Assessment of carbon dioxide removal potential via BECCS in a carbon-neutral Europe†
Received
2nd March 2021
, Accepted 13th April 2021
First published on 13th April 2021
Abstract
Bioenergy with Carbon Capture and Storage (BECCS) is a Carbon Dioxide Removal (CDR) technology that will likely be necessary to reach global net-zero carbon dioxide emission goals. In order to assess the European BECCS potential, we quantify at 1 km resolution the technical potential for biogenic CDR considering seven different BECCS configurations that do not require purpose-grown bio-energy plantations. Combining process engineering with geospatial assessment, we find that up to 5% of 2018 European emissions, or about 200 million tons CO2 per year, could be mitigated with biogenic CDR from BECCS. Such potential is at the lower bound of the range of projected CDR needs for Europe. Two thirds of this potential is from existing point sources (pulp and paper, biomass co-fired, waste-to-energy, and wastewater treatment facilities), while one third is from distributed sources (crop residues, organic food waste, and livestock manure). From a geopolitical perspective, only a few European countries reach or exceed their CDR needs via BECCS. Those countries, which will not be able to reach carbon-neutrality with domestic biomass resources, will likely need to resort to other CDR strategies or import biomass from abroad. From a geographic perspective, distances from emission sources and storage sites are rather unfavourably distributed. Based on our quantitative assessment we conclude that: (1) tapping into the whole 200 million tons CO2 per year will be challenging because of the unfavourable source–sink distance distribution; (2) there is a need for more and better distributed prospective CO2 storage sites in Europe; and (3) the mobilization of these large amounts of CO2 will require the realization of a Europe-wide CO2 transportation network.
Broader context
Bioenergy with Carbon Capture and Storage (BECCS) is a carbon dioxide removal (CDR) technology which involves the capture and permanent sequestration of biogenic CO2. To meet climate goals by 2050, cumulative values of 7.5 billion tons of CO2 may have to be sequestered through BECCS in Europe. In light of concerns over negative environmental impacts of large-scale bio-energy plantations, recent work has focused on BECCS opportunities from existing infrastructure, namely, pulp and paper, biomass co-firing, waste-to-energy, and wastewater treatment facilities. Moreover, distributed sources of biogenic CO2 (crop residues, organic food waste and livestock manure) could be collected and used for biogenic CDR. Considering these prospective BECCS opportunities, we assess biogenic CDR potential in Europe. We find that Europe has enough biomass resources to remove 5% of its 2018 greenhouse gas emissions, or about 200 million tons CO2 per year. However, if more CDR is required, European countries will likely need to resort to other CDR technologies or import biomass from other countries to reach net-zero emissions.
|
1. Introduction
Europe aims to be carbon-neutral by 2050 – that is, an economy with net-zero greenhouse gas emissions.1 Although Europe does not have a carbon dioxide removal (CDR) strategy yet,1–4 CDR via bio-energy with carbon capture and storage (BECCS) will most likely be needed to counteract residual emissions from hard-to-abate sectors.5–12 To reach carbon-neutrality, it has been estimated that up to 7.5 billion tons of CO2 may have to be sequestered through BECCS in Europe until 2050.13 BECCS involves the capture and permanent sequestration of biogenic CO2 produced during energy conversion from biomass14–17 and is considered promising due to its degree of commercial maturity.18–21 Recently, Sandalow et al., 2021 advanced the notion of biomass carbon removal and storage, suggesting that the value of using biomass for CDR may exceed the value of using biomass for bio-energy production.22 While several previous studies estimated the potential bio-energy generation from biomass resources in Europe,23–25 the European biogenic CDR potential from domestic biomass resources has not been estimated yet.
Various studies have raised concerns on the biophysical feasibility and socio-environmental effects of large-scale purpose-grown biomass plantations for BECCS,26–28 including competition for agricultural and natural land,29–31 increase in food crop prices,31,32 biodiversity loss,33 and water scarcity exacerbation.34,35 Therefore, technology developers and policymakers should ensure that BECCS operations reliably sequester CO2 and minimize environmental impacts. For example, Europe's renewable energy targets have had the unintended consequence of accelerating deforestation rates within Europe,36 outsourcing environmental damages to other countries,37 and potentially increasing net carbon emissions.38,39 These negative effects could worsen under European climate-neutrality targets if mitigation efforts through BECCS shift across countries especially in the Global South.40
With global food demand expected to increase by up to 100% by 2050,41 there is a pressing need to develop BECCS technologies that do not rely on dedicated energy feedstocks.42,43 There are several different BECCS processes that, if deployed, could avoid competition with food production while providing near-term opportunities for biogenic CDR.22,44,45 For example, the pulp and paper industry utilizes biomass for stationary heat and power production and emits large quantities of biogenic CO2.46,47 Waste-to-energy and biomass co-fired power plants emit biogenic CO2 from municipal solid waste and biomass combustion.48,49 The capture of biogenic CO2 from anaerobic treatment of biomass can be applied at existing biogas facilities that process urban wastewater.45 Moreover, biogenic CO2 could also be captured upon biogas production from crop residues, household organic food waste, and livestock manure.45 In biogas facilities, CO2 is separated at high purity during biogas upgrading to bio-methane (or renewable natural gas). Because policy makers are investigating pathways to reach net-zero emissions by 2050, there is a pressing need for a geospatial assessment50 that determines the potential biogenic CO2 that could be captured from these prospective BECCS processes in Europe.51,52
Here, we quantify the technical potential for biogenic CDR (Mtons CO2 per year) at 1 km resolution for 30 European countries (European Union countries, Switzerland, the United Kingdom, and Norway). Biogenic CDR is here defined as the amount of CO2 captured and sequestered from biomass conversion to bio-energy. Using geospatial assessment, the potential for biogenic CDR is assessed considering existing point sources and distributed biomass feedstock that can be used for BECCS. For existing point sources we consider: (1) industrial plants for the production of pulp and paper; (2) incineration of municipal non-hazardous waste, or waste-to-energy plants; (3) biomass co-fired power stations (or bio-power plants); and (4) urban wastewater treatment plants, which can produce biogas. For distributed sources we consider three BECCS technologies that could be deployed to produce renewable natural gas, namely from (5) crop residues, (6) household organic food waste, and (7) livestock manure. For these seven prospective BECCS processes, we first design BECCS supply chains and determine biogenic CDR efficiencies.35 Second, drawing from existing spatially-explicit database of distributed biomass availability23–25 and CO2 emissions from European point source emitters,53,54 we quantify the geospatial distribution of biogenic CDR. Third, we quantify the extent to which domestic biogenic CDR resources would allow for the adoption of BECCS as a means to generate CDR to mitigate hard-to-abate emissions. Fourth, we determine fossil CO2 capture potential from existing bio-energy point sources. Finally, by matching carbon sources with prospective CO2 sinks for permanent geological sequestration, we determine the quantity of CO2 available at different source–sink transport distances.
Results enable us to identify countries where biogenic CDR from domestic BECCS resources can provide sufficient CDR that will likely be needed to reach net-zero emissions by 2050. Our analysis identifies overlooked and near-term opportunities for BECCS in Europe and provides a vision on the future role of domestic biogenic CDR to meet net-zero emission goals.
2. Methods
2.1 BECCS technology chain
We design BECCS technology chains from CO2 source to sink considering feasible biomass feedstock-technology combinations. Depending on biomass type and bio-energy process, different biogenic CO2 quantities are generated and different CO2 capture rates are technically and economically achievable. Subsequently, captured CO2 is assumed to be transported through a shared CO2 network to a suitable storage site for permanent geological sequestration.
2.2 Assessment of biogenic CO2 emissions from existing point sources
We consider existing industrial processes that emits biogenic CO2, namely, pulp and paper mills, waste-to-energy plants, and biomass co-fired power plants. The location and annual CO2 emissions from these large point sources (each emitting more than 0.1 Mtons CO2 per year in year 2018) were taken from an open access dataset provided by the European Environmental Agency.54 Among already existing point sources, we also consider wastewater treatment plants as reported in the European Environmental Agency Waterbase dataset.53
For biomass co-fired power plants, the European Environmental Agency dataset reports both total and biogenic CO2 emissions. Some of these facilities are fossil-fuel-based thermal power plants that burn only a small fraction of biomass, while other facilities have only biomass as feedstock. Therefore, the share of biogenic CO2 emitted from the selected bio-power plants can vary from 1% to 100%.
For some pulp and paper mills, the European Environmental Agency dataset reports both total and biogenic CO2 emissions. For the remaining pulp and paper facilities, we assume that the quantity of biogenic CO2 emitted is 75% to 100% of total CO2 emissions, consistent with previous studies (Fig. 1).46,47
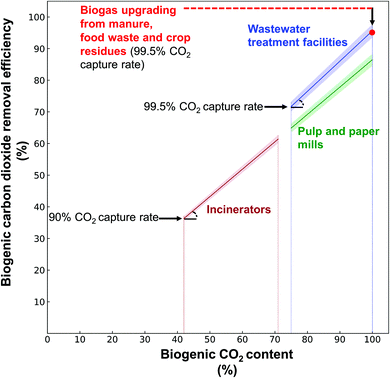 |
| Fig. 1 Biogenic CO2 content and biogenic carbon dioxide removal efficiencies from different BECCS technology chains. Solid lines represent the average scenario of CO2 losses in transport and injection; shaded areas show the estimated range of CO2 losses during transport and injection. Depending on the amount of biomass co-fired, biogenic CO2 content in biomass co-fired power plants can vary from 1% to 100%. | |
For incinerators, prior work estimates that 42% to 71% of total CO2 emissions are of biogenic origin (Fig. 1).55,57 Although the range considered here is representative of the seasonal variation in biogenic CO2 emissions, it is important to notice that biogenic CO2 emissions from incinerators have a significant seasonal variability due to the changes in municipal solid waste composition along the year.56
We consider wastewater facilities,53 where wastewater is treated through anaerobic digestion to produce biogas. Assuming that all CO2 emissions from wastewater treatment are of biogenic origin might lead to an underestimation of fossil CO2 emissions.58 In fact, a radiocarbon isotopes study conducted on Australian wastewater found that 4% to 14% of total organic carbon in wastewater is of fossil origin.58 Another study conducted in the United States determined that 25% of the organic carbon in wastewater is of fossil origin, most likely derived from cleaning products, pharmaceuticals, and other fossil-fuel-based products.59 Therefore, to capture the variability in biogenic CO2 content in wastewater, we here assume that between 75% to 100% of carbon emissions are biogenic (Fig. 1).
2.3 Assessment of biogenic CO2 from distributed sources
Spatially-explicit biomass availabilities at 1 km resolution were taken from open access datasets from the Joint Research Center by the European Commission.23–25 We consider biomass availability from crop residues, household organic food waste, and livestock manure. We here assume that crop residues, manure, and food waste have a 100% biogenic CO2 content (Fig. 1).
We use a 1 km resolution dataset from Scarlat et al., 2019 containing sustainable crop residues (kgDM per year, kg dry matter per year).25 Scarlat et al., 2019 assessed sustainable crop residues in Europe considering the physical amount of residues that could be removed from the field with machineries and without depleting soil organic carbon.25 For food waste, we use a 1 km resolution dataset containing spatially-explicit information of already composted household organic food waste in Europe (kgDM per year).23 For livestock manure, we use a 1 km resolution dataset containing bio-methane equivalent that can be produced by processing collectible manure through anaerobic digestion.24 Collectible manure is the weight of manure that could be technically removed from the field with machinery.
2.3.1 Assessment of CO2 emissions from distributed sources.
We assume application of anaerobic digestion technology for distributed biomass sources. Wastewater treatment facilities are here classified as already existing infrastructure, however, to our knowledge there are no publicly available datasets reporting CO2 emissions from these facilities. Therefore, we assess CO2 emissions from wastewater treatment plants assuming that organic material contained in wastewater is treated through anaerobic digestion to produce biogas. We consider a range of biogas yields from 18 to 26 L of biogas per person equivalent per day.60 Lower biogas yields and methane contents usually indicate irregularities in the anaerobic digestion process.60 We then assume that biogas from sewage sludge has a methane content by volume that ranges from 63% to 67%,60 the remaining fraction (33% to 37%) is considered to be CO2 available for capture and sequestration.
Crop residues are treated through an anaerobic digestion process into bio-methane. Bio-methane yields are assumed to be between 0.14 and 0.16 kg methane per kgDM.61 We then determine potential CO2 emissions assuming that biogas has a CO2 content by volume of 40%.45
Food waste is treated through an anaerobic digestion process into bio-methane. Bio-methane yields are assumed to be between 0.20 and 0.31 kg methane per kgDM.61 We then determine potential CO2 emissions assuming that biogas has a CO2 content by volume of 40%.45
Livestock manure is converted into biogas through anaerobic digestion. Biogas potential from collectible livestock manure is taken from a previous study from the Joint Research Center by the European Commission, which assessed biogas potentials considering livestock-specific biogas yields.24 We then determine potential CO2 emissions assuming that biogas has a CO2 content by volume that ranges from 35% to 45%.24,45
2.4 CO2 capture from bio-energy processes
Depending on the biomass conversion process, different CO2 capture rates are technically and economically achievable. For pulp and paper, incinerators and bio-power facilities, we assume a 90% CO2 capture rate (Fig. 1). This is an ubiquitous assumption in literature, however, going beyond 90% CO2 capture rate is feasible, although with higher economic costs.62 Importantly, because fossil and biogenic CO2 are mixed in the flue gas, by retrofitting already existing point sources with carbon capture units both biogenic and fossil CO2 will be captured. Therefore, the quantity of biogenic CDR at each point source depends on plant-specific biogenic CO2 content (Fig. 1).
Because a high purity stream of bio-methane from biogas upgrading is required for pipeline injection, in this study we assume that wastewater treatment facilities have a higher CO2 capture rate compared to other point source emitters (99.5% versus 90%) CO2 capture rate (Fig. 1).63 Similarly, for bio-methane production from crop residues, food waste and livestock manure we assume a CO2 capture rate of 99.5% (Fig. 1).
2.5 Transport and storage of CO2
To achieve carbon removal from BECCS technologies, captured CO2 is subsequently transported through a CO2 network to a suitable site for injection and permanent geological sequestration. Similarly to existing methane infrastructure, CO2 networks will likely have transport and injection leakages.35 Building on previous comprehensive studies on methane leakage rates from existing natural gas networks, we assume a suitable range of CO2 transport and injection leakages at the system level, ranging from 6% in a conservative scenario to 1.8% in an optimistic scenario.64,65 Importantly, Fig. 1 shows that biogenic CDR efficiencies have a small sensitivity to CO2 transport and injection losses and that CO2 capture rate and biogenic CO2 content are the main factors influencing biogenic CDR efficiencies.
2.6 Differentiation of biogenic CDR and negative emissions
This study determines the technical potential for biogenic CDR via BECCS in Europe. While a full life cycle assessment of the BECCS supply chains considered in this study is outside its scope, site-specific life cycle assessments are needed to determine if the biogenic CDR potentials will deliver negative emissions.66,67 Whether the biogenic CDR potential via BECCS delivers negative emissions, depends on the fact that: (1) biogenic CO2 is permanently removed and stored out of the atmosphere; and (2) the quantity of CO2 removed from the atmosphere is greater than cradle-to-grave life cycle greenhouse gas emissions associated with BECCS supply chains.66 One important consideration in the assessment of the carbon balance of biomass feedstock is the counterfactual, which is an assumption of what would occur to biomass feedstock in the case it would not be deployed for BECCS.45 Our assessment prioritize wastes and residues, which are typically burned or left to decompose on the field.45
3. Results
3.1 European biogenic CDR potential
For each BECCS supply chain, we employ our assumptions around biomass availability and biogenic CDR efficiencies to determine biogenic CDR potentials at high spatial resolution. By combining high resolution data of CO2 emissions from existing point sources with biogenic CDR efficiencies (Fig. 1), we determine the geospatial distribution of biogenic CDR from pulp and paper, incinerators, bio-power, and wastewater treatment facilities. Similarly, by combining high-resolution information of biomass availability with bio-energy yields and biogenic CDR efficiencies, we determine the geospatial distribution of biogenic CDR from crop residues, food waste, and livestock manure.
Fig. 2 and 3 show the potential geospatial distribution of existing and distributed biogenic CDR, respectively, in Europe in 2018. Existing industrial infrastructure emitting biogenic CO2 is mainly located in Northern European countries, while South-eastern European countries have fewer large industrial point sources emitting biogenic CO2 (Fig. 2). Considering distributed sources of biomass, we find that there is a large potential for biogenic CDR in regions with high population densities (e.g. Milan, London, Paris, Berlin metropolitan areas), and areas with intensive cropland and livestock production (such as, Northern Italy, Belgium, and the Netherlands) (Fig. 3). Fig. 3 also shows the location and the name of ten prospective storage sites for permanent geological sequestration of CO2 that are planned or in start-up phase in Europe.
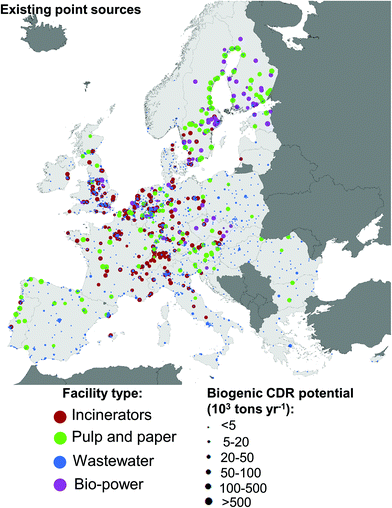 |
| Fig. 2 Geospatial distribution of biogenic carbon dioxide removal potential from existing point sources in Europe in 2018 (see ESI†). The figure shows incinerators, pulp and paper, and bio-power facilities emitting more than 0.1 Mtons CO2 per year,54 and wastewater treatment plants processing more than 100 000 population equivalent of wastewater per day.53 | |
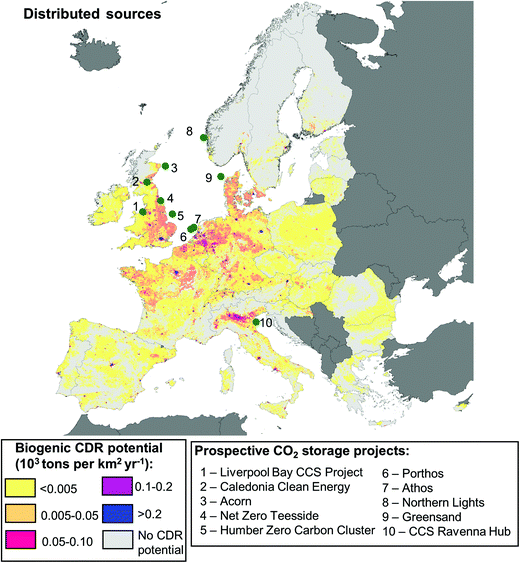 |
| Fig. 3 Geospatial distribution of biogenic carbon dioxide removal potential from distributed sources of biogenic CO2 in Europe. Distributed sources of biogenic CO2 are livestock manure, household organic food waste, and crop residues. The figure shows location and name of ten prospective carbon storage sites that are planned or in start-up phase in Europe. | |
Spatially-explicit biogenic CDR estimates have then been aggregated at different scales to determine total biogenic CDR potential. We estimate that the total potential for biogenic CDR in Europe is about 200 Mtons CO2 per year (Table 1). Of these 200 Mtons CO2 per year, two thirds are from already existing point sources and the remaining one third is from distributed sources (Table 1). With 62 Mtons CO2 per year, pulp and paper facilities have the greatest potential for biogenic CDR from existing point sources, followed by waste-to-energy facilities (36 Mtons CO2 per year), biomass co-fired plants (31 Mtons CO2 per year), and wastewater treatment plants (1.6 Mtons CO2 per year) (Table 1). Considering distributed sources, crop residues have the greatest potential for biogenic CDR (34 Mtons CO2 per year), followed by livestock manure (19 Mtons CO2 per year), and household organic food waste (18 Mtons CO2 per year) (Table 1). Since there is a variability in the parameters used to determine biogenic CO2 content, bio-energy conversion efficiency, and CO2 capture, transport and injection losses, Table 1 shows uncertainty ranges in biogenic CDR potentials from different BECCS configurations. Results are presented considering the average scenario, which represent the central values in original sources (Fig. 1). Conservative and optimistic scenarios consider the variability of biogenic CDR potentials and are depicted with error bars wherever necessary.
Table 1 Carbon dioxide removal potential from different BECCS configurations in Europe. Uncertainty ranges are shown in parentheses, determined by considering uncertainties in biogenic CO2 content, bio-energy conversion efficiency, and CO2 capture, transport and injection losses
Infrastructure |
BECCS configuration |
Biogenic CO2 (Mtons CO2 per year) |
Fossil CO2 (Mtons CO2 per year) |
Existing point sources |
Pulp and paper |
62 (±5) |
7 (±3) |
Incinerators |
36 (±9) |
28 (±10) |
Bio-power |
31 (±1) |
86 (±2) |
Wastewater |
1.6 (±0.6) |
0.2 (±0.1) |
Potential distributed sources |
Crop residues |
34 (±3) |
— |
Food waste |
18 (±4) |
— |
Manure |
19 (±8) |
— |
Total |
202 (±30) |
121 (±15) |
Fig. 4 shows country- and BECCS-specific biogenic CDR potentials. Sweden, with 31 Mtons CO2 per year, has the largest biogenic CDR potential among European countries, followed by Germany (28 Mtons CO2 per year), the United Kingdom (24 Mtons CO2 per year), Finland (23 Mtons CO2 per year), and France (22 Mtons CO2 per year) (Fig. 4a). We find that pulp and paper mills represent more than 60% of the share of biogenic CDR potential in Sweden, Finland, and Portugal (Fig. 4b). In the Netherlands, Switzerland, and Luxemburg more than 55% of domestic biogenic CDR potential could come from incinerators, while in the United Kingdom, Estonia, Chechia, and Slovenia more than 30% of biogenic CDR potential could be sourced by retrofitting biomass co-fired power plants (Fig. 4b). In France, Spain, Italy, and Poland more than 50% of the biogenic CDR potential would come from distributed BECCS sources (Fig. 4b).
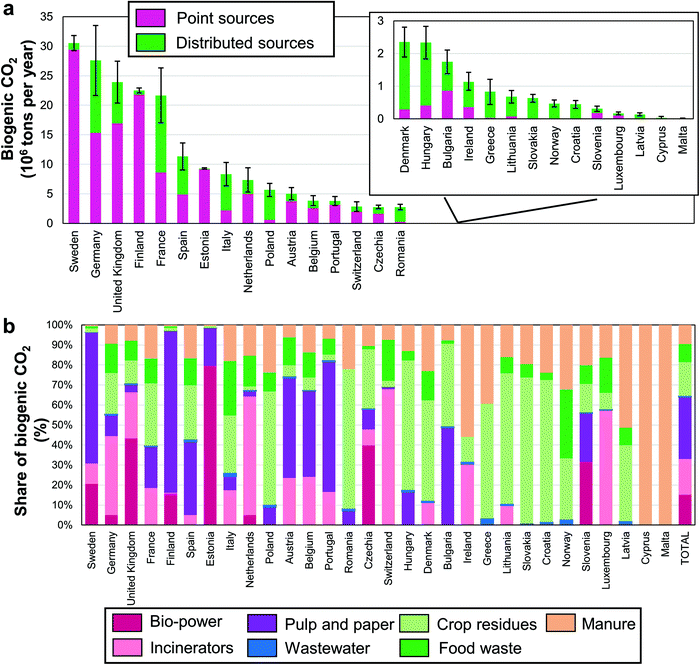 |
| Fig. 4 Country- and BECCS configuration-specific biogenic CDR potentials for European nations. (a) Country-specific biogenic CDR potentials. Interval bars depict the conservative and optimistic biogenic CDR potentials in each country. (b) BECCS configuration-specific contribution to national biogenic CDR potential considering the average scenario. | |
3.2 Fossil CO2 capture potential from existing bio-energy point sources
By retrofitting existing point sources with carbon capture units, both fossil and biogenic CO2 would be captured (Fig. 5). More specifically, the quantity of fossil CO2 captured depends on plant-specific emissions and biogenic CO2 content (Fig. 1). By aggregating plant-specific fossil and biogenic CO2 emissions that could be captured if existing infrastructure were retrofitted with carbon capture units, we estimate that the total fossil carbon capture potential from already existing facilities is 121 Mtons CO2 per year (Table 1); 71% of this is from biomass co-fired plants, while 23% and 6% is from incinerators and pulp and paper facilities, respectively (Table 1).
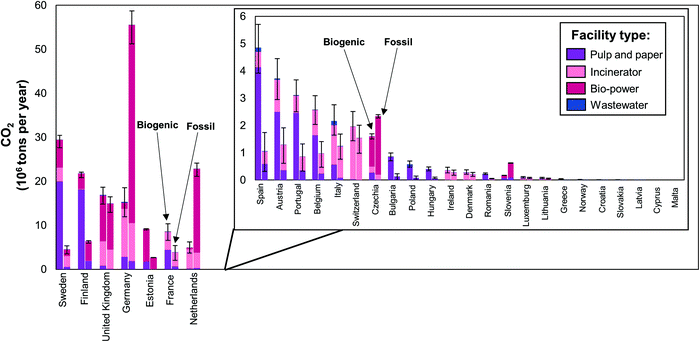 |
| Fig. 5 Country-specific fossil and biogenic carbon capture potentials from large existing point sources. The figure shows biogenic (right bars) and fossil (left bars) potentials by retrofitting large existing biogenic point sources with carbon capture units. Interval bars depict the conservative and optimistic potentials in each country. Germany has the greatest fossil carbon capture potential (56 Mtons CO2 per year), followed by the Netherlands (23 Mtons CO2 per year), and the United Kingdom (15 Mtons CO2 per year). | |
Our analysis shows country- and source-specific carbon capture potentials for both fossil and biogenic CO2 from existing bio-energy facilities (Fig. 5). The United Kingdom, Germany, and the Netherlands have large amounts of fossil CO2 emissions embedded in existing point sources. Therefore, these countries will have to capture large volumes of fossil CO2 to perform biogenic CDR from existing point sources, thus implying that realizing CDR objectives via BECCS will enable significant CO2 emissions mitigation. The downside of this is that it could require the transport of large quantities of CO2 without delivering large quantities of biogenic CDR.
3.3 Biogenic carbon source–sink matching
To ensure durable carbon removal, captured CO2 needs to be transported and injected into a suitable geological formation for permanent sequestration. To our knowledge, there are 10 prospective carbon storage sites available for general use that are planned or in start-up phase in Europe (Fig. 3). We perform a geospatial analysis to quantify the distribution of CO2 at different source–sink transport distances from these 10 prospective geological storage sites for carbon sink. The outcome is shown in Fig. 6, whereby Fig. 6a shows the cumulative emission-based distribution (Mtons CO2 per year) of source–sink CO2 transportation distances, whereas Fig. 6b shows the corresponding emission-based distribution (Mtons CO2 per year per km) of source–sink CO2 transportation distances. We find that 30% of European biogenic CDR potential (or 62 Mtons of biogenic CO2 per year) is located within a distance of 300 km to the 10 selected CO2 storage sites. Because point sources emitting large quantities of fossil CO2 are mainly located in Northern Europe, 55% of fossil CO2 capture potential is located within 300 km to prospective CO2 storage sites. The BECCS potential from distributed sources is on the other hand rather homogeneously distributed, with 50% of the potential located at least 500 km from a possible storage site.
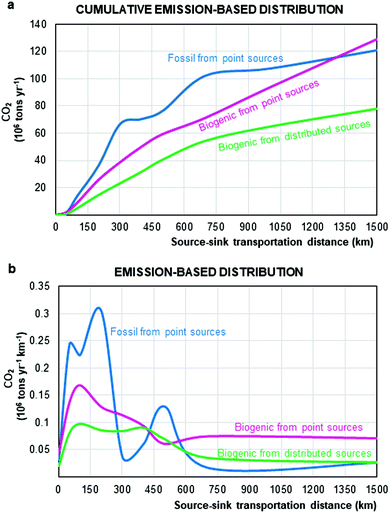 |
| Fig. 6 Emission-based distribution of source–sink transport amount and distance of European CO2. (a) Cumulative emission-based distribution of source–sink CO2 transportation distances from the 10 prospective CO2 storage sites reported in Fig. 3. (b) Emission-based distribution of source–sink CO2 transportation distances. | |
3.4 Potential BECCS contribution to European carbon-neutrality target
Using the country-specific biogenic CDR potentials estimated above, we estimate the extent to which biogenic CDR endowments could enable carbon-neutrality in EU countries. It is still not clear how much CDR will be needed by different European countries to reach net-zero emissions by 2050.3 In fact, the precise amount will depend on how fast European countries curb greenhouse gas emissions. According to an analysis published by the European Commission in 2018, about 10% (400 Mtons CO2 per year) of 2018 emissions will need to be removed through CDR to mitigate hard-to-abate emissions and reach climate-neutrality by 2050.1 More recently, Switzerland published its strategy to reach net-zero emissions by 2050 and it has estimated that around 26% (12 Mtons CO2 per year) of 2018 total emissions will need to be removed through CDR.68 Similarly, a recent study in California estimated that 29% (125 Mtons CO2 per year) of statewide emissions will need to be removed from the atmosphere to reach net-zero emissions by 2050.45
Given that nobody can for sure know the amount of CDR that will be needed to reach climate-neutrality by 2050, here, we consider that 5% to 30% of 2018 total greenhouse gas emissions will need to be removed from the atmosphere with CDR to mitigate hard-to-abate emissions.69 These scenarios allow us to establish an upper- and lower-bound on the CDR likely needed by each European country to reach net-zero emissions by 2050. Importantly, these numbers would increase if international equity and responsibility criteria were applied.4,70
According to the European Environmental Agency, in year 2018 total greenhouse gas emissions from the 30 European countries considered in this study were about 4000 Mtons CO2-equivalent per year. Therefore, we estimate that, to reach climate-neutrality, European countries will likely need to remove from the atmosphere between 200 Mtons CO2 per year (5% of 4000 Mtons CO2 per year) and 1200 Mtons CO2 per year (30% of the same figure). We can conclude that the overall BECCS potential calculated here allows to generate CDR to reach just the lower bound of this rather broad range. Some countries are better positioned to reach net-zero emissions with domestic BECCS endowments than others (Fig. 7). In fact, we find that Sweden, Estonia, and Finland will be able to mitigate more than 40% of their total emissions with domestic BECCS resources (Fig. 7); Sweden in fact 300% of them. Because of large existing BECCS point sources, Switzerland, Portugal, and Austria could mitigate just above 5% of their emissions with domestic biogenic CDR (Fig. 7). By deploying full biogenic CDR from BECCS endowments, the United Kingdom and France would be able to mitigate 5% of their emissions. However, Germany, Italy, Poland, Spain, and the Netherlands will not be able to mitigate 5% of their emissions even with full deployment of domestic BECCS resources (Fig. 7).
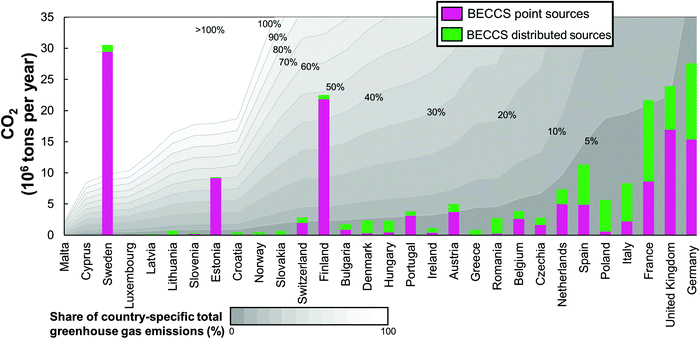 |
| Fig. 7 Comparison between domestic BECCS endowments and country-specific total greenhouse gas emissions. The gray-scale bar shows the share of year 2018 country specific total greenhouse gas emissions. Countries are listed in ascending order based on 2018 total greenhouse gas emissions. | |
4. Discussion and conclusions
Biogenic CDR through BECCS has a large untapped potential to help meet European carbon-neutrality goals. We assess the technical biogenic CDR potential from seven prospective BECCS technologies in Europe (Table 1). We consider BECCS configurations that require neither purpose-grown plantations, nor new forestry resources, nor additional land and water resources for biomass production, thus making the estimates made here conservative. Our analysis determines biogenic CO2 content and biogenic CDR efficiencies for each BECCS configuration (Fig. 1). We show that BECCS is a large, yet constrained, resource in Europe. There are about 200 Mtons of biogenic CO2 that could be technically deployed for CDR in Europe, which correspond to 5% of 2018 total European greenhouse gas emissions.
We find that 65% of the European biogenic CDR potential could come from the retrofitting of existing point sources with carbon capture and sequestration units. Because of their higher biogenic CO2 emissions, pulp and paper facilities have more potential to deliver net CDR than bio-power plants and incinerators. In fact, we find that 90% of CDR potential in pulp and papers mills would be of biogenic origin, while for incinerators and bio-power plants 43% and 73% of the carbon captured would be of fossil origin (Table 1). We find that 35% of the European biogenic CDR potential could come from distributed sources of biomass such as crop residues, food waste, and livestock manure. While, CO2 capture, transport and storage infrastructure will be needed to perform BECCS, to develop distributed sources of biomass, new anaerobic treatment facilities would also be required. Additional infrastructure would also be necessary to collect and transport biomass feedstock to processing facilities.
We assessed the feasibility to reach net-zero emissions by exploiting domestic biogenic CO2 sources. We show that opportunities for biogenic CDR differ markedly by country and BECCS configuration (Fig. 4). We show that under a high emissions reduction scenario, domestic BECCS resources are sufficient to meet European climate-neutrality in aggregate, although we also show that many countries do not possess sufficient BECCS potential to unilaterally meet their CDR quotas (Fig. 7). If BECCS were to be deployed at scale, Sweden, Finland and Estonia would have sufficient biogenic CDR potentials to exceed their national CDR quotas needed to reach carbon-neutrality. However, most European countries would not be able to meet CDR quotas with domestic BECCS endowments and would likely need to resort to other CDR strategies, outsource biomass from other countries, and cooperate with European nations that have abundant BECCS resources to offset their CDR quotas. This in turn would require a rapid growth in international trade of bio-energy – raising questions about global standards for bio-energy production, logistics and infrastructure.71
The European target to reach net-zero emissions by 2050 is very ambitious and requires urgent actions to reduce greenhouse gas emissions and mitigate residual ones. While European geological storage resources for CO2 are more than sufficient to meet CCS requirements under net-zero emission scenarios,72 the development of European-wide CO2 transport networks remains a technical, social, political and economic challenge. The BECCS potential calculated in this study allows to generate CDR to mitigate 5% (200 Mtons CO2 per year) of European greenhouse gas emissions. However, in the case more CDR is needed to mitigate hard-to-abate emissions, alternative CDR approaches, such as direct air capture and storage, will also need to be deployed. To deliver net-negative CDR through BECCS, most of the biogenic CDR potential assessed in this study will need to be transported over long distances for permanent geological sequestration (Fig. 6). Therefore, there is a pressing need to discover and develop additional CO2 storage sites and reduce CO2 transport distances needed to deliver negative emissions through BECCS. Importantly, distributed sources of biogenic CO2 will also need the development of biomass feedstock transport networks to suitable bio-energy facilities. We posit that siting new CO2 storage sites within continental and Southern Europe will reduce necessary CO2 transportation distances and ensuing environmental and economic costs.
While, our analysis accounts for the technical biogenic CDR potential in different European countries, most of the assessed potentials are unlikely to be deployed for various economic, social, and political reasons. Since BECCS deployment requires a high degree of customization and has a high design complexity,73 site-specific economic-, social-, environmental-, technical, and policy-related factors will combine to influence the deployment at scale of BECCS resources in Europe in the coming years.74,75 Successful BECCS deployment will likely rely on some form of government support and new institutional arrangements to overcome barriers associated with early development – including accounting and rewarding for negative emissions.22,76,77 Importantly, site-specific life cycle assessments are needed to determine whether the biogenic CDR potentials estimated in this study will be able to deliver negative emissions.66 By investigating where and what BECCS configurations could be deployed, this work helps to identify the role that biogenic CDR could have to sustainably deliver negative emissions in Europe.
Data availability
Spatially-explicit carbon dioxide removal potentials (Fig. 2 and 3) are available at: https://doi.org/10.5281/zenodo.4441678.
Author contributions
L. R. and M. M. conceived and designed the study; L. R. led data analysis, data collection, and writing; D. S. assisted with research design, data collection. M. M., D. S. assisted with writing.
Conflicts of interest
The authors declare no conflict of interest.
Acknowledgements
L. R. and M. M. thank the partners of the PATHFNDR consortium (a project starting in Spring 2021 and sponsored by the Swiss Federal Office of Energy's SWEET programme) for the valuable discussions.
References
- European Commission, 2018. 2050 long-term strategy. In-depth analysis in support of the commission communication COM(2018) 773. A Clean Planet for all. A European long-term strategic vision for a prosperous, modern, competitive and climate neutral economy. Available at: https://ec.europa.eu/clima/policies/strategies/2050_en. Accessed January 2021.
- V. Scott and O. Geden, The challenge of carbon dioxide removal for EU policy-making, Nat. Energy, 2018, 3(5), 350–352 CrossRef CAS.
- O. Geden, G. P. Peters and V. Scott, Targeting carbon dioxide removal in the European Union, Clim. Policy, 2019, 19(4), 487–494 CrossRef.
- C. Pozo, Á. Galán-Martín, D. M. Reiner, N. Mac Dowell and G. Guillén-Gosálbez, Equity in allocating carbon dioxide removal quotas, Nat. Clim. Change, 2020, 1–7 Search PubMed.
- C. Azar, D. J. Johansson and N. Mattsson, Meeting global temperature targets—the role of bioenergy with carbon capture and storage, Environ. Res. Lett., 2013, 8(3), 034004 CrossRef CAS.
- S. Fuss, J. G. Canadell, G. P. Peters, M. Tavoni, R. M. Andrew, P. Ciais, R. B. Jackson, C. D. Jones, F. Kraxner, N. Nakicenovic and C. Le Quéré, Betting on negative emissions, Nat. Clim. Change, 2014, 4(10), 850–853 CrossRef CAS.
- B. Solano Rodriguez, P. Drummond and P. Ekins, Decarbonizing the EU energy system by 2050: an important role for BECCS, Clim. Policy, 2017, 17(supp. 1), S93–S110 CrossRef.
- J. C. Minx, W. F. Lamb, M. W. Callaghan, S. Fuss, J. Hilaire, F. Creutzig, T. Amann, T. Beringer, W. de Oliveira Garcia, J. Hartmann and T. Khanna, Negative emissions—Part 1: Research landscape and synthesis, Environ. Res. Lett., 2018, 13(6), 063001 CrossRef.
- G. Luderer, Z. Vrontisi, C. Bertram, O. Y. Edelenbosch, R. C. Pietzcker, J. Rogelj, H. S. De Boer, L. Drouet, J. Emmerling, O. Fricko and S. Fujimori, Residual fossil CO 2 emissions in 1.5–2 C pathways, Nat. Clim. Change, 2018, 8(7), 626–633 CrossRef CAS.
-
J. Rogelj, et al., in Global Warming of 1.5 °C, ed. V. Masson-Delmotte, et al., IPCC, 2019, pp. 93–174 Search PubMed.
- G. Realmonte, L. Drouet, A. Gambhir, J. Glynn, A. Hawkes, A. C. Köberle and M. Tavoni, An inter-model assessment of the role of direct air capture in deep mitigation pathways, Nat. Commun., 2019, 10(1), 1–12 CrossRef PubMed.
- F. Schreyer, G. Luderer, R. Rodrigues, R. C. Pietzcker, L. Baumstark, M. Sugiyama, R. J. Brecha and F. Ueckerdt, Common but differentiated leadership: strategies and challenges for carbon neutrality by 2050 across industrialized economies, Environ. Res. Lett., 2020, 15(11), 114016 CrossRef.
- G. P. Peters and O. Geden, Catalysing a political shift from low to negative carbon, Nat. Clim. Change, 2017, 7(9), 619–621 CrossRef.
- J. Kemper, Biomass and carbon dioxide capture and storage: A review, Int. J. Greenhouse Gas Control, 2015, 40, 401–430 CrossRef CAS.
- D. L. Sanchez, N. Johnson, S. T. McCoy, P. A. Turner and K. J. Mach, Near-term deployment of carbon capture and sequestration from biorefineries in the United States, Proc. Natl. Acad. Sci. U. S. A., 2018, 115(19), 4875–4880 CrossRef CAS PubMed.
- M. Bui,
et al., Carbon capture and storage (CCS): the way forward, Energy Environ. Sci., 2018, 11(5), 1062–1176 RSC.
- C. Hepburn, E. Adlen, J. Beddington, E. A. Carter, S. Fuss, N. Mac Dowell, J. C. Minx, P. Smith and C. K. Williams, The technological and economic prospects for CO 2 utilization and removal, Nature, 2019, 575(7781), 87–97 CrossRef CAS PubMed.
- D. L. Sanchez, J. H. Nelson, J. Johnston, A. Mileva and D. M. Kammen, Biomass enables the transition to a carbon-negative power system across western North America, Nat. Clim. Change, 2015, 5(3), 230–234 CrossRef CAS.
- M. Fajardy and N. Mac Dowell, Can BECCS deliver sustainable and resource efficient negative emissions?, Energy Environ. Sci., 2017, 10(6), 1389–1426 RSC.
- M. Fajardy, S. Chiquier and N. Mac Dowell, Investigating the BECCS resource nexus: delivering sustainable negative emissions, Energy Environ. Sci., 2018, 11(12), 3408–3430 RSC.
- S. V. Hanssen, V. Daioglou, Z. J. N. Steinmann, J. C. Doelman, D. P. Van Vuuren and M. A. J. Huijbregts, The climate change mitigation potential of bioenergy with carbon capture and storage, Nat. Clim. Change, 2020, 10(11), 1023–1029 CrossRef CAS.
-
D. Sandalow, R. Aines, J. Friedmann, C. McCormick and D. Sanchez, Biomass carbon removal and storage (BiCRS) roadmap, ICEF, 2021. Available at: https://www.icef-forum.org/roadmap/.
- N. Scarlat, F. Fahl and J. F. Dallemand, Status and opportunities for energy recovery from municipal solid waste in Europe, Waste Biomass Valorization, 2019, 10(9), 2425–2444 CrossRef.
- N. Scarlat, F. Fahl, J. F. Dallemand, F. Monforti and V. Motola, A spatial analysis of biogas potential from manure in Europe, Renewable Sustainable Energy Rev., 2018, 94, 915–930 CrossRef.
- N. Scarlat, F. Fahl, E. Lugato, F. Monforti-Ferrario and J. F. Dallemand, Integrated and spatially explicit assessment of sustainable crop residues potential in Europe, Biomass Bioenergy, 2019, 122, 257–269 CrossRef.
- P. Smith, S. J. Davis, F. Creutzig, S. Fuss, J. Minx, B. Gabrielle, E. Kato, R. B. Jackson, A. Cowie, E. Kriegler and D. P. Van Vuuren, Biophysical and economic limits to negative CO2 emissions, Nat. Clim. Change, 2016, 6(1), 42–50 CrossRef CAS.
- V. Heck, D. Gerten, W. Lucht and A. Popp, Biomass-based negative emissions difficult to reconcile with planetary boundaries, Nat. Clim. Change, 2018, 8(2), 151–155 CrossRef CAS.
- S. Fuss, W. F. Lamb, M. W. Callaghan, J. Hilaire, F. Creutzig, T. Amann, T. Beringer, W. de Oliveira Garcia, J. Hartmann, T. Khanna and G. Luderer, Negative emissions—Part 2: Costs, potentials and side effects, Environ. Res. Lett., 2018, 13(6), 063002 CrossRef.
- P. A. Turner, C. B. Field, D. B. Lobell, D. L. Sanchez and K. J. Mach, Unprecedented rates of land-use transformation in modelled climate change mitigation pathways, Nat. Sustain., 2018, 1(5), 240–245 CrossRef.
- S. Roe, C. Streck, M. Obersteiner, S. Frank, B. Griscom, L. Drouet, O. Fricko, M. Gusti, N. Harris, T. Hasegawa and Z. Hausfather, Contribution of the land sector to a 1.5 C world, Nat. Clim. Change, 2019, 1–12 Search PubMed.
- J. Fuhrman, H. McJeon, P. Patel, S. C. Doney, W. M. Shobe and A. F. Clarens, Food-energy-water implications of negative emissions technologies in a +1.5 °C future, Nat. Clim. Change, 2020, 10(10), 920–927 CrossRef CAS.
- M. Muratori, K. Calvin, M. Wise, P. Kyle and J. Edmonds, Global economic consequences of deploying bioenergy with carbon capture and storage (BECCS), Environ. Res. Lett., 2016, 11(9), 095004 CrossRef.
- J. A. Rehbein, J. E. Watson, J. L. Lane, L. J. Sonter, O. Venter, S. C. Atkinson and J. R. Allan, Renewable energy development threatens many globally important biodiversity areas, Global Change Biol., 2020, 26(5), 3040–3051 CrossRef PubMed.
- L. Rosa, J. A. Reimer, M. S. Went and P. D’Odorico, Hydrological limits to carbon capture and storage, Nat. Sustain., 2020, 1–9 Search PubMed.
- L. Rosa, D. L. Sanchez, G. Realmonte, D. Baldocchi and P. D'Odorico, The water footprint of carbon capture and storage technologies, Renewable Sustainable Energy Rev., 2020, 110511 Search PubMed.
- G. Ceccherini, G. Duveiller, G. Grassi, G. Lemoine, V. Avitabile, R. Pilli and A. Cescatti, Abrupt increase in harvested forest area over Europe after 2015, Nature, 2020, 583(7814), 72–77 CrossRef CAS PubMed.
- R. Fuchs, C. Brown and M. Rounsevell, Europe’s Green Deal offshores environmental damage to other nations, Nature, 2020, 586, 671–673 CrossRef CAS PubMed.
- T. D. Searchinger, T. Beringer, B. Holtsmark, D. M. Kammen, E. F. Lambin, W. Lucht, P. Raven and J. P. van Ypersele, Europe's renewable energy directive poised to harm global forests, Nat. Commun., 2018, 9(1), 1–4 CrossRef CAS PubMed.
- M. Norton, A. Baldi, V. Buda, B. Carli, P. Cudlin, M. B. Jones, A. Korhola, R. Michalski, F. Novo, J. Oszlányi and F. D. Santos, Serious mismatches continue between science and policy in forest bioenergy, GCB Bioenergy, 2019, 11(11), 1256–1263 CrossRef.
- N. Bauer, C. Bertram, A. Schultes, D. Klein, G. Luderer, E. Kriegler, A. Popp and O. Edenhofer, Quantification of an efficiency–sovereignty trade-off in climate policy, Nature, 2020, 588(7837), 261–266 CrossRef CAS PubMed.
- A. Beltran-Peña, L. Rosa and P. D’Odorico, Global food self-sufficiency in the 21st century under sustainable intensification of agriculture, Environ. Res. Lett., 2020, 15(9), 095004 CrossRef.
- K. L. Kline, S. Msangi, V. H. Dale, J. Woods, G. M. Souza, P. Osseweijer, J. S. Clancy, J. A. Hilbert, F. X. Johnson, P. C. McDonnell and H. K. Mugera, Reconciling food security and bioenergy: priorities for action, GCB Bioenergy, 2017, 9(3), 557–576 CrossRef.
- J. S. Næss, O. Cavalett and F. Cherubini, The land–energy–water nexus of global bioenergy potentials from abandoned cropland, Nat. Sustain., 2021, 1–12 Search PubMed.
- D. L. Sanchez and D. M. Kammen, A commercialization strategy for carbon-negative energy, Nat. Energy, 2016, 1(1), 1–4 Search PubMed.
-
S. E. Baker, G. Peridas, J. K. Stolaroff, H. M. Goldstein, S. H. Pang, F. R. Lucci, W. Li, E. W. Slessarev, J. Pett-Ridge, F. R. Ryerson and R. D. Aines, Getting to neutral: options for negative carbon emissions in California (No. LLNL-TR-796100), Lawrence Livermore National Lab. (LLNL), Livermore, CA (United States), 2019. Available at: https://www-gs.llnl.gov/content/assets/docs/energy/Getting_to_Neutral.pdf.
- K. Onarheim, S. Santos, P. Kangas and V. Hankalin, Performance and costs of CCS in the pulp and paper industry part 1: Performance of amine-based post-combustion CO2 capture, Int. J. Greenhouse Gas Control, 2017, 59, 58–73 CrossRef CAS.
- W. J. Sagues, H. Jameel, D. L. Sanchez and S. Park, Prospects for
bioenergy with carbon capture & storage (BECCS) in the United States pulp and paper industry, Energy Environ. Sci., 2020, 13(8), 2243–2261 RSC.
- N. Pour, P. A. Webley and P. J. Cook, Potential for using municipal solid waste as a resource for bioenergy with carbon capture and storage (BECCS), Int. J. Greenhouse Gas Control, 2018, 68, 1–15 CrossRef CAS.
- M. Bui, M. Fajardy and N. Mac Dowell, Bio-energy with carbon capture and storage (BECCS): Opportunities for performance improvement, Fuel, 2018, 213, 164–175 CrossRef CAS.
- R. Slade, A. Bauen and R. Gross, Global bioenergy resources, Nat. Clim. Change, 2014, 4(2), 99–105 CrossRef.
- R. Bellamy and O. Geden, Govern CO2 removal from the ground up, Nat. Geosci., 2019, 12(11), 874–876 CrossRef CAS.
- G. F. Nemet, M. W. Callaghan, F. Creutzig, S. Fuss, J. Hartmann, J. Hilaire, W. F. Lamb, J. C. Minx, S. Rogers and P. Smith, Negative emissions—Part 3: Innovation and upscaling, Environ. Res. Lett., 2018, 13(6), 063003 CrossRef.
- European Environmental Agency and Directorate-General for Environment. Waterbase – UWWTD: Urban Waste Water Treatment Directive – reported data. Available at: https://www.eea.europa.eu/data-and-maps/data/waterbase-uwwtd-urban-waste-water-treatment-directive-7. Accessed October 2020.
- European Environmental Agency. Industrial Reporting under the Industrial Emissions Directive 2010/75/EU and European Pollutant Release and Transfer Register Regulation (EC) No 166/2006. Available at: https://www.eea.europa.eu/data-and-maps/data/industrial-reporting-under-the-industrial-1. Accessed October 2020.
- J. Mohn, S. Szidat, K. Zeyer and L. Emmenegger, Fossil and biogenic CO2 from waste incineration based on a yearlong radiocarbon study, Waste Manage., 2012, 32(8), 1516–1520 CrossRef CAS PubMed.
- K. Fuglsang, N. H. Pedersen, A. W. Larsen and T. F. Astrup, Long-term sampling of CO2 from waste-to-energy plants: 14C determination methodology, data variation and uncertainty, Waste Manage. Res., 2014, 32(2), 115–123 CrossRef CAS PubMed.
- T. Schwarzböck, H. Rechberger, O. Cencic and J. Fellner, Determining national greenhouse gas emissions from waste-to-energy using the Balance Method, Waste Manage., 2016, 49, 263–271 CrossRef PubMed.
- Y. Law, G. E. Jacobsen, A. M. Smith, Z. Yuan and P. Lant, Fossil organic carbon in wastewater and its fate in treatment plants, Water Res., 2013, 47(14), 5270–5281 CrossRef CAS PubMed.
- D. R. Griffith, R. T. Barnes and P. A. Raymond, Inputs of fossil carbon from wastewater treatment plants to US rivers and oceans, Environ. Sci. Technol., 2009, 43(15), 5647–5651 CrossRef CAS PubMed.
-
N. Bachmann, J. la Cour, G. Bochmann and N. Montpart, Sustainable biogas production in municipal wastewater treatment plants, IEA Bioenergy, Massongex, Switzerland, 2015 Search PubMed.
- Y. Li, R. Zhang, G. Liu, C. Chen, Y. He and X. Liu, Comparison of methane production potential, biodegradability, and kinetics of different organic substrates, Bioresour. Technol., 2013, 149, 565–569 CrossRef CAS PubMed.
- P. Brandl, M. Bui, J. P. Hallett and N. Mac Dowell, Beyond 90% capture: Possible, but at what cost?, Int. J. Greenhouse Gas Control, 2021, 105, 103239 CrossRef CAS.
- F. Bauer, T. Persson, C. Hulteberg and D. Tamm, Biogas upgrading–technology overview, comparison and perspectives for the future, Biofuels, Bioprod. Biorefin., 2013, 7(5), 499–511 CrossRef CAS.
- R. A. Alvarez, D. Zavala-Araiza, D. R. Lyon, D. T. Allen, Z. R. Barkley, A. R. Brandt, K. J. Davis, S. C. Herndon, D. J. Jacob, A. Karion and E. A. Kort, Assessment of methane emissions from the US oil and gas supply chain, Science, 2018, 361(6398), 186–188 CAS.
- A. R. Brandt, G. A. Heath, E. A. Kort, F. O'Sullivan, G. Pétron, S. M. Jordaan, P. Tans, J. Wilcox, A. M. Gopstein, D. Arent and S. Wofsy, Methane leaks from North American natural gas systems, Science, 2014, 343(6172), 733–735 CrossRef CAS PubMed.
- S. E. Tanzer and A. Ramirez, When are negative emissions negative emissions?, Energy Environ. Sci., 2019, 12(4), 1210–1218 RSC.
- T. Terlouw, C. Bauer, L. Rosa and M. Mazzotti, Life cycle assessment of carbon dioxide removal technologies: A critical review, Energy Environ. Sci., 2021 10.1039/d0ee03757e.
- Swiss Federal Office of Energy, 2020. Energy perspectives 2050. Available at: https://www.bfe.admin.ch/bfe/en/home/policy/energy-strategy-2050/documentation/energy-perspectives-2050.html. Accessed January 2021.
-
O. Geden and F. Schenuit, Unconventional Mitigation: Carbon Dioxide Removal as a New Approach in EU Climate Policy, German Institute for International and Security Affairs, Berlin, 2020.
- C. L. Fyson, S. Baur, M. Gidden and C. F. Schleussner, Fair-share carbon dioxide removal increases major emitter responsibility, Nat. Clim. Change, 2020, 10(9), 836–841 CrossRef CAS.
- V. Daioglou, M. Muratori, P. Lamers, S. Fujimori, A. Kitous, A. C. Köberle, N. Bauer, M. Junginger, E. Kato, F. Leblanc and S. Mima, Implications of climate change mitigation strategies on international bioenergy trade, Clim. Change, 2020, 163(3), 1639–1658 CrossRef.
- Global CCS Institute, Global Status of CCS Report 2020, Melbourne, Australia, 2020.
- A. Malhotra and T. S. Schmidt, Accelerating Low-Carbon Innovation, Joule, 2020, 4(11), 2259–2267 CrossRef CAS.
- M. Fridahl and M. Lehtveer, Bioenergy with carbon capture and storage (BECCS): Global potential, investment preferences, and deployment barriers, Energy Res. Soc. Sci., 2018, 42, 155–165 CrossRef.
- R. Bellamy, J. Lezaun and J. Palmer, Perceptions of bioenergy with carbon capture and storage in different policy scenarios, Nat. Commun., 2019, 10(1), 1–9 CrossRef PubMed.
- C. Gough, S. Garcia-Freites, C. Jones, S. Mander, B. Moore, C. Pereira, M. Röder, N. Vaughan and A. Welfle, Challenges to the use of BECCS as a keystone technology in pursuit of 1.5 °C, Global Sustain., 2018, 1, E5 CrossRef.
- A. Torvanger, Governance of bioenergy with carbon capture and storage (BECCS): accounting, rewarding, and the Paris agreement, Clim. Policy, 2019, 19(3), 329–341 CrossRef.
Footnote |
† Electronic supplementary information (ESI) available. See DOI: 10.1039/d1ee00642h |
|
This journal is © The Royal Society of Chemistry 2021 |