures, will be changed. In addition, the water content and salinity in the soil will also be changed. Therefore, the microbial degradation of petroleum will be affected.98,99
For example, after six months of weathering, the light components of crude oil in the soil decreased seriously, and the heavy components changed little. Under the conditions of stronger light irradiation and higher temperature, the hydrocarbon in the oil can combine with the oxygen molecules to produce new oxygen-containing substances. With the process of weathering, the oil will diffuse and the local concentration of oil in the soil will decrease. These transformations require the species of flora to be changed accordingly. Moreover, weathering decreases the sizes of the soil particles, which can increase the contact area between the oil and soil and help the bacteria to degrade oil. In a word, many factors affect the efficiency of the biodegradation process during weathering.62,99
3. Strengthening effects of native petroleum-degrading bacteria
In the natural state, native soil microorganisms can degrade petroleum. However, their degradation ability is weak and the degradation period is generally lengthy. At the same time, with increased degrees of petroleum pollution, toxic effects on the degrading bacteria will occur and the biodegradation effect may even be lost. To overcome the problem of the low natural degradation efficiency of petroleum-degrading bacteria, artificial interventions are carried out for soil microbes, and measures such as addition of nutrients and biological surfactants and increasing the dissolved oxygen in the soil are taken to enhance biodegradation by the original petroleum-degrading bacteria.38,100
3.1 The strengthening effect of nutrients on petroleum-degrading bacteria
Growth of petroleum-degrading bacteria in soil is dependent on the nutrient supply, especially carbon, nitrogen and phosphorus, which play important roles in the remediation process. Petroleum-degrading bacteria need a suitable proportion of nutrients in a proper environment for normal growth. After petroleum enters the soil, the carbon concentrations greatly increase. The carbon source is mainly provided by petroleum, and the contents of nitrogen and phosphorus in soils cannot meet the demands of the degrading bacteria. A lack of soluble nitrogen and phosphorus becomes the limiting factor. Therefore, proper proportions of carbon, nitrogen and phosphorus can effectively promote petroleum degradation.101–103
Due to the different petroleum-degrading bacteria and different levels of soil pollution, the proportions of the necessary nutrients vary. Nikolopoulou et al.104 used uric acid and lecithin as nitrogen and phosphorus sources, respectively, and found that the enzyme activity improved when the ratio of nitrogen to phosphorus was 10
:
1. Płaza et al.105 pointed out that the mass ratio of carbon, nitrogen and phosphorus needed for petroleum-degrading bacterial growth is 120
:
10
:
1, which is in agreement with the study by Mills and Frankenberger.106 Many researchers found when the ratio of carbon, nitrogen and phosphorus in the soil was adjusted to 100
:
10
:
1, it reached the appropriate nutrient element ratio required.107–109
Some inorganic salts and fertilizers are often used as sources of N and P for microbial growth. Potassium sulphate, nitro phosphate, potassium hydrogen phosphate, potassium dihydrogen phosphate and nitrogen phosphorous inorganic salt can be used to adjust the proportions of nutrients in the soil. Furthermore, animal manure, crop straw, or sewage sludge can be used as substitutes for inorganic salts.110–113 Nutrients are gradually consumed in the process of petroleum degradation. Therefore, maintaining a continuous supply of nutrients in the appropriate proportions is also an important factor which affects the effective degradation of pollutants.114
3.2 The strengthening effect of dissolved oxygen on petroleum-degrading bacteria
In general, when molecular oxygen is used as an electron acceptor, it can provide large amounts of energy to microorganisms.115 Therefore, the activity of petroleum-degrading bacteria is affected not only by nutrient elements but also by dissolved soil oxygen. When soil is seriously polluted by petroleum, the oxygen demand rate for petroleum biodegradation increases. Therefore, increasing the oxygen supply rate can improve the petroleum bioremediation ability.
Godoy-Faúndez et al.116 continuously aerated polluted soil which contained 5% petroleum, and the petroleum degradation rate in the soil reached 35% after 56 days. Leahy and Colwell117 emphasized that oxygen is an important factor which can limit biodegradation in soil environments. Providing sufficient oxygen can improve the degradation efficiency of petroleum.
3.3 The strengthening effects of surfactants on petroleum-degrading bacteria
Petroleum exhibits strong hydrophobicity; therefore, it is difficult to dissolve in soil solutions and limits the metabolism of petroleum-degrading bacteria.118 Surfactants are amphiphilic compounds that contain hydrophilic and lipophilic groups, which can greatly reduce the interfacial tension between soil solutions and organic pollutants. Therefore, surfactants can promote the diffusion of organic pollutants from the soil interface to the aqueous phase. At the same time, surfactants can be used as cometabolism substrates.119–121 A schematic of the remediation of petroleum-contaminated soil by a surfactant is shown in Fig. 5.
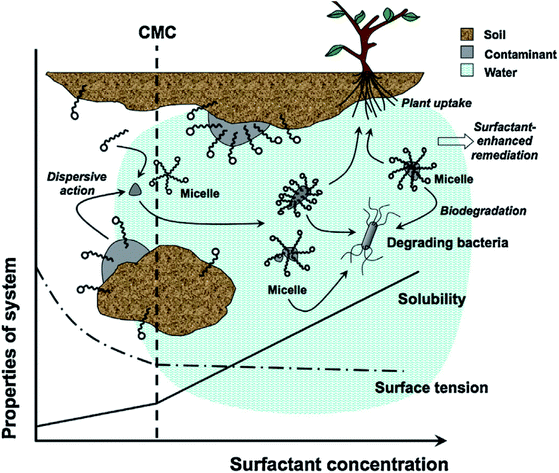 |
| Fig. 5 Schematic of surfactant enhanced-remediation of contaminated soils.122 | |
Fig. 5 shows that when the surfactant concentration exceeds the critical micelle concentration (CMC),123 elliptic and spherical micelles are formed to disperse pollutants to the aqueous phase. The petroleum is finally removed by petroleum-degrading bacteria.122
At present, the commonly used synthetic surfactants in petroleum-contaminated soils are fatty alcohol polyoxyethylene ether, dodecyl polyglycol ether, branched chain polyoxyethylene, SDS (sodium dodecyl sulfate), and SDBS (sodium dodecyl benzene sulfonate). Edwards et al.124 found that the use of synthetic surfactants can cause PAHs to be more soluble and has no negative effects on bacteria. Moldes et al.125 compared bioremediation effects under different conditions and found that surfactants significantly increased the petroleum degradation rate of bacteria.
In contrast, due to the toxicity of surfactants, when their concentrations reach a certain level, they will exert an inhibitory effect on petroleum-degrading bacteria. Compared with chemical surfactants, biosurfactants have the advantages of low biotoxicity, biodegradability and better adaptability to extreme environments. Kaczorek et al.126 investigated the efficiency of three surfactants (e.g., rhamnose-lipid, Triton X-100 and saponins) in degrading diesel oil. Compared with the other two surfactants, rhamnose-lipid significantly improved the degradation efficiency, and 88% of the diesel oil was biodegraded after 14 days.
Although biosurfactants have many advantages over chemical surfactants, their high production costs limit their large-scale use. In the process of soil remediation, if petroleum-degrading bacteria can not only degrade petroleum pollutants but can also produce surfactants themselves, this activity will effectively promote efficient degradation of petroleum hydrocarbons. Bao et al.127 isolated the petroleum-degrading bacteria D3-2, which can produce biosurfactants from contaminated soil. This bacterial strain significantly reduced the surface tension of the system, and the degradation rate of petroleum reached 82%.
4. Strengthening effect of exogenous petroleum-degrading bacteria
With increasing levels of soil petroleum pollution, soil pollutant types increase daily; meanwhile, the structures of petroleum compounds continue to become more complex and diverse, which leads to deterioration of the soil environment. To further improve the degradation ability of petroleum-degrading bacteria and achieve soil remediation effects more rapidly and effectively, human intervention and reinforcement are commonly used methods in the biodegradation process. The introduction of exogenous petroleum-degrading bacteria and a variety of degrading bacteria mixed cultures, microbial immobilization technologies and other methods that enhance the degradation process are used to improve the environmental quality of soil.
4.1 Adding exogenous petroleum-degrading bacteria to enhance petroleum degradation
4.1.1 Strengthening effects of a single exogenous petroleum-degrading bacterial type.
When soils are seriously petroleum-polluted, native microorganisms in the soils show low degradation efficiency for petroleum and are even unable to degrade certain petroleum types. Therefore, exogenous, efficient petroleum-degrading bacteria are introduced to improve the degradation efficiency for petroleum. At present, the main research objects are fungi and bacteria. By separating, cultivating and screening high-efficiency petroleum-degrading strains, they can be modified into dry bacteria and preserved for use. In addition, genetic engineering methods are used to induce mutations in existing petroleum-degrading bacteria and obtain efficient strains for soil pollution remediation.128,129
Mancera-López et al.36 separated rhizopus, fungi and earthen mold from petroleum-contaminated soil, and the petroleum removal rate increased by 16% after culture and domestication of these organisms when compared with native degradation bacteria. Mishra et al.130 repaired soil at a refinery and found a degradation rate of 89.7% of the petroleum in the degraded soil that was inoculated with exogenous bacteria, while the degradation rate of petroleum in the control group without exogenous bacteria was only 14%. Therefore, introducing exogenous petroleum-degrading bacteria into contaminated soil can significantly enhance the metabolism of native petroleum-degrading bacteria and improve the degradation efficiency of soil petroleum. In addition, the compatibility between the exogenous bacteria and native degradation bacteria, reproductive ability of the exogenous bacteria, environmental adaptability and genetic stability of the exogenous bacteria should be considered before introduction of exogenous bacteria so that they are able to work synergistically.
4.1.2 Strengthening effects of mixed exogenous petroleum-degrading bacteria.
Because petroleum consists of a variety of compounds, there are many kinds of pollutants and complex structures in contaminated soil, which also causes the problem of unstable degradation efficiency by a single bacterial strain/species. Multiple strains are usually separated and screened from the contaminated soil, and the combined action of mixed bacteria is used to degrade soil petroleum. There are two main situations in mixed cultures of multiple strains. One is that each strain can degrade pollutants when acting alone, but the degradation efficiency is higher after mixing, which generally occurs when phenol and alkyl benzene sulfonate are degraded. The other is that each strain cannot degrade the pollutant when it exists alone. After a mixed culture is created, it can promote pollutant degradation synergistically.131 In either case, the mixed culture method is beneficial for improving the degradation efficiency of petroleum and provides new ideas for bioremediation processes of petroleum-contaminated soil.
When a variety of strains are mixed in a culture, the metabolites of one strain may be the substrate of the growth of another strain, and their interspecific interactions play a positive role in their metabolism. Compared with single biodegradable bacteria, biodegradable bacteria have the advantages of stable biological communities, high microbial activity, high degradation efficiency and strong adaptability to environmental changes.132,133 Cerqueira et al.134 studied the biodegradability of aliphatic and aromatic hydrocarbons in oily sludges; they found that mixed degrading bacteria demonstrated better degradability when treating pollutants, reducing the aliphatic fraction by 90.7% and aromatic fraction by 51.8%. Rahman et al.135 selected five biodegradable bacteria for mixed cultures and compared them with single bacteria; they found that in petroleum-contaminated soil with a concentration of 1%, mixed bacteria showed the highest degradation efficiency, up to 78%, while single bacteria showed the highest degradation rate of 66%. Chen et al.136 isolated Crotella NW03 and Bacillus subtilis NW08 from petroleum cracking bacteria in Changqing, Shaanxi Province, and proposed a new method for the combined remediation of oil-polluted soil by fungi and bacteria. The removal of total petroleum hydrocarbon (TPH) by the mixture was higher than the sum of the individual removal obtained with the pure cultures. Yang et al.137 isolated four strains of bacteria from the oil-polluted soil of The Leng Hu Oil field, Qilian Mountain and Qinghai-Tibet Plateau, respectively, in Qaidam Basin. The results showed that the mixed culture had a stronger ability to degrade hydrocarbons than pure culture, and the crude oil degradation rate could reach almost 100% when two strains were present. Russel et al.138 isolated three strains from Panjin Liaohe Oilfield, and the results showed that the biodegradability of the single strains was weaker than that of the mixed strain. Pristane, which is the most difficult alkane to degrade, is easily degraded by mixed strains.
4.2 Immobilized exogenous microbes enhance petroleum degradation
At the present stage, petroleum-degrading bacteria are used for soil remediation and, regardless of whether native degradation bacteria or introduced high-efficiency exogenous bacteria are used, all are free bacteria. In the actual degradation process, the concentration of degrading bacteria per unit volume is low, and the bacteria are not able to exert continuous action on petroleum pollutants.139 Microbial immobilization technology is a method of using physical or chemical methods to locate free microorganisms in an area with limited space to increase the concentration of microbial cells and to maintain them at a high level biological activity and enable them to be repeatedly reused. With the advantages of high concentrations of biological cells, stable environment and resistance to changes in adverse conditions, low costs of energy consumption and simple operation, this technology has been used in recent years to repair soil contaminated with oil.140,141
4.2.1 Microbial immobilization method and immobilized carriers.
Microbial immobilization methods can be divided into five types: adsorption, surface binding (electrostatic or covalent), flocculation (natural or artificial), entrapment and encapsulation, as shown in Fig. 6.
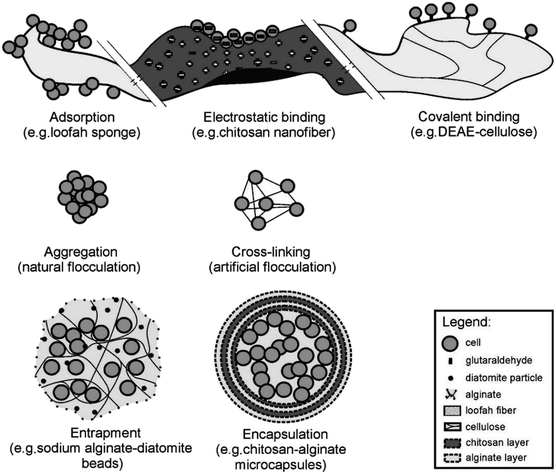 |
| Fig. 6 Schematic of microbial immobilization methods.142 | |
Adsorption methods have the advantages of being simple and rapid in environmental protection; however, they easily cause cell leakage. Covalent bonding surface binding methods are mostly used for enzyme immobilization because the binding agent required for immobilization is usually toxic to cells and will reduce their activity. Flocculation does not need a carrier and is often used in reactors. Entrapment is a fast, nontoxic and universal method which can prevent cell leakage. However, cells can only move inside the carrier after entrapment, which limits the exchange of nutrients and metabolites. Moreover, cell leakage will occur when the pores of the carrier are larger than the cell size. The encapsulation method is similar to the entrapment method. Its greatest advantage is that it can protect cells from interference by external adverse environments. However, due to limited membrane permeability and damage to cell growth, it is rarely used in in situ remediation.142–144
The immobilization methods required by the carrier are different, and a good carrier should have high microbial metabolic activity, not be easily metabolized by microorganisms, be nontoxic and harmless to cells and the environment, be easily obtainable, have low cost, exhibit structural stability, regenerate easily and have a long service life; thus, the research, development and choice of suitable carriers is an important part of the process of microbial immobilization.145
At present, the carriers used for microbial immobilization can be divided into organic carrier materials, inorganic carrier materials and natural carrier materials. Their types, advantages and disadvantages are shown in Table 4.149
Table 4 Types, advantages and disadvantages of microbial immobilized carriers146–148
Carrier type |
Representative substance |
Advantages and disadvantages |
Organic carrier material |
Polypropylene, polyvinyl chloride, polystyrene, polyacrylamide gel, etc. |
With many functional groups, high strength and good chemical stability, the porosity and pore size of the carrier can be controlled in the synthesis process. However, the mass transfer performance is poor and it is easy to cause cell inactivation |
Inorganic carrier material |
Activated carbon, porous glass, diatomite, magnetite, ceramics, etc. |
It has high physical, chemical and biological resistance, which is sensitive, harmless to cells, cheap and easy to make. However, it has few functional groups, which prevents the biocatalyst from fully binding and the microorganisms are easy to fall off |
Natural carrier material |
Bagasse, alginate, sawdust, straw, etc. |
It has many functional groups, and because it is mostly waste, it is relatively easy to obtain and low in cost. However, it has good biodegradability and biocompatibility, so it is easy to be decomposed and the scope of application is limited |
Xu and Lu150 used peanut shell powder as a microbial immobilized carrier to remediate soil that was contaminated by crude oil. After 12 weeks of treatment, the total removal rate of petroleum was between 26% and 61%. The use of this carrier improved the activity of soil dehydrogenase, and the degreasing effect was obviously enhanced. Liang et al.151 used zeolites and activated carbon as microbial immobilized carriers. After 33 days of treatment, the degradation rate of petroleum increased from 13.0% for the state of natural decay to 48.9%, which was higher than the degradation rate of 26.3% when supplementing with nitrogen and phosphorus.
4.2.2 Microbial immobilization technology enhances petroleum degradation.
Microbial immobilization technology can significantly improve and maintain the biological activity of petroleum-degrading bacteria, improve their ability to degrade pollutants, and effectively remove petroleum pollutants. The principle of remediating contaminated soil is shown in Fig. 7.
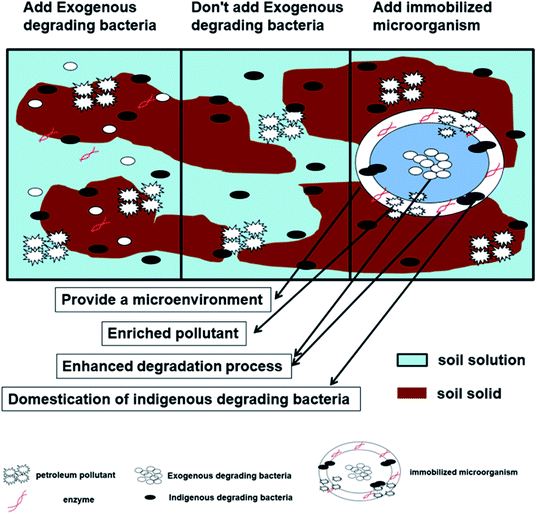 |
| Fig. 7 Repair principle of microbial immobilization technology. | |
The presence of an immobilized carrier provides a good attachment point for the degrading bacteria and the extracellular enzymes they secrete; this can increase the number of microorganisms in a unit environment, increase the contact area with pollutants and thus achieve the purpose of degrading petroleum pollutants. An immobilized carrier can provide a favorable environment for exogenous degradation bacteria, avoid competition between native degradation bacteria and exogenous degradation bacteria, prevent the soil environment from harming the bacteria, and ensure efficient growth of the added exogenous degradation bacteria. An immobilized carrier can enrich soil pollutants and thus become a favorable place for domestication of native degradation bacteria, which is conducive to joint remediation of contaminated soil by both native degradation bacteria and exogenous high-efficiency degradation bacteria. In addition, the immobilized material can loosen the soil, increase the oxygen content in pores, and accelerate mineralization of organic pollutants.152–154
Chen and Yuan155 evaluated the adsorption effects of soil modified with biochar as the immobilized carrier and found that the adsorption level of PAHs in soil increased significantly after biochar treatment. El-Naas et al.156 used polyvinyl alcohol (PVA) hydrogel microspheres as the immobilized material and explored different experimental conditions of immobilized Pseudomonas phenol degradation; the experimental results showed that at 30 °C, pH 7 and a phenol concentration of 75 mg L−1, the biodegradation effect was the best. Excessive phenol concentrations reduced the biodegradation rate. When the phenol concentrations were low, as the particle size of the polyvinyl alcohol particles decreased, the biodegradation rate increased.
5. Microbial fuel cell technology enhances petroleum degradation
Microbial fuel cell (MFC) technology is a sustainable environmental remediation technology which produces energy while degrading environmental pollutants. This energy source can not only feed the operation of its own system but can also provide excess energy output. The use of microbial fuel cells to repair environmental pollution has important research significance and has been widely used in treatments of wastewater, sediment, sludge and soil in recent years.157–159 Compared with aqueous phase microbial fuel cell remediation, soil microbial fuel cell repair is still lacking in its methods and technologies. As a new interdisciplinary field, soil bioelectrochemistry continues to receive increasing attention.
Noninvasive technology provides an ideal means for remediating contaminated soil. At present, microbial fuel cell technology is being widely used as an effective means of remediating environmental pollution. MFC is a device for directly converting chemical energy into electrical energy by degrading organic or inorganic matter through microbial metabolism. It has the advantages of no secondary pollution, high energy conversion rates, mild operating conditions and facile control.160 As highly effective catalysts, microorganisms can metabolize organic matter to produce electrons under anaerobic conditions. The electrons are transferred to the cathode through an external circuit to complete the current output process. The main structure of an MFC consists of an anaerobic anode, aerobic cathode and proton exchange membrane. The organic matter of the anode is oxidized by microorganisms to release electrons and protons. The electrons are transferred to the anode and the cathode through an external circuit. At the same time, the protons are transferred to the cathode through the proton exchange membrane and the electrons and protons on the cathode react with oxygen to form water, as shown in Fig. 8.162,163
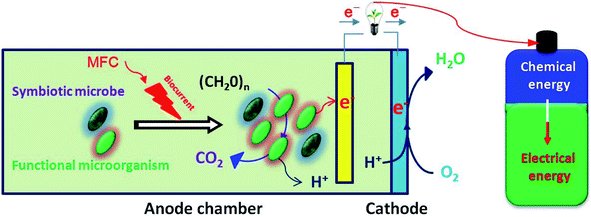 |
| Fig. 8 Schematic diagram of MFC remediation.161 | |
In addition to biodegradable organisms which can provide electrons, some inorganic salts such as S2− and Fe2+ can be used as electron donors and follow the same principle. Wang et al.164 discussed remediation of chromium-contaminated soil by use of microbial fuel cell technology. Experimental results have shown that MFC can be used as a potential and sustainable method for remediation of chromium-contaminated soil, and the remediation process does not require energy input. Habibul et al.165 studied metal removal efficiency and its influence on soil physiological characteristics for microbial fuel cell remediation of toxic metal-contaminated soil. After 108 and 143 days of operation, the removal rates of Cd and Pb in the anode area reached 31.0% and 44.1%, respectively. Soil pH values and electrical conductivities were significantly redistributed from the anode area to the cathode area.
In recent years, due to the problems of traditional soil petroleum bioremediation methods, such as low activity of functional microorganisms, lack of electron acceptors and low efficiency of electron transfer, MFC has been widely used to remediation of soil contaminated by petroleum. Using u-tube microbial fuel cells (MFCs), Wang et al.166 increased the degradation rate of petroleum hydrocarbons near the anode from 6.9 to 15.2, or 120%, during the 25 day test period. The research of Srikanth167 and Li et al.168 also showed that the use of microbial fuel cells to repair soil could significantly improve the total degradation rate of petroleum hydrocarbons. Zhao et al.169 studied the effects of plants, carbon sources and surfactants on the degradation of petroleum and polyaromatic hydrocarbons in soil and their mechanisms by using an enhanced plant microbial fuel cell (P-MFCS). The results showed that the synergistic effect of plants and surfactants significantly improved the efficiency of MFC systems in removing petroleum from soil.
6. Summary and prospects
Petroleum spills and spills during production and processing processes have caused serious impacts on the global environment and on human productivity and lives. When faced with the increasingly serious emergency of worldwide soil pollution, treatment and restoration of petroleum pollution is extremely urgent. At present, the main remediation methods include physical, chemical and biological methods, among which biological methods have become the main way of addressing petroleum pollution due to their advantages of environmental protection and high efficiency. Under normal circumstances, the number of petroleum-degrading bacteria in soil increases with increased soil pollution, and more than 200 kinds of petroleum-degrading bacteria have been found. The activities of degrading bacteria are affected not only by their own activities but also by soil environmental factors. Addition of nutrients, dissolved oxygen, biosurfactants and other additives to the soil can improve the degradation ability of degrading bacteria in the soil. The approaches of adding exogenous petroleum-degrading bacteria, microbial immobilization technology and microbial fuel cell technology can also be adopted to strengthen the degradation process. These measures will achieve better removal effects of petroleum pollution and restore the ecological environment of soil.
Some gains have been made in soil pollution remediation at home and abroad which provide theoretical guidance and technical support for the rational and efficient use of biological methods to remediate soil pollution. In the future, in-depth research on petroleum pollution remediation by biological methods should be conducted from the following perspectives:
(1) When adding nutrients, dissolved oxygen, biosurfactants and other additives to the soil to improve the degradation ability of the degrading bacteria, it is important to strive to find environmentally friendly additives and consider using a continuous supply of additives to maintain the efficient degradation ability of the degrading bacteria.
(2) Activities of petroleum-degrading bacteria are sensitive to environmental changes. For some soils in special environments, such as saline-alkali soils and frozen soils at low temperature, it is necessary to further select and cultivate high-efficiency degrading bacteria and explore their degradation mechanisms.
(3) When introducing exogenous petroleum-degrading bacteria, they often have antagonistic effects against native degradation bacteria which seriously affect pollutant removal. Therefore, it is necessary to select high-efficiency bacterial agents from genetic experimentation and further explore the synergistic and antagonistic mechanisms among all bacteria involved in the degradation process.
(4) In the process of soil remediation with high oil content, direct use of microbial remediation will lead to inactivation of degrading bacteria by highly toxic pollutants. At this time, physicochemical pretreatments should first be considered to better make use of biological remediation, while the impact of pretreatments on bioremediation needs further research.
Conflicts of interest
There are no conflicts to declare.
Acknowledgements
This study was funded by the National Natural Science Foundation of China (No. 5207040321), the Petrochina Innovation Foundation (No. 2016D-5007-0604), the Application Research and Development Program of Heilongjiang Province (No. GC13C305), and the Graduate Education Innovation Project of Northeast Petroleum University (No. JYCX_CX05-2018, No. JYCX_JG05-2018).
References
- M. E. McLearn, M. J. Miller, P. T. Kostecki, E. J. Calabrese, L. M. Preslo, W. Suyama and W. A. Kucharski, Remedial options for leaking underground storage tanks, JAPCA, 1988, 38, 428–435 CrossRef CAS.
- M. E. Zappi, B. A. Rogers, C. L. Teeter, D. Gunnison and R. Bajpai, Bioslurry treatment of a soil contaminated with low concentrations of total petroleum hydrocarbons, J. Hazard. Mater., 1996, 46, 1–12 CrossRef CAS.
- C. H. Peterson, Long-Term Ecosystem Response to the Exxon Valdez Oil Spill, Science, 2003, 302, 2082–2086 CrossRef CAS.
- S. Kirsch, Between the devil and the deep blue sea: objectivity and political responsibility in the litigation of the Exxon Valdez oil spill, Crit. Anthropol., 2020, 40, 403–419 CrossRef.
- R. Camill, C. M. Reddy, D. R. Yoerger, B. A. S. Van Mooy, M. V. Jakuba, J. C. Kinsey, C. P. McIntyre, S. P. Sylva and J. V. Maloney, Tracking Hydrocarbon Plume Transport and Biodegradation at Deepwater Horizon, Science, 2010, 330, 201–204 CrossRef.
- H. K. White, P. Y. Hsing, W. Cho, T. M. Shank, E. E. Cordes, A. M. Quattrini, R. K. Nelson, R. Camilli, A. W. J. Demopoulos, C. R. German, J. M. Brooks, H. H. Roberts, W. Shedd, C. M. Reddy and C. R. Fisher, Impact of the Deepwater Horizon oil spill on a deep-water coral community in the Gulf of Mexico, Proc. Natl. Acad. Sci. U. S. A., 2012, 109, 20303–20308 CrossRef CAS.
- I. Gildeeva, Environmental protection during exploration and exploitation of oil and gas fields, Environ. Geosci., 1999, 6, 153–154 CrossRef.
- R. Iturbe, C. Flores, R. M. Flores and L. G. Torres, Subsoil TPH and other petroleum fractions-contamination levels in an oil storage and distribution station in north-central Mexico, Chemosphere, 2005, 61, 1618–1631 CrossRef CAS.
- R. Iturbe, A. Castro, G. Perez, C. Flores and L. G. Torres, TPH and PAH concentrations in the subsoil of polyduct segments, oil pipeline pumping stations, and right-of-way pipelines from Central Mexico, Environ. Geol., 2008, 55, 1785–1795 CrossRef CAS.
- B. K. Gogoi, N. N. Dutta, P. Goswami and T. K. Mohan, A case study of bioremediation of petroleum-hydrocarbon contaminated soil at a crude oil spill site, Adv. Environ. Res., 2003, 7, 767–782 CrossRef CAS.
- W. B. McGill and M. J. Rowell, Determination of oil content of oil contaminated soil, Sci. Total Environ., 1980, 14, 245–253 CrossRef CAS.
- M. Tejada, M. T. Hernandez and C. Garcia, Soil restoration using composted plant residues: effects on soil properties, Soil Tillage Res., 2009, 102, 109–117 CrossRef.
- A. R. Autry and G. M. Ellis, Bioremediation: an effective remedial alternative for petroleum hydrocarbon-contaminated soil, Environ. Prog., 1992, 11, 318–323 CrossRef CAS.
- J. Haimi, Decomposer animals and bioremediation of soils, Environ. Pollut., 2000, 107, 233–238 CrossRef CAS.
- B. Dhal, H. N. Thatoi, N. N. Das and B. D. Pandey, Chemical and microbial remediation of hexavalent chromium from contaminated soil and mining/metallurgical solid waste: a review, J. Hazard. Mater., 2013, 250, 272–291 CrossRef.
- H. Li, Y. Zhang, C. G. Zhang and G. X. Chen, Effect of petroleum-containing wastewater irrigation on bacterial diversities and enzymatic activities in a paddy soil irrigation area, J. Environ. Qual., 2005, 34, 1073–1080 CrossRef CAS.
- F. Baud-Grasset, S. Baud-Grasset and S. I. Safferman, Evaluation of the bioremediation of a contaminated soil with phytotoxicity tests, Chemosphere, 1993, 26, 1365–1374 CrossRef CAS.
- D. Sarkar, M. Ferguson, R. Datta and S. Birnbaum, Bioremediation of petroleum hydrocarbons in contaminated soils: comparison of biosolids addition, carbon supplementation, and monitored natural attenuation, Environ. Pollut., 2005, 136, 187–195 CrossRef CAS.
- Y. P. Ting, H. L. Hu and H. M. Tan, Bioremediation of petroleum hydrocarbons in soil microcosms, Resour. Environ. Biotechnol., 1999, 2, 197–218 CAS.
- Q. Zhou, F. Sun and R. Liu, Joint chemical flushing of soils contaminated with petroleum hydrocarbons, Environ. Int., 2005, 31, 835–839 CrossRef.
- L. Mohammadi, A. Rahdar, E. Bazrafshan, H. Dahmardeh, M. Abu Bin Hasan Susan and G. Z. Kyzas, Petroleum Hydrocarbon Removal from Wastewaters: a Review, Processes, 2020, 8, 447 CrossRef CAS.
- J. G. Singh, I. Chang-Yen, V. A. Stoute and L. Chatergoon, Hydrocarbon levels in edible fish, crabs and mussels from the marine environment of Trinidad, Mar. Pollut. Bull., 1992, 24, 270–272 CrossRef CAS.
- H. Shim, E. Shin and S. T. Yang, A continuous fibrous-bed bioreactor for BTEX biodegradation by a co-culture of Pseudomonas putida and Pseudomonas fluorescens, Adv. Environ. Res., 2002, 7, 203–216 CrossRef CAS.
- S. K. Singh and A. K. Haritash, Polycyclic aromatic hydrocarbons: soil pollution and remediation, Int. J. Environ. Sci. Technol., 2019, 16, 6489–6512 CrossRef.
- P. A. Vieira, S. Faria, R. B. Vieira, F. P. França and V. L. Cardoso, Statistical analysis and optimization of nitrogen, phosphorus, and inoculum concentrations for the biodegradation of petroleum hydrocarbons by response surface methodology, J. Microbiol. Biotechnol., 2009, 25, 427–438 CrossRef CAS.
- N. Vasudevan and P. Rajaram, Bioremediation of oil sludge-contaminated soil, Environ. Int., 2001, 26, 409–411 CrossRef CAS.
- R. L. Rhykerd, B. Crews, K. J. McInnes and R. W. Weaver, Impact of bulking agents, forced aeration, and tillage on remediation of oil-contaminated soil, Bioresour. Technol., 1999, 67, 279–285 CrossRef CAS.
- M. Pazos, A. Plaza, M. Martín and M. C. Lobo, The impact of electrokinetic treatment on a loamy-sand soil properties, Chem. Eng. J., 2012, 183, 231–237 CrossRef CAS.
- P. P. Falciglia and F. G. A. Vagliasindi, Remediation of hydrocarbon polluted soils using 2.45 GHz frequency-heating P.P.: influence of operating power and soil texture on soil temperature profiles and contaminant removal kinetics, J. Geochem. Explor., 2015, 151, 66–73 CrossRef CAS.
- I. C. Ossai, A. Ahmed, A. Hassan and F. S. Hamid, Remediation of soil and water contaminated with petroleum hydrocarbon: a review, Environ. Technol. Innov., 2020, 17, 100526 CrossRef.
- J. Rivas, O. Gimeno, G. Ruth and F. J. BeltrÃn, Ozone treatment of PAH contaminated soils: operating variables effect, J. Hazard. Mater., 2009, 169, 509–515 CrossRef CAS.
- O. R. S. Da Rocha, R. F. Dantas, M. M. M. B. Duarte, M. M. L. Duarte and V. L. Da Silva, Oil sludge treatment by photocatalysis applying black and white light, Chem. Eng. J., 2010, 157, 80–85 CrossRef.
- M. Lu, Z. Zhang, W. Qiao, Y. Guan, M. Xiao and C. Peng, Removal of residual contaminants in petroleum-contaminated soil by Fenton-like oxidation, J. Hazard. Mater., 2010, 179, 604–611 CrossRef CAS.
- P. M. Rutherford, D. K. Banerjee, S. M. Luther, M. R. Gray, M. J. Dudas, W. B. McGill, M. A. Pickard and M. J. Salloum, Slurry-phase bioremediation of creosote and petroleum-contaminated soils, Environ. Technol., 1998, 19, 683–696 CrossRef CAS.
- R. Z. Hoff, Bioremediation: an overview of its development and use for oil spill cleanup, Mar. Pollut. Bull., 1993, 26, 476–481 CrossRef CAS.
- M. E. Mancera-López, F. Esparza-García, B. Chávez-Gómez, R. Rodríguez-Vázquez, G. Saucedo-Castaneda and J. Barrera-Cortés, Bioremediation of an aged hydrocarbon-contaminated soil by a combined system of biostimulation-bioaugmentation with filamentous fungi, Int. Biodeterior. Biodegrad., 2008, 61, 151–160 CrossRef.
- O. Potin, C. Rafin and E. Veignie, Bioremediation of an aged polycyclic aromatic hydrocarbons (PAHs)-contaminated soil by filamentous fungi isolated from the soil, Int. Biodeterior. Biodegrad., 2004, 54, 45–52 CrossRef CAS.
- M. Wu, W. A. Dick, W. Li, X. Wang, Q. Yang, T. Wang, L. Xu, M. Zhang and L. Chen, Bioaugmentation and biostimulation of hydrocarbon degradation and the microbial community in a petroleum-contaminated soil, Int. Biodeterior. Biodegrad., 2016, 107, 158–164 CrossRef CAS.
- M. W. Lim, E. V. Lau and P. E. Poh, A comprehensive guide of remediation technologies for oil contaminated soil – present works and future directions, Mar. Pollut. Bull., 2016, 109, 14–45 CrossRef CAS.
- S. Covino, A. D'Annibale, S. R. Stazi, T. Cajthami, M. Cvancarova, T. Stella and M. Petruccioli, Assessment of degradation potential of aliphatic hydrocarbons by autochthonous filamentous fungi from a historically polluted clay soil, Sci. Total Environ., 2015, 505, 545–554 CrossRef CAS.
- S. J. Varjani and V. N. Upasani, Carbon spectrum utilization by an indigenous strain of Pseudomonas aeruginosa NCIM 5514: Production, characterization and surface active properties of biosurfactant, Bioresour. Technol., 2016, 221, 510–516 CrossRef CAS.
- F. Abbasian, R. Lockington, M. Mallavarapu and R. Naidu, A comprehensive review of aliphatic hydrocarbon biodegradation by bacteria, Appl. Biochem. Biotechnol., 2015, 176, 670–699 CrossRef CAS.
- J. Ma, G. Yan, W. Ma, C. Cheng, Q. Wang and S. Guo, Isolation and characterization of oil-degrading microorganisms for bench-scale evaluations of autochthonous bioaugmentation for soil remediation, Water, Air, Soil Pollut., 2015, 226, 272 CrossRef.
- W. Liu, Y. Luo, Y. Teng, Z. Li and P. Christie, Prepared bed bioremediation of oily sludge in an oilfield in northern China, J. Hazard. Mater., 2009, 161, 479–484 CrossRef CAS.
- J. P. Salanitro, P. C. Johnson, G. E. Spinnler, P. M. Maner, H. L. Wisniewski and C. Bruce, Field-scale demonstration of enhanced MTBE bioremediation through aquifer bioaugmentation and oxygenation, Environ. Sci. Technol., 2000, 34, 4152–4162 CrossRef CAS.
- R. M. Atlas and T. C. Hazen, Oil biodegradation and bioremediation: a tale of the two worst spills in U.S. history, Environ. Sci. Technol., 2011, 45, 6709–6715 CrossRef CAS.
- J. R. Bragg, R. C. Prince, E. J. Harner and R. M. Atlas, Effectiveness of bioremediation for the Exxon Valdez oil spill, Nature, 1994, 368, 413–418 CrossRef CAS.
- E. Rosenberg, R. Legmann, A. Kushmaro, R. Taube, E. Adler and E. Z. Ron, Petroleum bioremediation-a multiphase problem, Biodegradation, 1992, 3, 337–350 CrossRef CAS.
- P. H. Pritchard, J. G. Mueller, J. C. Rogers, F. V. Kremer and J. A. Glaser, Oil spill bioremediation: experiences, lessons and results from the Exxon Valdez oil spill in Alaska, Biodegradation, 1992, 3, 315–335 CrossRef CAS.
- J. E. Lindstrom, R. C. Prince, J. C. Clark, M. J. Grossman, T. R. Yeager, J. F. Braddock and E. J. Brown, Microbial populations and hydrocarbon biodegradation potentials in fertilized shoreline sediments affected by the T/V Exxon Valdez oil spill, Appl. Environ. Microbiol., 1991, 57, 2514–2522 CrossRef CAS.
- P. H. Pritchard and C. F. Costa, EPA's Alaska oil spill bioremediation project. Part 5, Environ. Sci. Technol., 1991, 25, 372–379 CrossRef CAS.
- S. Harvey, I. Elashvili, J. J. Valdes, D. Kamely and A. M. Chakrabarty, Enhanced removal of Exxon Valdez spilled oil from Alaskan gravel by a microbial surfactant, Biotechnology, 1990, 8, 228–230 CAS.
- J. Harris, Soil microbial communities and restoration ecology: facilitators or followers?, Science, 2009, 325, 573–574 CrossRef CAS.
- S. Chandra, R. Sharma, K. Singh and A. Sharma, Application of bioremediation technology in the environment contaminated with petroleum hydrocarbon, Ann. Microbiol., 2013, 63, 417–431 CrossRef CAS.
- M. Potthoff, K. L. Steenwerth, L. E. Jackson, R. E. Drenovsky, K. M. Scow and R. G. Joergensen, Soil microbial community composition as affected by restoration practices in California grassland, Soil Biol. Biochem., 2006, 38, 1851–1860 CrossRef CAS.
- V. Torsvik, J. Goksøyr and F. L. Daae, High diversity in DNA of soil bacteria, Appl. Environ. Microbiol., 1990, 56, 782–787 CrossRef CAS.
- K. L. Steenwerth, L. E. Jackson, F. J. Calderón, M. R. Stromberg and K. M. Scow, Soil microbial community composition and land use history in cultivated and grassland ecosystems of coastal California, Soil Biol. Biochem., 2002, 34, 1599–1611 CrossRef CAS.
- M. Macková, T. Macek, P. Kucerová, L. Poláchová, J. Burkhard and J. Tríska, The beneficial effect of plants on detoxification of environmental pollutants, Biologia, 1999, 54, 76–77 Search PubMed.
- F. J. Calderón, L. E. Jackson, K. M. Scow and D. E. Rolston, Microbial responses to simulated tillage in cultivated and uncultivated soils, Soil Biol. Biochem., 2000, 32, 1547–1559 CrossRef.
- C. M. McCarthy and L. Murray, Viability and metabolic features of bacteria indigenous to a contaminated deep aquifer, Microb. Ecol., 1996, 32, 305–321 CrossRef CAS.
- V. Andreoni, L. Cavalca, M. A. Rao, G. Nocerino, S. Bernasconi, E. Dell'Amico, M. Colombo and L. Gianfreda, Bacterial communities and enzyme activities of PAHs polluted soils, Chemosphere, 2004, 57, 401–412 CrossRef CAS.
- J. E. Kostka, O. Prakash, W. A. Overholt, S. J. Green, G. Freyer, A. Canion, J. Delgardio, N. Norton, T. C. Hazen and M. Huettel, Hydrocarbon-degrading bacteria and the bacterial community response in gulf of Mexico beach sands impacted by the deepwater horizon oil spill, Appl. Environ. Microbiol., 2011, 77, 7962–7974 CrossRef CAS.
- P. Y. Cheung and B. K. Kinkle, Mycobacterium diversity and pyrene mineralization in petroleum-contaminated soils, Appl. Environ. Microbiol., 2001, 67, 2222–2229 CrossRef CAS.
- B. M. Coppotelli, A. Ibarrolaza, M. T. Del Panno and I. S. Morelli, Effects of the inoculant strain Sphingomonas paucimobilis 20006FA on soil bacterial community and biodegradation in phenanthrene-contaminated soil, Microb. Ecol., 2008, 55, 173–183 CrossRef CAS.
- S. Barathi and N. Vasudevan, Utilization of petroleum hydrocarbons by Pseudomonas fluorescens isolated from a petroleum-contaminated soil, Environ. Int., 2001, 26, 413–416 CrossRef CAS.
- R. Vanloocke, R. De Borger, J. P. Voets and W. Verstraete, Soil and groundwater contamination by oil spills; problems and remedies, Int. J. Environ. Stud., 1975, 8, 99–111 CrossRef CAS.
- R. J. Brooijmans, M. I. Pastink and R. J. Siezen, Hydrocarbon-degrading bacteria: the oil-spill clean-up crew, Microb. Biotechnol., 2009, 2, 587 CrossRef CAS.
- K. S. M. Rahman, T. J. Rahman, Y. Kourkoutas, I. Petsas, R. Marchant and I. M. Banat, Enhanced bioremediation of n-alkane in petroleum sludge using bacterial consortium amended with rhamnolipid and micronutrients, Bioresour. Technol., 2003, 90, 159–168 CrossRef CAS.
- X. Tang, L. Y. He, X. Q. Tao, Z. Dang, C. L. Guo, G. N. Lu and X. Y. Yi, Construction of an artificial microalgal-bacterial consortium that efficiently degrades crude oil, J. Hazard. Mater., 2010, 181, 1158–1162 CrossRef CAS.
- M. F. Imron, S. B. Kurniawan, N. I. Ismail and S. R. S. Abdullah, Future challenges in diesel biodegradation by bacteria isolates: a review, J. Cleaner Prod., 2020, 251, DOI:10.1016/j.jclepro.2019.119716.
- I. A. Hassan, E. E. Mohamedelhassan, E. K. Yanful, B. Weselowski and Z. C. Yuan, Isolation and characterization of novel bacterial strains for integrated solar-bioelectrokinetic of soil contaminated with heavy petroleum hydrocarbons, Chemosphere, 2019, 237, 124514, DOI:10.1016/j.chemosphere.2019.124514.
- P. Logeshwaran, M. Megharaj, S. Chadalavada, M. Bowman and R. Naidu, Petroleum hydrocarbons (PH) in groundwater aquifers: an overview of environmental fate, toxicity, microbial degradation and risk-based remediation approaches, Environ. Technol. Innov., 2018, 10, 175–193 CrossRef.
- O. Hentati, R. Lachhab, M. Ayadi and M. Ksibi, Toxicity assessment for petroleum-contaminated soil using terrestrial invertebrates and plant bioassays, Environ. Monit. Assess., 2013, 185, 2989–2998 CrossRef CAS.
- C. H. ChaIneau, J. L. Morel and J. Oudot, Microbial degradation in soil microcosms of fuel oil hydrocarbons from drilling cuttings, Environ. Sci. Technol., 1995, 29, 1615–1621 CrossRef CAS.
- J. P. Del'Arco and F. P. De Franca, Influence of oil contamination levels on hydrocarbon biodegradation in sandy sediment, Environ. Pollut., 2001, 112, 515–519 CrossRef.
-
NRC, Oil in the Sea III: Inputs, Fates, and Effects, The National Academies Press, Washington, DC, 2003, p. 280, DOI:10.17226/10388.
- P. E. Benville, Jr, J. A. Whipple and M. B. Eldridge, Acute toxicity of seven alicyclic hexanes to striped bass, Morone saxatilis, and bay shrimp, Crangon franciscorum, in seawater, Calif. Fish Game, 1985, 71, 132–140 Search PubMed.
- R. B. Spies, The biological effects of petroleum hydrocarbons in the sea: assessments from the field and microcosms, Long-Term Environ. Eff. Offshore Oil Gas Dev., 1987, 411–467 Search PubMed.
-
CCME, Canada-Wide standards for petroleum hydrocarbons (PHCs) in soil, Canadian Council of Ministers of the Environment, Winnipeg, MN, Canada, 2000 Search PubMed.
- M. Barron, T. Podrabsky, S. Ogle and R. Ricker, Are aromatic hydrocarbons the primary determinant of petroleum toxicity to aquatic organisms?, Aquat. Toxicol., 1999, 46, 253–268 CrossRef CAS.
- H. C. Xu, Y. N. Wu, X. M. Xu, M. Gu, D. Y. Jiang, J. L. Xue and L. Li, Degradation properties of petroleum degrading bacteria immobilized on modified corn straw in marine environment, Pet. Sci. Technol., 2018, 36, 2043–2048 CrossRef CAS.
- J. Q. Sun, L. Xu, X. Y. Liu, G. F. Zhao, H. Cai, Y. Nie and X. L. Wu, Functional Genetic Diversity and Culturability of Petroleum-Degrading Bacteria Isolated From Oil-Contaminated Soils, Front. Microbiol., 2018, 9, 1332, DOI:10.3389/fmicb.2018.01332.
- B. Q. Liu, J. P. Liu, M. T. Ju, X. J. Li and Q. L. Yu, Purification and characterization of biosurfactant produced by Bacillus licheniformis Y-1 and its application in remediation of petroleum contaminated soil, Mar. Pollut. Bull., 2016, 107, 46–51 CrossRef CAS.
- S. H. Lee, B. I. Oh and J. G. Kim, Effect of various amendments on heavy mineral oil bioremediation and soil microbial activity, Bioresour. Technol., 2008, 99, 2578–2587 CrossRef CAS.
- D. E. Wellman, A. L. Ulery, M. P. Barcellona and S. Duerr-Auster, Animal waste-enhanced degradation of hydrocarbon-contaminated soil, Soil Sediment Contam., 2001, 10, 511–523 CrossRef CAS.
- K. Q. Ding, Y. M. Luo, T. H. Sun and P. J. Li, Bioremediation of soil contaminated with petroleum using forced-aeration composting, Pedosphere, 2002, 12, 145–150 CAS.
- R. U. Meckenstock, M. Boll, H. Mouttaki, J. S. Koelschbach, P. C. Tarouco, P. Weyrauch, X. Y. Dong and A. M. Himmelberg, Anaerobic degradation of benzene and polycyclic aromatic hydrocarbons, J. Mol. Microbiol. Biotechnol., 2016, 26, 92–118 CrossRef CAS.
- N. Das and P. Chandran, Microbial degradation of petroleum hydrocarbon contaminants: an overview, Biotechnol. Res. Int., 2011, 2011, 1–13 Search PubMed.
- W. Fritsche and M. Hofrichter, Aerobic degradation by microorganisms, Biotechnology, 2000, 11, 146–164 Search PubMed.
- T. J. McGenity, B. D. Folwell, B. A. McKew and G. O. Sanni, Marine crude-oil biodegradation: a central role for interspecies interactions, Aquat. Biosyst., 2012, 8, 10, DOI:10.1186/2046-9063-8-10.
- S. N. Singh, B. Kumari and S. Mishra, Microbial degradation of alkane, Environ. Sci. Eng., 2012, 439–469, DOI:10.1007/978-3-642-23789-8_17.
- M. F. Imron and H. S. Titah, Optimization of diesel biodegradation by Vibrio alginolyticus using Box-Behnken design, Environ. Eng. Res., 2018, 23, 374–382 CrossRef.
- R. Bartha, Biotechnology of petroleum pollutant biodegradation, Microb. Ecol., 1986, 12, 155–172 CrossRef CAS.
- M. R. Smith, The biodegradation of aromatic hydrocarbons by bacteria, Biodegradation, 1990, 1, 191–206 CrossRef CAS.
- W. C. Suen and D. T. Gibson, Isolation and preliminary characterization of the subunits of the terminal component of naphthalene dioxygenase from Pseudomonas putida NCIB 9816-4, J. Bacteriol., 1993, 175, 5877–5881 CrossRef CAS.
- A. Truskewycz, T. D. Gundry, L. S. Khudur, A. Kolobaric, M. Taha, A. Aburto-Medina, A. S. Ball, E. Shahsavari and Ł. Chrzanowski, Petroleum Hydrocarbon Contamination in Terrestrial Ecosystems—Fate and Microbial Responses, Molecules, 2019, 24, 3400, DOI:10.3390/molecules24183400.
- P. D. Boehm, D. L. Fiest, D. Mackay and S. Paterson, Physical-chemical weathering of petroleum hydrocarbons from the IXTOC I blowout: chemical measurements and a weathering model, Environ. Sci. Technol., 1982, 16, 498–505 CrossRef CAS.
- R. M. Atlas and R. Bartha, Degradation and mineralization of petroleum in sea water: limitation by nitrogen and phosphorous, Biotechnol. Bioeng., 1972, 14, 309–318 CrossRef CAS.
- D. M. Ward, R. M. Atlas, P. D. Boehm and J. A. Calder, Microbial Biodegradation and Chemical Evolution of Oil from the Amoco Spill, Ambio, 1980, 9, 277–283 CAS.
- C. M. R. Almeida, I. Reis, M. N. Couto, A. A. Bordalo and A. P. Mucha, Potential of the microbial community present in an unimpacted beach sediment to remediate petroleum hydrocarbons, Environ. Sci. Pollut. Res., 2013, 20, 3176–3184 CrossRef CAS.
- C. W. Chong, G. A. Tan, R. C. Wong, M. J. Riddle and I. K. Tan, DGGE fingerprinting of bacteria in soils from eight ecologically different sites around Casey Station, Antarctica, Polar Biol., 2009, 32, 853–860 CrossRef.
- F. F. Evans, A. S. Rosado, G. V. Sebastián, R. Casella, P. L. Machado, C. Holmström, S. Kjelleberg, J. D. Elsas and L. Seldin, Impact of oil contamination and biostimulation on the diversity of indigenous bacterial communities in soil microcosms, FEMS Microbiol. Ecol., 2004, 49, 295–305 CrossRef CAS.
- M. S. Safdari, H. R. Kariminia, M. Rahmati, F. Fazlollahi, A. Polasko, S. Mahendra, W. V. Wilding and T. H. Fletcher, Development of bioreactors for comparative study of natural attenuation, biostimulation, and bioaugmentation of petroleum-hydrocarbon contaminated soil, J. Hazard. Mater., 2018, 342, 270–278 CrossRef CAS.
- M. Nikolopoulou, N. Pasadakis and N. Kalogerakis, Enhanced bioremediation of crude oil utilizing lipophilic fertilizers, Desalination, 2007, 211, 286–295 CrossRef CAS.
- G. Płaza, G. Nałęcz-Jawecki, K. Ulfig and R. L. Brigmon, The application of bioassays as indicators of petroleum-contaminated soil remediation, Chemosphere, 2005, 59, 289–296 CrossRef.
- S. A. Mills and W. T. Frankenberger, Evaluation of phosphorus sources promoting bioremediation of diesel fuel in soil, Bull. Environ. Contam. Toxicol., 1994, 53, 280–284 CrossRef CAS.
- S. Khaitan, S. Kalainesan, L. E. Erickson, P. Kulakow, S. Martin, R. Karthikeyan, S. L. L. Hutchinson, L. C. Davis, T. H. Illangasekare and C. Ng'oma, Remediation of sites contaminated by oil refinery operations, Environ. Prog., 2006, 25, 20–31 CrossRef CAS.
- E. W. Liebeg and T. J. Cutright, The investigation of enhanced bioremediation through the addition of macro and micro nutrients in a PAH contaminated soil, Int. Biodeterior. Biodegradation, 1999, 44, 55–64 CrossRef CAS.
- X. Wang, Z. Cai, Q. X. Zhou, Z. N. Zhang and C. H. Chen, Bioelectrochemical stimulation of petroleum hydrocarbon degradation in saline soil using U-tube microbial fuel cells, Biotechnol. Bioeng., 2012, 109, 426–433 CrossRef CAS.
- K. Kamaluddeen, M. B. Yerima, T. R. Abu and Y. Deeni, Biostimulatory Effect of Processed Sewage Sludge in Bioremediation of Engine Oil Contaminated soils, Int. J. Sci. Technol. Res., 2016, 5, 203–207 Search PubMed.
- F. V. Adams, A. Niyomugabo and O. P. Sylvester, Bioremediation of crude oil contaminated soil using agricultural wastes, Procedia Manuf., 2017, 7, 459–464 CrossRef.
- X. Zhu, B. Chen, L. Zhu and B. Xing, Effects and mechanisms of biochar-microbe interactions in soil improvement and pollution remediation: a review, Environ. Pollut., 2017, 227, 98–115 CrossRef CAS.
- X. Han, H. Hu, X. Shi, L. Zhang and J. He, Effects of different agricultural wastes on the dissipation of PAHs and the PAH-degrading genes in a PAH-contaminated soil, Chemosphere, 2017, 172, 286–293 CrossRef CAS.
- Y. Liao, X. Min, Z. Yang, L. Chai, S. Zhang and Y. Wang, Physicochemical and biological quality of soil in hexavalent chromium-contaminated soils as affected by chemical and microbial remediation, Environ. Sci. Pollut. Res., 2014, 21, 379–388 CrossRef CAS.
- S. Ye, G. Zeng, H. Wu, C. Zhang, J. Dai, J. Liang, J. Yu, X. Ren, H. Yi, M. Cheng and C. Zhang, Biological technologies for the remediation of co-contaminated soil, Crit. Rev. Biotechnol., 2017, 37, 1062–1076 CrossRef CAS.
- A. Godoy-Faúndez, B. Antizar-Ladislao, L. Reyes-Bozo, A. Camano and C. Sáez-Navarrete, Bioremediation of contaminated mixtures of desert mining soil and sawdust with fuel oil by aerated in-vessel composting in the Atacama Region (Chile), J. Hazard. Mater., 2008, 151, 649–657 CrossRef.
- J. G. Leahy and R. R. Colwell, Microbial degradation of hydrocarbons in the environment, Microbiol. Mol. Biol. Rev., 1990, 54, 305–315 CrossRef CAS.
- A. R. Johnsen, L. Y. Wick and H. Harms, Principles of microbial PAH-degradation in soil, Environ. Pollut., 2005, 133, 71–84 CrossRef CAS.
- C. N. Mulligan, R. N. Yong and B. F. Gibbs, Surfactant-enhanced remediation of contaminated soil: a review, Eng. Geol., 2001, 60, 371–380 CrossRef.
- S. G. Costa, M. Nitschke, F. Lépine, E. Déziel and J. Contiero, Structure, properties and applications of rhamnolipids produced by Pseudomonas aeruginosa L2-1 from cassava wastewater, Process Biochem., 2010, 45, 1511–1516 CrossRef CAS.
- S. H. Kim, E. J. Lim, S. O. Lee, J. D. Lee and T. H. Lee, Purification and characterization of biosurfactants from Nocardia sp. L-417, Biotechnol. Appl. Biochem., 2000, 31, 249–253 CrossRef CAS.
- X. Mao, R. Jiang, W. Xiao and J. Yu, Use of surfactants for the remediation of contaminated soils: a review, J. Hazard. Mater., 2015, 285, 419–435 CrossRef CAS.
- S. Paria, Surfactant-enhanced remediation of organic contaminated soil and water, Adv. Colloid Interface Sci., 2008, 138, 24–58 CrossRef CAS.
- D. A. Edwards, Z. Liu and R. G. Luthy, Surfactant solubilization of organic compounds in soil/aqueous systems, J. Environ. Eng., 1994, 120, 5–22 CrossRef CAS.
- A. B. Moldes, R. Paradelo, D. Rubinos, R. Devesa-Rey, J. M. Cruz and M. T. Barral, Ex situ treatment of hydrocarbon-contaminated soil using biosurfactants from Lactobacillus pentosus, J. Agric. Food Chem., 2011, 59, 9443–9447 CrossRef CAS.
- E. Kaczorek, T. Jesionowski, A. Giec and A. Olszanowski, Cell surface properties of Pseudomonas stutzeri in the process of diesel oil biodegradation, Biotechnol. Lett., 2012, 34, 857–862 CrossRef CAS.
- M. Bao, Y. Pi, L. Wang, P. Sun, Y. Li and L. Cao, Lipopeptide biosurfactant production bacteria Acinetobacter sp. D3-2 and its biodegradation of crude oil, Environ. Sci.: Processes Impacts, 2014, 16, 897–903 RSC.
- T. M. April, S. P. Abbott, J. M. Foght and R. S. Currah, Degradation of hydrocarbons in crude oil by the ascomycete Pseudallescheria boydii (Microascaceae), Can. J. Microbiol., 1998, 44, 270–278 CrossRef CAS.
- H. S. Joo, P. M. Ndegwa, M. Shoda and C. G. Phae, Bioremediation of oil-contaminated soil using Candida catenulata and food waste, Environ. Pollut., 2008, 156, 891–896 CrossRef CAS.
- S. Mishra, J. Jyot, R. C. Kuhad and B. Lal, Evaluation of inoculum addition to stimulate in situ bioremediation of oily-sludge-contaminated soil, Appl. Environ. Microbiol., 2001, 67, 1675–1681 CrossRef CAS.
- M. M. Fogel, A. R. Taddeo and S. Fogel, Biodegradation of chlorinated ethenes by a methane-utilizing mixed culture, Appl. Environ. Microbiol., 1986, 51, 720–724 CrossRef CAS.
- F. M. Ghazali, R. N. Z. A. Rahman, A. B. Salleh and M. Basri, Biodegradation of hydrocarbons in soil by microbial consortium, Int. Biodeterior. Biodegrad., 2004, 54, 61–67 CrossRef CAS.
- H. Mikesková, Č. Novotný and K. Svobodová, Interspecific interactions in mixed microbial cultures in a biodegradation perspective, Appl. Microbiol. Biotechnol., 2012, 95, 861–870 CrossRef.
- V. S. Cerqueira, E. B. Hollenbach, F. Maboni, M. H. Vainstein, F. A. Camargo, R. P. Maria do Carmo and F. M. Bento, Biodegradation potential of oily sludge by pure and mixed bacterial cultures, Bioresour. Technol., 2011, 102, 11003–11010 CrossRef CAS.
- K. S. M. Rahman, T. J. Rahman, P. Lakshmanaperumalsamy and I. M. Banat, Towards efficient crude oil degradation by a mixed bacterial consortium, Bioresour. Technol., 2002, 85, 257–261 CrossRef CAS.
- L. Chen, Y. Chen, L. Chen and W. L. Chen, Study on fungi-bacteria augmented remediation of petroleum contaminated soil from Northwest of China, J. Food, Agric. Environ., 2009, 7, 750–753 CAS.
- R. Q. Yang, G. S. Zhang, S. W. Li, F. Moazeni, Y. S. Li, Y. N. Wu, W. Zhang, T. Chen, G. X. Liu, B. L. Zhang and X. K. Wu, Degradation of crude oil by mixed cultures of bacteria isolated from the Qinghai-Tibet plateau and comparative analysis of metabolic mechanisms, Environ. Sci. Pollut. Res., 2019, 26, 1834–1847 CrossRef CAS.
- M. Russel, X. Li, M. Qu, M. Wu, L. Liu and M. M. Alam, Exploring the novel indigenous strains for degrading the crude oil contaminants in soil sample, Int. J. Environ. Sci. Technol., 2019, 16, 5657–5668 CrossRef CAS.
- Y. J. Liu, A. N. Zhang and X. C. Wang, Biodegradation of phenol by using free and immobilized cells of Acinetobacter sp. XA05 and Sphingomonas sp. FG03, Biochem. Eng. J., 2009, 44, 187–192 CrossRef CAS.
- Y. Wu, L. Xia, Z. Yu, S. Shabbir and P. G. Kerr, In situ bioremediation of surface waters by periphytons, Bioresour. Technol., 2014, 151, 367–372 CrossRef CAS.
- I. Eş, J. D. G. Vieira and A. C. Amaral, Principles, techniques, and applications of biocatalyst immobilization for industrial application, Appl. Microbiol. Biotechnol., 2015, 99, 2065–2082 CrossRef.
- A. Dzionek, D. Wojcieszyńska and U. Guzik, Natural carriers in bioremediation: a review, Electron. J. Biotechnol., 2016, 19, 28–36 CrossRef.
- Y. Kourkoutas, A. Bekatorou, I. M. Banat, R. Marchant and A. A. Koutinas, Immobilization technologies and support materials suitable in alcohol beverages production: a review, Food Microbiol., 2004, 21, 377–397 CrossRef CAS.
- M. Bilal, M. Asgher, R. Parra-Saldivar, H. Hu, W. Wang, X. Zhang and H. M. Iqbal, Immobilized ligninolytic enzymes: an innovative and environmental responsive technology to tackle dye-based industrial pollutants–a review, Sci. Total Environ., 2017, 576, 646–659 CrossRef CAS.
- S. C. S. Martins, C. M. Martins, L. M. C. G. Fiúza and S. T. Santaella, Immobilization of microbial cells: a promising tool for treatment of toxic pollutants in industrial wastewater, Afr. J. Biotechnol., 2013, 12, 4412–4418 CrossRef CAS.
- S. Siripattanakul, W. Wirojanagud, J. McEvoy and E. Khan, Effect of cell-to-matrix ratio in polyvinyl alcohol immobilized pure and mixed cultures on atrazine degradation, Water, Air, Soil Pollut., 2008, 8, 257–266 CrossRef CAS.
- A. Yunoki, E. Tsuchiya, Y. Fukui, A. Fujii and T. Maruyama, Preparation of inorganic/organic polymer hybrid microcapsules with high encapsulation efficiency by an electrospray technique, ACS Appl. Mater. Interfaces, 2014, 6, 11973–11979 CrossRef CAS.
- R. Paliwal, S. Uniyal and J. P. N. Rai, Evaluating the potential of immobilized bacterial consortium for black liquor biodegradation, Environ. Sci. Pollut. Res., 2015, 22, 6842–6853 CrossRef CAS.
- P. Zajkoska, M. Rebroš and M. Rosenberg, Biocatalysis with immobilized Escherichia coli, Appl. Microbiol. Biotechnol., 2013, 97, 1441–1455 CrossRef CAS.
- Y. Xu and M. Lu, Bioremediation of crude oil-contaminated soil: comparison of different biostimulation and bioaugmentation treatments, J. Hazard. Mater., 2010, 183, 395–401 CrossRef CAS.
- Y. Liang, X. Zhang, D. Dai and G. Li, Porous biocarrier-enhanced biodegradation of crude oil contaminated soil, Int. Biodeterior. Biodegrad., 2009, 63, 80–87 CrossRef CAS.
- D. Su, P. Li, X. Wang, F. Stagnitti and X. Xiong, Biodegradation of benzo [a] pyrene in soil by immobilized fungus, Environ. Eng. Sci., 2008, 25, 1181–1188 CrossRef CAS.
- C. J. Cunningham, I. B. Ivshina, V. I. Lozinsky, M. S. Kuyukina and J. C. Philp, Bioremediation of diesel-contaminated soil by microorganisms immobilised in polyvinyl alcohol, Int. Biodeterior. Biodegrad., 2004, 54, 167–174 CrossRef CAS.
- P. H. Pritchard, Use of inoculation in bioremediation, Curr. Opin. Biotechnol., 1992, 3, 232–243 CrossRef CAS.
- B. Chen and M. Yuan, Enhanced sorption of polycyclic aromatic hydrocarbons by soil amended with biochar, J. Soils Sediments, 2011, 11, 62–71 CrossRef CAS.
- M. H. El-Naas, S. A. Al-Muhtaseb and S. Makhlouf, Biodegradation of phenol by Pseudomonas putida immobilized in polyvinyl alcohol (PVA) gel, J. Hazard. Mater., 2009, 164, 720–725 CrossRef CAS.
- Z. J. Ren and A. K. Umble, Recover wastewater resources locally, Nature, 2016, 529, 25 CrossRef CAS.
- D. R. Bond, D. E. Holmes, L. M. Tender and D. R. Lovley, Electrode-reducing microorganisms that harvest energy from marine sediments, Science, 2002, 295, 483–485 CrossRef CAS.
- X. J. Li, X. Wang, Q. Zhao, L. L. Wan, Y. T. Li and Q. X. Zhou, Carbon fiber enhanced bioelectricity generation in soil microbial fuel cells, Biosens. Bioelectron., 2016, 85, 135–141 CrossRef CAS.
- B. E. Logan and J. M. Regan, Microbial fuel cells-challenges and applications, Environ. Sci. Technol., 2006, 40, 5172–5180 CrossRef CAS.
- X. Li, X. Wang, L. Weng, Q. Zhou and Y. Li, Microbial fuel cells for organic-contaminated soil remedial applications: a review, Energy Technol., 2017, 5, 1156–1164 CrossRef CAS.
- D. R. Lovley, Microbial fuel cells: novel microbial physiologies and engineering approaches, Curr. Opin. Biotechnol., 2006, 17, 327–332 CrossRef CAS.
- S. Oh, B. Min and B. E. Logan, Cathode performance as a factor in electricity generation in microbial fuel cells, Environ. Sci. Technol., 2004, 38, 4900–4904 CrossRef CAS.
- C. Wang, H. Deng and F. Zhao, The remediation of chromium (VI)-contaminated soils using microbial fuel cells, Soil Sediment Contam., 2016, 25, 1–12 CrossRef CAS.
- N. Habibul, Y. Hu and G. P. Sheng, Microbial fuel cell driving electrokinetic remediation of toxic metal contaminated soils, J. Hazard. Mater., 2016, 318, 9–14 CrossRef CAS.
- X. Wang, Q. Wang, S. Wang, F. Li and G. Guo, Effect of biostimulation on community level physiological profiles of microorganisms in field-scale biopiles composed of aged oil sludge, Bioresour. Technol., 2012, 111, 308–315 CrossRef CAS.
- S. Srikanth, M. Kumar, D. Singh, M. P. Singh and B. P. Das, Electro-biocatalytic treatment of petroleum refinery wastewater using microbial fuel cell (MFC) in continuous mode operation, Bioresour. Technol., 2016, 221, 70–77 CrossRef CAS.
- X. Li, X. Wang, L. Wan, Y. Zhang, N. Li, D. Li and Q. Zhou, Enhanced biodegradation of aged petroleum hydrocarbons in soils by glucose addition in microbial fuel cells, J. Chem. Technol. Biotechnol., 2016, 91, 267–275 CrossRef CAS.
- L. Zhao, J. H. Deng, H. J. Hou, J. C. Li and Y. K. Yang, Investigation of PAH and oil degradation along with electricity generation in soil using an enhanced plant-microbial fuel cell, J. Cleaner Prod., 2019, 221, 678–683 CrossRef CAS.
|
This journal is © The Royal Society of Chemistry 2021 |