DOI:
10.1039/D1DT02790E
(Paper)
Dalton Trans., 2021,
50, 15140-15152
Lead calix[n]arenes (n = 4, 6, 8): structures and ring opening homo-/co-polymerization capability for cyclic esters†
Received
20th August 2021
, Accepted 30th September 2021
First published on 30th September 2021
Abstract
Reaction of [LiPb(OiPr)3]2 (generated in situ) with either p-tert-butylcalix[4]areneH4 (L4H4) or p-tert-butylcalix[6]areneH6 (L6H6) resulted in the heterometallic lithium/lead complexes [Pb4Li2(L4)4H6(MeCN)3]·4.5MeCN (1·4.5MeCN) and [Pb8Li10Cl2(L6H2)3(L6)(OH)2(O)2(H2O)2(MeCN)4]·14MeCN (2·14MeCN), respectively. Use of the dimethyleneoxa-bridged p-tert-butyltetrahomodioxacalix[6]areneH6 (L6′H6) with five equivalents of [Pb(OiPr)2] afforded [Pb13(L6′)3O4(iPrOH)]·11MeCN (3·11MeCN). Use of the larger p-tert-butylcalix[8]areneH8 (L8H8) with [Pb(OtBu)2] or {Pb[N(TMS)2]} (TMS = SiMe3) afforded the products [Pb12(L8)2O4]·8.7C7H8 (4·8.7C7H8) or [Pb6(SiMe3)2(L8)O2Cl2] (5), respectively. Reaction of {Pb[N(TMS)2]} (generated in situ from (Me3Si)2NH, nBuLi and PbCl2) with L6H6 afforded, after work-up (MeCN), the mixed-metal complex [Pb10Li2(L6)2(OH)Cl(O)4]·9.5MeCN (6·9.5MeCN). Reaction of distilled {Pb[N(TMS)2]} (six equivalents) with L8H8 resulted in the complex [Pb12(L8)2O4]·12MeCN (7·12MeCN). Complexes 1–7, Pb(OiPr)2 and [Pb(N(TMS)2)2] have been screened for their potential to act as pre-catalysts in the ring opening polymerization (ROP) of ε-caprolactone (ε-CL) and δ-valerolactone (δ-VL) and the copolymerization thereof. Generally, the lithiated complexes 1 and 2 exhibited better activities than the other pre-catalysts screened herein. For ε-CL and δ-VL, moderate activity at 130 °C over 24 h was observed for 1–7. In the case of the co-polymerization of ε-CL with δ-VL, 1–7, Pb(OiPr)2 and [Pb(N(TMS)2)2] afforded reasonable conversions and high molecular weight polymers. The systems 1–7, Pb(OiPr)2 and [Pb(N(TMS)2)2] also proved to be active in the ROP of the rac-lactide (r-LA); the activity trend was found to be 1 > 2 ≈ Pb(OiPr)2 ≈ [Pb(N(TMS)2)2] > 4 > 5 ≈ 6 ≈ 7 > 3.
Introduction
The ring opening polymerization (ROP) of cyclic esters is attracting much attention in light of the current environmental issues with petroleum-derived plastics.1 As seen in previous work, coordination chemistry can play a crucial role in the development of new greener polymers with desirable features, given that control over the ligands at the metal centre of the catalyst employed for ROP can allow for control over the resultant polymer properties.2 Ideally, the metal centre needs to be highly active, as well as abundant and non-toxic, however sometimes it is necessary for one of these criteria to become secondary if one or more of the others is exemplary. With this in mind, we note the elegant work by Sarazin et al. reporting that for main group elements of group IV, systems employing the ligand set 2-CH2NR2-4,6-tBu2-C6H2OH, {LOi}H, (i = 1, NR2 = N((CH2)2OCH3)2; i = 2, NR2 = NEt2; i = 3, NR2 = aza-15-crown-5) were ROP active. The ROP activity followed the trend GeII ≪ SnII ≪ PbII,3 whilst for a subsequent report on [M(μ2-OiPr)2]n, the trend was Ge ≪ Sn < Pb.4 Thus, despite the issue of toxicity associated with lead compounds, and given our interest in metallocalix[n]arenes as ROP catalysts,5 combined with a lack of lead calixarenes,6 this work has utilized the calix[n]arenes shown in Chart 1, which differ in both size (n = 4, 6, 8) and/or bridge (–CH2– or –CH2OCH2–), to access new lead-containing calix[n]arene complexes. We note that a number of lead complexes derived from carboxylated calixarenes have been reported.7 Moreover, the coordination chemistry of calix[n]arenes, where n ≥ 6, is somewhat limited.8 A number of interesting multi-metallic species (see Chart 2) have been structurally characterized. The ability of these complexes to act as catalysts for the ROP of ε-caprolactone (ε-CL), δ-valerolactone (δ-VL) and rac-lactide (r-LA) has been investigated; the copolymerization of ε-CL and δ-VL was also investigated.
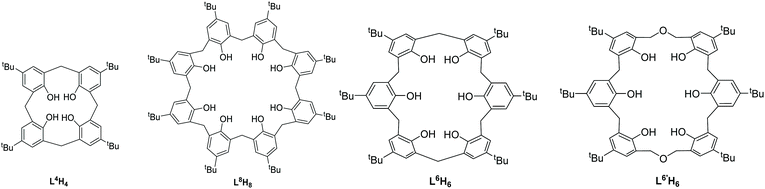 |
| Chart 1 The calixarenes employed herein. | |
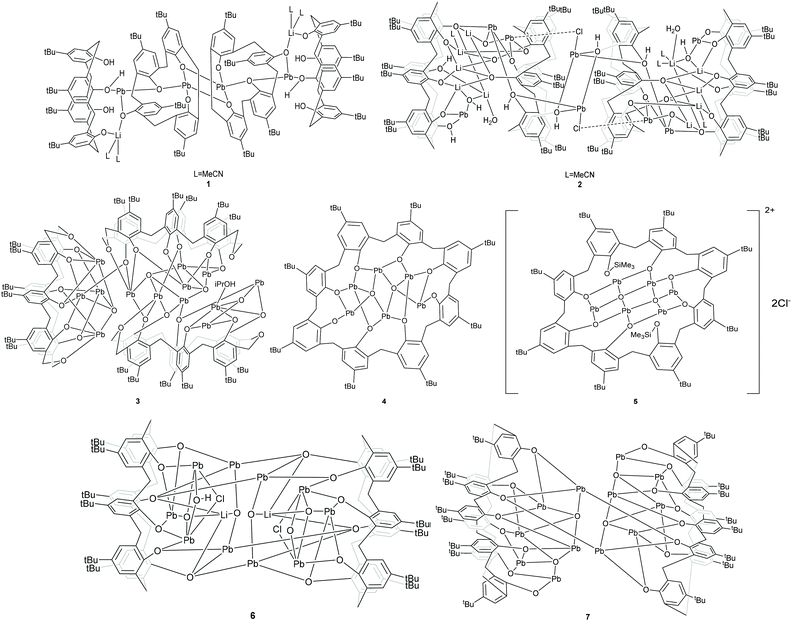 |
| Chart 2 Lead calix[n]arenes reported herein. | |
Results and discussion
Syntheses and solid-state structures
Given that we have previously found the metallocalix[n]arenes generated from in situ heterobimetallic reagents of the form Li[M(OR)x] (M = Cr, Fe) tend to be highly crystalline,9 we selected here as our entry point the reagent [LiPb(OiPr)3]2, which was prepared by the addition of LiOiPr to in situ generated [Pb(OiPr)2] (from PbCl2, (TMS)2NH, nBuLi and iPrOH; TMS = trimethylsilyl). We note that the Sarazin et al. preparation of [LiPb(μ2-OtBu)3(μ3-OtBu)]2 initially isolates [Pb(μ2-OtBu)2]3 prior to the addition of LiOtBu.4 Subsequent reaction of our in situ generated precursor with one equivalent of p-tert-butylcalix[4]areneH4 (L4H4) in refluxing toluene led, following work-up (extraction into acetonitrile), to the complex [Pb4Li2(L4)4H6(MeCN)3]·4.5MeCN (1·4.5MeCN) in moderate yield (37%). The molecular structure of 1·4.5MeCN is shown in Fig. 1, with selected bond lengths and angles given in the caption. For crystallographic data, see Table S1 (ESI†). The cluster is centrosymmetric and contains four Pb atoms and two lithiums. At the centre of the cluster are two Pb atoms (Pb2) and two oxygen atoms (O2) from a calix[4]arene in a diamond formation (Fig. 1 bottom). On either side of this lie calix[4]arene molecules that are twisted so that one of the aromatic rings points downwards from the bowl of the calixarene; this enables it to bind Pb2 on one side and Pb1 on the other. There is a further calix[4]arene at each end of the cluster (in bowl configuration) and this forms one O–Pb bond to Pb1. The Pb–O bond lengths [2.235(5)–2.528(6) Å] are somewhat longer than those found in the thiacalix[4]arene (L4S) complexes {Pb[L4S(Obenzyl)2]} (2.149(2) Å) and {Pb[L4S(OTMS)2]} (2.1632(18)/2.1891(19) Å),6b and in the aryloxides [(BDI)Pb(Ar)] (2.182(4)/2.212(2) Å; Ar = 2,6-tBu-4-MeC6H2, BDI = [{N(2,6-iPr2C6H3)C(Me)}2CH]) and [Pb(OAr)2] (2.211(2)/2.228(2) Å; Ar = C6H3-2.6-(C6H3-2,6-iPr2).10b The longer bond lengths in 1 and in the other structures herein reflect the bridging modes adopted by the Pb centres. The lithium ions are coordinated by one oxygen from each of the two calixarenes; coordination about the Li is completed by MeCN. In essence, the complex can be described as a cluster of four stacked calix[4]arene molecules threaded by an LiOPbOPb(μ2-O)PbOPbOLi chain.
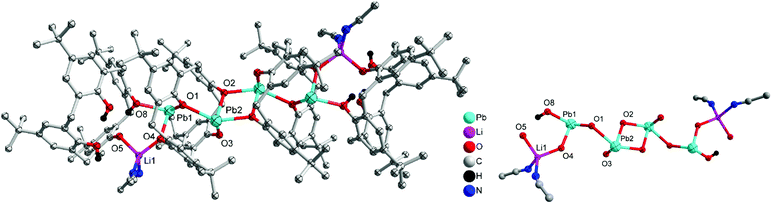 |
| Fig. 1 Molecular structure and core of [Pb4Li2(L4)4H6(MeCN)3]·4.5MeCN (1·4.5MeCN). Non coordinated solvent molecules have been removed for clarity. Selected bond lengths (Å) and angles (°): Pb(1)–O(1) 2.235(5), Pb(1)–O(4) 2.260(6), Pb(1)–O(8) 2.357(6), Pb(2)–O(1) 2.528(6), Pb(2)–O(2) 2.283(6), O(1)–Pb(1)–O(4) 82.7(2), O(1)–Pb(1)–O8 101.3(2), O(4)–Pb(1)–O(8) 81.5(2), O(2)–Pb(2)–O(1) 77.74(19), O(3)–Pb(2)–O(1) 98.7(2), O(4)–Li(1)–O(5) 102.4(8), O(4)–Li(1)–N(2) 126.5(11), O(4)–Li(1)–N(1) 102.9(10), O(5)–Li(1)–N(1) 121.2(12). | |
Using a similar method to 1, but using p-tert-butylcalix[6]areneH6 (L6H6) led to the isolation of the complex [Pb8Li10Cl2(L6H2)3(L6)(OH)2(O)2(H2O)2(MeCN)4]·14MeCN (2·14MeCN) in moderate yield (47%). The molecular structure of 2·14MeCN is shown in Fig. 2, with selected bond lengths and angles given in the caption. The cluster is reasonably close to being centrosymmetric and is composed of four calixarenes that bind 8 lead ions and 10 lithium ions. At the centre of the cluster there is a Pb2O2 diamond which is linked to further lead atoms via bridging chloride ions. Two further Pb ions are bound near the plane of the six oxygen atoms by each calixarene adjacent to the centre. One more Pb is bound on each side by the terminal calixarenes, which also binds 5 lithium ions. There are additional oxide and hydroxide anions between the Pb and Li ions and bound acetonitrile. The presence of the oxo ligands is thought to be due to adventitious hydrolysis.
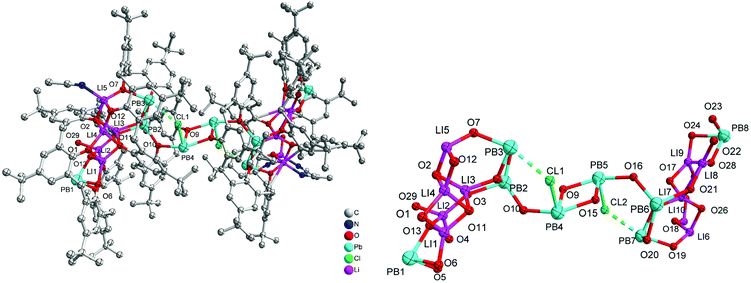 |
| Fig. 2 Molecular structure and core of [Pb8Li10Cl2(L6H2)3(L6)(OH)2(O)2(H2O)2(MeCN)4]·14MeCN (2·14MeCN). Selected bond lengths (Å) and angles (°): O(1)–Li(1) 2.063(16), O(1)–Li(4) 1.964(17), O(2)–Li(3) 1.978(15), O(2)–Li(4) 1.960(17), O(2)–Li(5) 1.943(16), O(3)–Pb(2) 2.496(6), O(3)–Li(2) 2.064(19), O(5)–Pb(1) 2.266(6), O(6)–Pb(1) 2.288(6), O(7)–Pb(3) 2.318(5), O(8)–Pb(3) 2.313(6), O(9)–Pb(4) 2.280(6), O(9)–Pb(5) 2.467(6), O(10)–Pb(2) 2.342(6), O(10)–Pb(4) 2.390(6), Li(4)–O(2)–Li(3) 81.2(6), Li(5)–O(2)–Li(3) 105.2(6), Li(5)–O(2)–Li(4) 83.2(7), Li(2)–O(3)–Pb(2) 157.1(6), Li(3)–O(3)–Pb(2) 93.9(5), Li(3)–O(3)–Li(2) 95.1(7), Pb(3)–O(8)–Pb(2) 104.7(2), Li(5)–O(7)–Pb(3) 122.3(5). | |
Use of the precursors [Pb(OR)2] (R = iPr or tBu) or {Pb[N(TMS)2]} also allows access to metallocalix[n]arene species. Indeed, interaction of five equivalents of [Pb(OiPr)2] with p-tert-butyltetrahomodioxacalix[6]areneH6 (L6′H6) afforded, following work-up, the complex [Pb13(L6′)3O4(iPrOH)]·11MeCN (3·11MeCN). We note that use of dioxamethylene-bridged calixarenes can be beneficial for certain polymerization processes.11 The molecular structure of 3·11MeCN is shown in Fig. 3, with selected bond lengths and angles given in the caption. The complex is a large cluster of 13 unique Pb atoms composed of two smaller clusters. A pair of calixarenes are arranged roughly on top of each other. Between these lie nine lead atoms (Pb5–Pb13), and four oxide anions (O26–O29). Each lead is coordinated by the oxygen atoms of the calixarene and the oxide. Uniquely, the coordination of Pb11 is completed by an isopropoxide. The second part of the cluster is approximately perpendicular to the first and linked to it through the bond Pb2–O9. This contains four lead atoms (Pb1–Pb4) which surround a central oxide (O25). Around the cluster there are 11 unbound molecules of MeCN, some of which are ordered, others located by SQUEEZE.
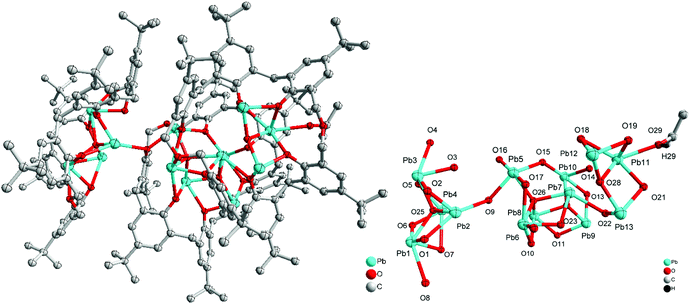 |
| Fig. 3 Molecular structure and core of [Pb13(L6′)3O4(iPrOH)]·11MeCN (3·11MeCN). Selected bond lengths (Å) and angles (°): Pb(1)–Pb(2) 3.6746(6), Pb(1)–O(1) 2.356(8), Pb(1)–O(6) 2.553(8), Pb(1)–O(7) 2.359(8), Pb(2)–Pb(3) 3.5012(7), Pb(2)–O(1) 2.405(8), Pb(2)–O(2) 2.224(9), Pb(2)–O(9) 2.738(8), Pb(3)–O(2) 2.483(8), Pb(3)–O(3) 2.340(9), Pb(3)–O(4) 2.757(9), Pb(3)–O(5) 2.365(9), Pb(4)–O(5) 2.379(10), Pb(4)–O(6) 2.208(8), Pb(4)–O(7) 2.751(8), Pb(5)–O(9) 2.447(7), Pb(5)–O(15) 2.503(8), Pb(5)–O(16) 2.586(7), Pb(6)–O(17) 2.581(7), Pb(6)–O(23) 2.251(7), Pb(7)–Pb(10) 3.5418(6), O(1)–Pb(1)–Pb(2) 39.97(19), O(1)–Pb(1)–Pb(4) 108.51(19), O(1)–Pb(1)–O(6) 145.6(2), O(1)–Pb(1)–O(7) 106.6(3), Pb(5)–O(9)–Pb(2) 118.6(3), O(1)–Pb(2)–Pb(1) 38.99(19), O(1)–Pb(2)–O(9) 149.7(3), O(2)–Pb(2)–O(1) 76.6(3), O(2)–Pb(2)–O(9) 128.1(3), O(3)–Pb(3)–O(2) 68.7(3), O(5)–Pb(3)–O(4) 70.5(3), O(5)–Pb(4)–O(7) 132.0(3), O(9)–Pb(5)–O(15) 127.1(3). | |
Similar use of [Pb(OtBu)2], but with p-tert-butylcalix[8]areneH8 (L8H8) afforded the colourless dimer [Pb12(L8)2O4]·8.7C7H8 (4·8.7C7H8). The molecular structure of half of the dimer of 4·8.7MeCN is shown in Fig. 4, with selected bond lengths and angles given in the caption. In each half dimer, a hexanuclear lead cluster (Fig. 4, right) sits on the open face of an L8 bowl. The μ4-oxo centres are thought to arise via the presence of adventitious hydrolysis. Two Pb–aryl interactions present result in the formation of the observed dimers (see Fig. S1, ESI†). In solution, we only see (by 207Pb NMR spectroscopy) two distinct lead environments (see Experimental section).
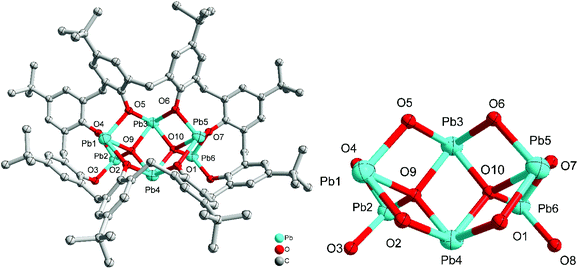 |
| Fig. 4 Molecular structure and core of [Pb12(L8)2O4]·8.7C7H8 (4·8.7C7H8). Selected bond lengths (Å) and angles (°): Pb(1)–O(9) 2.297(4), Pb(1)–O(5) 2.336(5), Pb(1)–O(2) 2.419(5), Pb(1)–O(4) 2.432(5), Pb(2)–O(3) 2.206(4), Pb(2)–O(9) 2.268(5), Pb(2)–O(4) 2.287(4), Pb(3)–O(5) 2.258(4), Pb(3)–O(10) 2.317(4), Pb(3)–O(6) 2.341(4), Pb(3)–O(9) 2.350(4), O(9)–Pb(1)–O(2) 69.91(15), O(5)–Pb(1)–O(2) 101.56(17), O(9)–Pb(1)–O(4) 68.63(16), O(3)–Pb(2)–O(9) 91.99(16), O(3)–Pb(2)–O(4) 90.09(17), O(9)–Pb(2)–O(4) 71.73(16), O(5)–Pb(3)–O(6) 79.54(16), O(10)–Pb(3)–O(6) 71.26(15), O(5)–Pb(3)–O(9) 73.37(16). | |
The same calix[8]arene, namely L8H8, on interaction with {Pb[N(TMS)2]} (TMS = SiMe3) afforded after, work-up (MeCN), the product [Pb6(SiMe3)2(L8)O2Cl2] (5). The complex is a centrosymmetric Pb cluster that contains a single calix[8]arene. The asymmetric unit contains one half of the calixarene, three Pb ions and one oxide. The molecular structure of 5 is shown in Fig. 5, with selected bond lengths and angles given in the caption. Two of the phenols of the calixarene on opposite sides of the ring carry SiMe3 groups and do not bind to a metal. At the centre of the ring there is a cluster with composition Pb6O2 that is formed from two edge-sharing OPb4 tetrahedra (oxide surrounded by four Pb ions). The coordination about the Pb ions is completed by the oxygen atoms of the calixarene. Pb1 sits at the apex of a square pyramid; the other unique Pb ions are three coordinate.
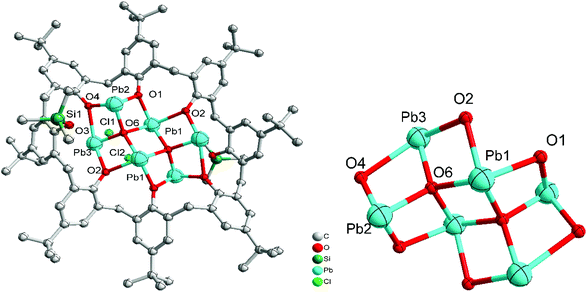 |
| Fig. 5 Molecular structure and core of [Pb6(SiMe3)2(L8)O2Cl2] (5). Selected bond lengths (Å) and angles (°): O(1)–Pb(1) 2.416(12), O(1)–Pb(2) 2.244(13), O(2)–Pb(1) 2.485(13), O(2)–Pb(3) 2.442(12), O(3)–Si(1) 1.729(14), O(4)–Pb(2) 2.249(11), O(4)–Pb(3) 2.678(11), Pb(1)–O(6) 2.369(10), Pb(2)–O(6) 2.236(10), Pb(3)–O(6) 2.227(10), Pb(2)–O(1)–Pb(1) 104.9(4), Pb(3)–O(2)–Pb(1) 100.9(4), Pb(2)–O(4)–Pb(3) 95.8(4), O(1)–Pb(1)–O(2) 76.4(4), O(6)–Pb(1)–O(1) 120.3(4), O(6)–Pb(1)–O(2) 72.2(4), O(2)–Pb(3)–O(4) 131.7(4), O(6)–Pb(3)–O(2) 75.4(4), Pb(2)–O(6)–Pb(1) 114.8(5), Pb(3)–O(6)–Pb(1) 111.5(4), Pb(3)–O(6)–Pb(2) 110.5(4). | |
Furthermore, the precursor {Pb[N(TMS)2]}, generated in situ from (Me3Si)2NH, nBuLi and PbCl2, was treated with L6H6. Following work-up (MeCN), the complex [Pb10Li2(L6)2(OH)Cl(O)4]·9.5MeCN (6·9.5MeCN) was isolated. The molecular structure of 6·9.5MeCN is shown in Fig. 6, with selected bond lengths and angles given in the caption. The compound features a centrosymmetric cluster comprised of two symmetry-equivalent parts. Each calixarene binds one lithium ion and five lead ions, but between these ions there are oxide anions surrounded by four metal ions. In addition, there are hydroxide and chloride bridging between two lead ions (each 50% occupied and sharing a site). The two calixarenes do not lie face-on in the solid state but one is translated related to the other so that the bonding holing the Pb10Li2 cluster together is two pairs of Pb–O(calix) bonds; hydroxide and oxide are not involved in bridging.
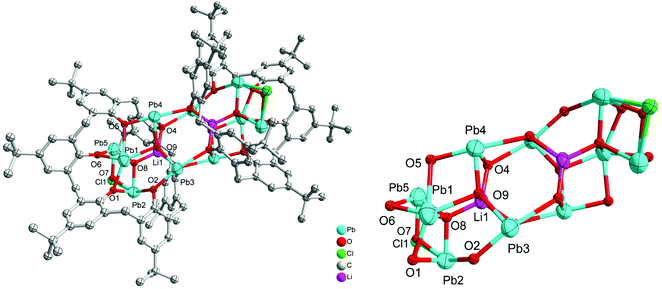 |
| Fig. 6 Molecular structure and core of [Pb10Li2(L6)2(OH)Cl(O)4]·9.5MeCN (6·9.5MeCN). Selected bond lengths (Å) and angles (°): Pb(3)–O(9) 2.158(3), Pb(3)–O(4) 2.728(3), Pb(3)–O(3) 2.392(3), Pb(3)–O(2) 2.306(3), Pb(5)–Cl(1) 2.669(4), Pb(4)–O(9) 2.159(3), Pb(4)–O(4) 2.428(3), Pb(4)–O(3) 2.719(3), Pb(4)–O(5) 2.313(3), O(9)–Pb(4)–O(4) 79.83(11), O(9)–Pb(4)–O(3) 99.61(10), O(9)–Pb(4)–O(5) 84.26(10), O(9)–Pb(4)–Li(1) 40.34(19), O(4)–Pb(4)–O(3) 70.32(9), O(8)–Pb(1)–O(1) 73.17(11), O(8)–Pb(1)–O(6) 74.46(10), O(6)–Pb(1)–O(1) 72.49(10), O(6)–Pb(1)–O(1) 72.49(10), O(8)–Pb(2)–O(1) 77.32(11), O(8)–Pb(2)–Li(1) 29.17(15), O(8)–Pb(2)–O(7) 72.2(3). | |
Finally, to avoid any possible chloride or lithium contamination, freshly distilled {Pb[N(TMS)2]} was reacted with L8H8. Work-up led to the isolation of the colourless complex [Pb12(L8)2O4]·12MeCN (7·12MeCN) in 31% isolated yield. A view of the molecular structure is shown in Fig. 7, with selected bond lengths and angles given in the caption. The asymmetric unit of 7·12MeCN contains two independent molecules each comprising one L8H8-derived ligand and five Pb ions (Pb1 to Pb5 in first molecule and the rest five Pb ion in second molecule. This is a centrosymmetric dimer bridged by the Pb2O2 square.
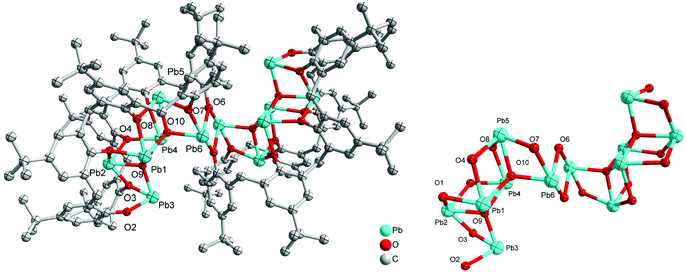 |
| Fig. 7 Molecular structure and core of [Pb12(L8)2O4]·12MeCN (7·12MeCN). For clarity, hydrogen atoms and solvent molecules are not shown. Selected bond lengths (Å) and angles (°): Pb(4)–O(10) 2.356(6), Pb(4)–O(9) 2.335(6), Pb(4)–O(5) 2.278(6), Pb(4)–O(4) 2.370(6), Pb(2)–O(9) 2.247(6), Pb(2)–O(3)––2.454(7), Pb(2)–O(4) 2.287(6), Pb(2)–O(1) 2.388(7), Pb(6)–O(6) 2.664(7), Pb(1)–O(10) 2.281(6), Pb(1)–O(9) 2.374(7), Pb(1)–O(8) 2.419(7), Pb(5)–O(10) 2.275(6), Pb(5)–O(5) 2.421(6), Pb(3)–O(9) 2.269(7), Pb(3)–O(2) 2.192(7), O(10)–Pb(4)–O(4) 119.7(2), O(9)–Pb(4)–O(10) 74.4(2), O(9)–Pb(4)–O(4) 72.3(2), O(5)–Pb(4)–O(10) 70.0(2), O(5)–Pb(4)–O(9) 111.7(2), O(9)–Pb(2)–O(3) 71.1(2), O(9)–Pb(2)–O(4) 75.5(2), O(4)–Pb(2)–O(3) 80.8(2), O(10)–Pb(1)–O(9) 75.1(2). | |
Ring opening polymerization studies
General.
The performance of these complexes to act as catalysts for the ring opening polymerization (ROP) of ε-caprolactone (ε-CL, Table 1), δ-valerolactone (δ-VL, Table 2), the co-polymerization of ε-caprolactone and δ-valerolactone (Table 3) and rac-lactide (r-LA, Table 4) has been investigated. Results are compared versus the precursors Pb(OiPr)2, and [Pb(N(TMS)2)2].
Table 1 ROP of ε-CL using 1–7, Pb(OiPr)2, and [Pb(N(TMS)2)2]
Run |
Cat. |
CL : Pb : BnOH |
T/°C |
t (h) |
Conva (%) |
M
n,GPC × 10−3 b |
M
w × 10−3 b |
M
n,cal × 10−3 c |
PDId |
TONf |
Determined by 1H NMR spectroscopy.
M
n/w, GPC values corrected considering Mark–Houwink factor (0.56) from polystyrene standards in THF.
Calculated from ([monomer]0/Pb) × conv (%) × monomer molecular weight (MCL = 114.14) + molecular weight of BnOH.
From GPC.
Reaction performed in air.
Turnover number (TON) = number of moles of ε-CL consumed/number of moles Pb.
|
1 |
1
|
1000 : 1 : 1 |
130 |
8 |
54.2 |
8.05 |
15.34 |
61.86 |
1.91 |
542 |
2 |
1
|
500 : 1 : 1 |
130 |
8 |
60.4 |
8.49 |
14.55 |
34.47 |
1.71 |
302 |
3 |
1
|
250 : 1 : 1 |
130 |
8 |
57.3 |
6.52 |
9.45 |
16.35 |
1.45 |
143 |
4 |
1
|
100 : 1 : 1 |
130 |
8 |
52.4 |
3.56 |
5.14 |
5.98 |
1.44 |
52 |
5 |
1
|
500 : 1 : 1 |
100 |
8 |
35.4 |
4.38 |
5.79 |
20.20 |
1.32 |
177 |
6 |
1
|
500 : 1 : 1 |
80 |
8 |
24.8 |
1.23 |
1.43 |
14.15 |
1.16 |
124 |
7 |
1
|
500 : 1 : 1 |
130 |
8 |
49.1 |
3.22 |
5.42 |
28.02 |
1.69 |
246 |
8 |
2
|
500 : 1 : 1 |
130 |
8 |
72.6 |
8.94 |
11.57 |
41.43 |
1.29 |
363 |
9 |
3
|
500 : 1 : 1 |
130 |
8 |
55.2 |
6.72 |
8.09 |
31.50 |
1.20 |
276 |
10 |
4
|
500 : 1 : 1 |
130 |
8 |
40.7 |
2.95 |
3.57 |
23.23 |
1.21 |
204 |
11 |
5
|
500 : 1 : 1 |
130 |
8 |
49.1 |
3.75 |
4.50 |
28.02 |
1.20 |
246 |
12 |
6
|
500 : 1 : 1 |
130 |
8 |
42.0 |
3.89 |
5.12 |
23.97 |
1.32 |
210 |
13 |
7
|
500 : 1 : 1 |
130 |
8 |
35.8 |
3.12 |
4.37 |
20.43 |
1.40 |
179 |
14 |
Pb(OiPr)2 |
500 : 1 : 1 |
130 |
8 |
24.9 |
— |
— |
— |
— |
124 |
15 |
[Pb(N(TMS)2)2] |
500 : 1 : 1 |
130 |
8 |
15.8 |
— |
— |
— |
— |
79 |
16 |
1
|
500 : 1 : 1 |
130 |
24 |
70.5 |
10.59 |
14.24 |
40.24 |
1.34 |
353 |
17 |
2
|
500 : 1 : 1 |
130 |
24 |
92.1 |
11.25 |
12.45 |
52.56 |
1.11 |
461 |
18 |
3
|
500 : 1 : 1 |
130 |
24 |
66.9 |
6.81 |
8.06 |
38.18 |
1.18 |
335 |
19 |
4
|
500 : 1 : 1 |
130 |
24 |
59.4 |
4.20 |
4.81 |
33.90 |
1.15 |
297 |
20 |
5
|
500 : 1 : 1 |
130 |
24 |
62.6 |
5.11 |
6.09 |
35.73 |
1.19 |
313 |
21 |
6
|
500 : 1 : 1 |
130 |
24 |
65.8 |
4.89 |
5.25 |
37.55 |
1.07 |
329 |
22 |
7
|
500 : 1 : 1 |
130 |
24 |
49.8 |
4.01 |
5.32 |
28.42 |
1.33 |
249 |
23 |
Pb(OiPr)2 |
500 : 1 : 1 |
130 |
24 |
33.8 |
1.35 |
1.56 |
19.29 |
1.16 |
169 |
24 |
[Pb(N(TMS)2)2] |
500 : 1 : 1 |
130 |
24 |
27.1 |
1.24 |
1.74 |
15.47 |
1.40 |
135 |
25 |
1
|
500 : 1 : 0 |
130 |
24 |
44.7 |
8.33 |
8.73 |
25.51 |
1.05 |
224 |
26 |
2
|
500 : 1 : 0 |
130 |
24 |
72.4 |
5.23 |
6.98 |
41.32 |
1.33 |
362 |
27 |
3
|
500 : 1 : 0 |
130 |
24 |
58.1 |
3.21 |
4.53 |
33.16 |
1.41 |
291 |
28 |
4
|
500 : 1 : 0 |
130 |
24 |
35.2 |
2.51 |
3.70 |
20.09 |
1.47 |
176 |
29 |
5
|
500 : 1 : 0 |
130 |
24 |
52.8 |
2.86 |
4.21 |
30.13 |
1.47 |
264 |
30 |
6
|
500 : 1 : 0 |
130 |
24 |
36.0 |
2.54 |
4.65 |
20.55 |
1.83 |
180 |
31 |
7
|
500 : 1 : 0 |
130 |
24 |
36.4 |
2.86 |
3.27 |
20.77 |
1.14 |
182 |
32 |
Pb(OiPr)2 |
500 : 1 : 0 |
130 |
24 |
28.4 |
0.56 |
0.82 |
16.21 |
1.46 |
142 |
33 |
[Pb(N(TMS)2)2] |
500 : 1 : 0 |
130 |
24 |
19.6 |
0.72 |
1.25 |
11.19 |
1.74 |
98 |
Table 2 ROP of δ-VL using 1–7, Pb(OiPr)2, and [Pb(N(TMS)2)2]
Run |
Cat. |
VL : Pb : BnOH |
T/°C |
t (h) |
Conva (%) |
M
n,GPC × 10−3 b |
M
w × 10−3 b |
M
n,cal × 10−3 c |
PDId |
TONf |
Determined by 1H NMR spectroscopy.
M
n/w, GPC values corrected considering Mark–Houwink factor (0.57) from polystyrene standards in THF.
Calculated from ([monomer]0/Pb) × conv (%) × monomer molecular weight (MVL = 100.16) + molecular weight of BnOH.
From GPC.
Reaction performed in air.
Turnover number (TON) = number of moles of δ-VL consumed/number of moles Pb.
|
1 |
1
|
1000 : 1 : 1 |
130 |
8 |
61.2 |
7.53 |
12.57 |
61.29 |
1.67 |
612 |
2 |
1
|
500 : 1 : 1 |
130 |
8 |
68.9 |
7.90 |
12.87 |
34.50 |
1.63 |
345 |
3 |
1
|
250 : 1 : 1 |
130 |
8 |
61.0 |
5.12 |
8.56 |
15.27 |
1.67 |
153 |
4 |
1
|
100 : 1 : 1 |
130 |
8 |
55.5 |
2.52 |
3.96 |
5.56 |
1.57 |
56 |
5 |
1
|
500 : 1 : 1 |
100 |
8 |
41.2 |
3.49 |
7.12 |
20.63 |
2.04 |
206 |
6 |
1
|
500 : 1 : 1 |
80 |
8 |
31.5 |
— |
— |
— |
— |
158 |
7 |
1
|
500 : 1 : 1 |
130 |
8 |
43.1 |
4.01 |
6.54 |
21.58 |
1.63 |
216 |
8 |
2
|
500 : 1 : 1 |
130 |
8 |
75.9 |
10.98 |
13.83 |
38.00 |
1.26 |
380 |
9 |
3
|
500 : 1 : 1 |
130 |
8 |
52.4 |
5.32 |
6.00 |
26.24 |
1.13 |
262 |
10 |
4
|
500 : 1 : 1 |
130 |
8 |
36.2 |
2.15 |
2.65 |
18.13 |
1.23 |
181 |
11 |
5
|
500 : 1 : 1 |
130 |
8 |
34.3 |
1.97 |
2.38 |
17.18 |
1.20 |
172 |
12 |
6
|
500 : 1 : 1 |
130 |
8 |
37.8 |
2.23 |
4.02 |
18.92 |
1.80 |
189 |
13 |
7
|
500 : 1 : 1 |
130 |
8 |
30.1 |
1.54 |
2.86 |
15.07 |
1.86 |
176 |
14 |
Pb(OiPr)2 |
500 : 1 : 1 |
130 |
8 |
26.2 |
— |
— |
— |
— |
131 |
15 |
[Pb(N(TMS)2)2] |
500 : 1 : 1 |
130 |
8 |
14.2 |
— |
— |
— |
— |
71 |
Table 3 ROP of co-polymer (ε-CL + δ-VL) using 1–7
Run |
Cat |
CL : VL : Pb : BnOH |
T/°C |
CL : VLa |
Convb (%) |
M
n,GPC × 10−3 c |
M
w × 10−3 c |
PDId |
Ratio of ε-CL to δ-VL observed in the co-polymer by 1H NMR spectroscopy.
Determined by 1H NMR spectroscopy.
M
n/w, GPC values corrected considering Mark–Houwink method from polystyrene standards in THF, Mn/w GPC = [0.56 × Mn/w measured × (1 − %CL) + 0.57 × Mn/w measured × (1 − %VL)] × 103.
From GPC.
ε-Caprolactone was firstly added for 24 h, then δ-valerolactone was added and heating for 24 h.
δ-Valerolactone was firstly added for 24 h, then ε-caprolactone was added and heating for 24 h.
ε-Caprolactone and δ-valerolactone were added at the same time and heating for 24 h.
|
1 |
1
|
250 : 250 : 1 : 1 |
130 |
42 : 58 |
61.0 |
10.98 |
13.83 |
1.26 |
2 |
2
|
250 : 250 : 1 : 1 |
130 |
50 : 50 |
68.4 |
11.10 |
15.97 |
1.44 |
3 |
3
|
250 : 250 : 1 : 1 |
130 |
52 : 48 |
52.6 |
6.97 |
8.69 |
1.25 |
4 |
4
|
250 : 250 : 1 : 1 |
130 |
45 : 55 |
53.1 |
5.60 |
8.37 |
1.50 |
5 |
5
|
250 : 250 : 1 : 1 |
130 |
43 : 57 |
47.2 |
4.56 |
6.89 |
1.51 |
6 |
5
|
250 : 250 : 1 : 1 |
130 |
64 : 36 |
53.9 |
6.16 |
7.56 |
1.23 |
7 |
5
|
250 : 250 : 1 : 1 |
130 |
49 : 51 |
46.2 |
3.96 |
5.89 |
1.49 |
8 |
6
|
250 : 250 : 1 : 1 |
130 |
45 : 55 |
39.2 |
4.55 |
6.24 |
1.37 |
9 |
7
|
250 : 250 : 1 : 1 |
130 |
46 : 54 |
28.4 |
2.95 |
4.78 |
1.62 |
Table 4 ROP of rac-lactide using complexes 1–7, Pb(OiPr)2, and [Pb(N(TMS)2)2]
Run |
Cat. |
LA : Pb : BnOH |
T/°C |
t (h) |
Conva (%) |
M
n,GPC × 10−3 b |
M
w × 10−3 b |
P
r c |
M
n,cal × 10−3 d |
PDIe |
TONf |
Determined by 1H NMR spectroscopy on crude reaction mixture.
M
n/w, GPC values corrected considering Mark–Houwink factor (0.58) from polystyrene standards in THF.
From 2D J-resolved 1H NMR spectroscopy.
Calculated from ([monomer]0/Pb) × conv. (%) × monomer molecular weight (MLA = 144.13) + molecular weight of BnOH.
From GPC.
Turnover number (TON) = number of moles of rac-lactide consumed/number of moles Pb.
|
1 |
1
|
500 : 1 : 1 |
130 |
24 |
64.3 |
5.33 |
9.01 |
0.45 |
46.40 |
1.69 |
322 |
2 |
2
|
500 : 1 : 1 |
130 |
24 |
56.2 |
6.82 |
14.51 |
0.61 |
40.57 |
2.13 |
281 |
3 |
3
|
500 : 1 : 1 |
130 |
24 |
24.9 |
1.45 |
2.46 |
0.52 |
18.04 |
1.70 |
125 |
4 |
4
|
500 : 1 : 1 |
130 |
24 |
34.9 |
1.64 |
3.05 |
0.39 |
25.24 |
1.86 |
175 |
5 |
5
|
500 : 1 : 1 |
130 |
24 |
30.5 |
1.63 |
2.76 |
0.55 |
22.07 |
1.69 |
153 |
6 |
6
|
500 : 1 : 1 |
130 |
24 |
35.8 |
2.14 |
3.89 |
0.51 |
25.80 |
1.82 |
179 |
7 |
7
|
500 : 1 : 1 |
130 |
24 |
31.6 |
1.83 |
3.44 |
0.49 |
22.77 |
1.88 |
158 |
8 |
Pb(OiPr)2 |
500 : 1 : 1 |
130 |
24 |
54.7 |
5.45 |
8.45 |
0.52 |
31.22 |
1.55 |
273 |
9 |
[Pb(N(TMS)2)2] |
500 : 1 : 1 |
130 |
24 |
52.4 |
3.92 |
4.60 |
0.55 |
29.90 |
1.17 |
262 |
ε-Caprolactone (ε-CL)
Complexes 1–7, Pb(OiPr)2, and [Pb(N(TMS)2)2] were screened for their ability to polymerise ε-caprolactone and the results are collated in Table 1. The polymerization screening indicated that the best conditions were 500 equivalents of ε-caprolactone to metal at a temperature of 130 °C. Complex 1 was also active at low catalyst loading leading to ca. 54% conversion after 8 h for 1000 equivalents of monomer. All polymers obtained were of low polydispersity (PDI < 2), which suggested that these polymerizations occurred without significant side reactions. Interestingly, complex 1 proved to be active also under aerobic conditions achieving ca. 49% conversion over 8 h (Table 1, run 7).
The screening of complexes 1–7, Pb(OiPr)2, and [Pb(N(TMS)2)2] (Table 1) revealed that the lead/lithium-based complexes 1 and 2 exhibited higher activities than other complexes under the conditions employed herein. After 24 h (Table 1), complexes 3–7 afforded relatively lower conversions (<70%), whereas higher conversions (>70%) were reached using complexes 1 and 2, under similar conditions. The higher conversions achieved using 1 and 2 may be attributed to the presence of the lithium centers, although it should be noted that the lability of the MeCN ligands present in these species may also prove beneficial. This is in line with our recent study on titanocalix[4]arenes, in which the presence of a labile ligand (i.e. MeCN and H2O) proved beneficial for the catalyst activity.12 From a kinetic study (Fig. 8), it was observed that the PCL polymerization rate followed the order: 2 > 1 > 3 > 4 ≈ 5 ≈ 6 > 7 (with first order dependence, see Kinetics section). In general, compared with the larger lead-calix[8]arene complexes 4, 5 and 7, those derived from calix[4 or 6]arenes, i.e.1–3, were found to be relatively more active (Table 1, runs 16–18). This is similar to the situation observed for a series of tungstocalix[6 and 8]arenes and lithium-containing calix[6 and 8]arenes, where use of L8-derived complexes was detrimental for the ROP of cyclic esters.13,14 Indeed, results for the lead calixarene systems herein are comparable with those of the lithium calix[6 and 8]arenes under similar conditions (130 °C, 24 h, 500
:
1
:
1); conversions for the latter are in the range 35–81%. An aluminium/lithium system was found to be more active (98%).14 Furthermore, compared with 4 and 5, the higher activity of 3 was thought to be due to the higher flexibility of the –CH2OCH2– bridge allowing better access to the active centre(s) and/or to the stabilization of the active species by the oxygen atoms of said bridge.11 The precursors Pb(OiPr)2, and [Pb(N(TMS)2)2] were far less effective for the ring polymerization of ε-CL (runs 14/15, 23/24, 32/33). Based on the results in Table 1 (runs 16–24), the activity trend was found to be 2 > 1 > 3 > 4 ≈ 5 ≈ 6 > 7 > Pb(OiPr)2 > [Pb(N(TMS)2)2]. The MALDI-ToF mass spectra (e.g. Fig. S2, ESI†) indicated the presence of a BnO end group, which agrees with the 1H NMR spectra of the PCL (e.g. Fig. S3, ESI†) and indicates that the polymerization proceeded via a coordination insertion mechanism. Indeed, the MALDI-ToF spectrum of the sample displayed a major series of peaks separated by 114 m/z units accountable to two OH terminated PCL n-mers [M = 17 (OH) + 1(H) + n × 114.14 (CL) + 22.99 (Na+)]. Additionally, there is a family of peaks consistent with the polymer terminated by OH and BnO end groups [M = n × 114.12 (CL) + 108.05 (BnOH) + 22.99 (Na+)].
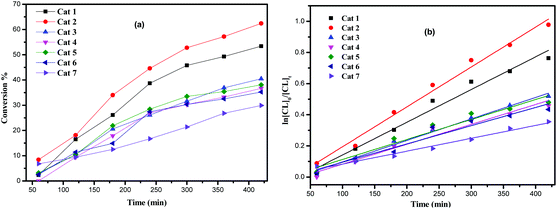 |
| Fig. 8 (a) Relationship between conversion and time for the polymerization of ε-CL by using complex 1–7; (b) plot of ln[CL]0/[CL]tvs. time for the polymerization of ε-CL by using complexes 1–7; conditions: T = 130 °C, nmonomer : nPb : BnOH = 500 : 1 : 1. | |
δ-Valerolactone (δ-VL)
Complexes 1–7, Pb(OiPr)2, and [Pb(N(TMS)2)2] were also evaluated as catalysts, in the presence of one equivalent of BnOH, for the ROP of δ-VL (Table 2). Using 1, the conditions of temperature and [Pb]
:
[δ-VL] ratio were varied. On increasing the temperature to 130 °C and lowering the monomer to catalyst ratio, best observed results were achieved at 130 °C using [V]
:
[δ-VL] at 1
:
500 over 8 h. As in the case of the ROP of ε-CL, kinetic studies (Fig. 9) revealed that the catalytic activities followed the order: 2 > 1 > 3 > 4 ≈ 5 ≈ 6 ≈ 7 > Pb(OiPr)2 > [Pb(N(TMS)2)2]. The systems 1 and 2 (runs 2 and 8, Table 2), which contain the smaller calix[4 or 6]arenes, outperform lithiated calix[6 and 8]arenes under similar conditions (500
:
1
:
1, 130 °C, 8 h); the conversions for the latter are 33–55%. An aluminium/lithium system was found to be more active (89%).14
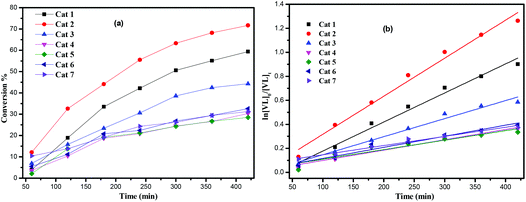 |
| Fig. 9 (a) Relationship between conversion and time for the polymerization of δ-VL by using complex 1–7; (b) plot of ln[VL]0/[VL]tvs. time for the polymerization of δ-VL by using complexes 1–7; conditions: T = 130 °C, nmonomer : nPb : BnOH = 500 : 1 : 1. | |
As for the ROP of ε-Cl, there was evidence of significant transesterification and nearly all observed Mn values were significantly lower than the calculated values. The MALDI-ToF mass spectra (Fig. S4, ESI†) exhibited a major family of peaks consistent with BnO end groups [M = 108.05 (BnOH) + n × 100.12 (VL) + 22.99 (Na+)], and a family assigned to the PVL with two OH end groups [M = 17 (OH) + 1(H) + n × 114.14 (CL) + 22.99 (Na+)]. 1H NMR spectra of the PVL also indicated the presence of an BnO end group (e.g. Fig. S5, ESI†).
Co-polymerization of ε-CL and δ-VL
The complexes exhibited moderate conversions, with the mixed-metal complex 2 performing best (68.4%). Under the conditions employed, the systems 2, 3 and 5 showed a preference for CL incorporation (50–64%), and in the case of 5, this was despite the initial addition of δ-VL. Complex 1 exhibited the highest preference (58%) for VL incorporation. In general, the systems appeared to be relatively well behaved with PDIs in the range 1.23–1.62; 1H NMR spectra were consistent with the presence of BnO and OH end groups (Fig. S6, ESI†). The composition of the copolymer was further investigated by 13C NMR spectroscopy. In fact, diagnostic resonances belonging to CL–VL, CL–CL, VL–VL and VL–CL dyads can be observed in the region between δ 63.73 and 64.35 ppm (Fig. S7, ESI†). Based on the current results, the number-average sequence length was found to be 1.35 and 3.09 for CL and VL, respectively, consistent with a randomness degree R of 0.98, which suggests the copolymers possess a “blocking” tendency (Fig. S7, eqn (S1)–(S3), ESI†).15
ROP of rac-lactide
Selected complexes were also employed, in combination with BnOH, as catalysts in the ROP of r-LA (Table 4). Best conversion was achieved in the presence of 1 (64.3%, run 1). The Mn of the polymer was lower than the calculated value albeit with narrow molecular weight distribution (5330 and 1.69, respectively). In the case of systems 1–7, all polymers obtained were of relatively low polydispersity (PDI < 2.13), which suggested that there was reasonable control for polymerization. However, 3 only allowed for ca. 25% monomer conversion affording low molecular weight species. Interestingly, compared with ε-CL and δ-VL, both Pb(OiPr)2, and [Pb(N(TMS)2)2] exhibited better performances for the ROP of r-LA (runs 8 and 9), whereas surprisingly the dioxacalix[6]arene-derived system performed the worst in terms of conversion. Conversions, PDIs and TONs were comparable with the best performing lead calixarenes. Moreover, the lead calixarenes were found to be comparable with lithium-based calix[6 and 8]arenes (23–55% conversion) under the same conditions (500
:
1
:
1, 130 °C, 24 h), with 1 exhibiting a slightly higher conversion than an aluminium/lithium calix[8]arene (62%).14 Based on the results in Table 4 (runs 1–9), it was observed that the PLA polymerization conversion rate followed the order: 1 > 2 ≈ Pb(OiPr)2 ≈ [Pb(N(TMS)2)2] > 4 > 5 ≈ 6 ≈ 7 > 3. 1H NMR spectra of the PLA indicated the presence of a BnO end group (e.g. Fig. S8, ESI†), which agrees with the MALDI-ToF mass spectra (e.g. Fig. S9, ESI†). The sample was analysed by MALDI-ToF mass spectra in positive-linear mode, the expected series corresponding to repeating unit mass of 72/144 for half/full LA was observed and the polymer chain was terminated by OH and BnO end group [M = 108.05 (BnOH) + n × 72.06 (C3H4O2) + 22.99 (Na+)]. The syndiotactic bias was determined by 2D J-resolved 1H NMR spectroscopy, investigating the methine area (5.13–5.20 ppm) of the spectra (e.g. figure. S10, ESI†).16 The peaks were assigned to the corresponding tetrads according to the literature.17 For rac-lactide, when Pr = 0.5, the afforded PLA is an atactic polymer, and when Pr = 0, an isotactic polymer. The observed values herein (Pr = 0.39–0.61) suggested the catalysts afforded almost heterotactic polymers.
Kinetics
From a kinetic study of the ROP of ε-CL using 1–7, it was observed that the polymerization rate exhibited first-order dependence on the ε-CL concentration (Fig. 8), and the conversion of monomer achieved over 420 min was >20%. The activity trend in this case revealed that 2 was the most active followed by 1 > 3 > 4 ≈ 5 ≈ 6 > 7. An induction period of the first 2 h was observed for complexes 1–7, which could be ascribed to the longer time required for the formation of the catalytically active species. A similar result was also observed for the polymerization of δ-VL (Fig. 9).
The dependence of the Mn and molecular weight distribution on the monomer conversion in the reactions catalyzed by 1, 3, 5 and 7 with BnOH was also investigated (Fig. 10). For the ROP of ε-CL, the polymer Mn was shown to increase linearly with the conversion, which suggested that the polymerization was well controlled (Fig. 10, left). A similar outcome was also observed in the reaction involving δ-VL (Fig. 10, right).
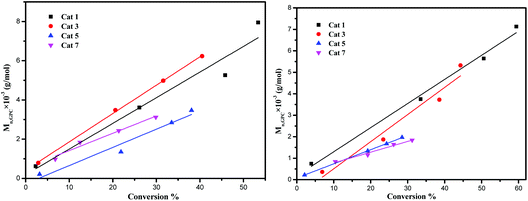 |
| Fig. 10 Left: Mnvs. monomer conversion in the ROP of ε-CL by using 1, 3, 5 and 7; right: Mnvs. monomer conversion in the ROP of δ-VL by using 1, 3, 5 and 7; conditions: T = 130 °C, nmonomer : nPb : BnOH = 500 : 1 : 1. | |
Conclusions
In conclusion, the use of the precursors [LiPb(OiPr)3], Pb(OiPr)2, Pb(OtBu)2 or Pb(N(TMS)2)2, on interaction with a series of calix[n]arenes (n = 4, 6, 8) allowed access to a number of rather complicated lead compounds (see Chart 2). The molecular structures reveal how these macrocycles can support multiple metal centres, which adopt some interesting structural motifs. Complex 1–7 proved active in the ring opening homo-/co-polymerization of ε-caprolactone (ε-CL) and δ-valerolactone (δ-VL) under the conditions employed, and the activity trend was found to be 2 > 1 > 3 > 4 ≈ 5 ≈ 6 > 7 > Pb(OiPr)2 > [Pb(N(TMS)2)2]; first order kinetics were observed for the ROP of ε-CL and δ-VL. The NMR spectroscopy and mass spectrometry (MALDI-ToF) characterization of selected polymer samples suggested the formation of linear PCLs and PVLs ended by –BnO and –OH groups. The catalysts 1–7 also be proved to be active in the ROP of the rac-lactide (r-LA), the activity trend was found to be 1 > 2 ≈ Pb(OiPr)2 ≈ [Pb(N(TMS)2)2] > 4 > 5 ≈ 6 ≈ 7 > 3, affording near heterotactic polymers.
Experimental
General
All reactions were conducted under an inert atmosphere using standard Schlenk techniques. Toluene and hexane was dried from sodium, acetonitrile was distilled from calcium hydride, diethylether was distilled from sodium benzophenone, and all solvents were degassed prior to use. The dioxacalix[6]arene,18 [LiPb(OiPr)3],4 [Pb(N(TMS)2)2],19 Pb(OiPr)2 and Pb(OtBu)2
20 were prepared according to the literature methods. All other chemicals were purchased from commercial sources. IR spectra (nujol mulls, KBr or NaCl windows) were recorded on a Nicolet Avatar 360 FT IR spectrometer; 1H NMR spectra were recorded at room temperature on a Varian VXR 400 S spectrometer at 400 MHz or a Gemini 300 NMR spectrometer or a Bruker Advance DPX-300 spectrometer at 300 MHz. The 1H NMR spectra were calibrated against the residual protio impurity of the deuterated solvent. Elemental analyses were performed by the elemental analysis service at the University of Hull. Matrix Assisted Laser Desorption/Ionization Time of Flight (MALDI-TOF) mass spectrometry was performed in a Bruker autoflex III smart beam in linear mode, and the spectra were acquired by averaging at least 100 laser shots. 2,5-Dihydroxybenzoic acid was used as the matrix and THF as solvent. Sodium chloride was dissolved in methanol and used as the ionizing agent. Samples were prepared by mixing 20 μl of matrix solution in THF (2 mg mL−1) with 20 μL of matrix solution (10 mg mL−1) and 1 μL of a solution of ionizing agent (1 mg mL−1). Then 1 mL of these mixtures was deposited on a target plate and allowed to dry in air at ambient temperature.
Preparation of [Pb4Li2(L4)4H6(MeCN)3]·4.5MeCN (1·4.5MeCN)
L4H4 (1.00 g, 1.54 mmol) and [LiPb(OiPr)3] (0.63 g, 1.57 mmol), generated in situ from PbCl2 and LiOiPr, were combined in toluene (20 mL) and the system was refluxed for 12 h. On cooling, the volatiles were removed in vacuo, and the residue was extracted into MeCN (20 mL). On standing at 0 °C for 2 days, colourless prisms formed. Yield, 0.53 g, 37%. Anal. calcd for C182H223Li2N3O17Pb4 (sample dried in vacuo for 12 h, −4.5MeCN): C, 61.28; H, 6.30; N, 1.18%; found C, 61.40; H, 6.25%; N, 1.51%; IR (nujol mull, KBr): 2726w, 2263w, 1607w, 1460s, 1377s, 1304m, 1280m, 1260s, 1204m, 1125m, 1093m, 1018m, 914w, 872m, 818s, 795s. 1H NMR (CDCl3) δ: 10.33 (s, 6H, –OH), 6.98–7.48 (m, 32H, arylH), 4.42–4.38 (m, 4H, –CH2), 4.24 (d, J = 14 Hz, 12H, –CH2–), 3.83–3.89 (m, 4H, –CH2–), 3.48 (d, J = 14 Hz, 12H, –CH2–), 1.96 (s, 12H, MeCN), 1.13–1.36 (m, 144H, –C(CH3)3). 7Li (CDCl3) δ: 3.61(bs). 207Pb NMR (C6D6) δ: −2392 (s), −3401 (s). Mass Spec (EI): 1761 ([M − 7.5MeCN]/2 + 2Na+).
Preparation of [Pb8Li10Cl2(L6H2)3(L6)(OH)2(O)2(H2O)2(MeCN)4]·14MeCN (2·14MeCN)
L6H6 (1.00 g, 1.03 mmol) and [LiPb(OiPr)3] (0.82 g, 2.04 mmol), generated in situ from PbCl2 and LiOiPr, were combined in toluene (20 mL) and the system was refluxed for 12 h. On cooling, the volatiles were removed in vacuo, and the residue was extracted into MeCN (20 mL). On standing at 0 °C for 2 days, colourless prisms formed. Yield, 0.79 g, 47%. Anal. calcd for C268H323Cl2Li10N2O30Pb8 (sample dried in vacuo for 12 h, −16MeCN): C, 55.02; H, 5.57; N, 0.48%; found C, 54.75; H, 5.24; N, 0.79%; IR: 2726w, 2359w, 1645w, 1462s, 1377m, 1364m, 1297w, 1260m, 1203m, 1093m, 1021m, 909w, 872w, 801m, 735m, 527w. 1H NMR (CDCl3) δ: 6.95–7.29 (m, 48H, arylH), 4.56 (d, J = 12 Hz, 12H, –CH2–), 3.98 (d, J = 12 Hz, 12H, –CH2–), 3.46 (d, J = 4.8 Hz, 6H, –CH2–), 3.08–3.18 (m, 18H, –CH2–), 1.97 (s, 18H, MeCN), 1.50 (s, 4H, H2O), 1.13–1.30 (m, 216H, –C(CH3)3). 7Li (CDCl3) δ: 3.82 (bs), 3.04 (s), 2.83 (bs), 2.61 (bs), 2.41 (bs). 207Pb NMR (C6D6) δ: −2911 (s), −2932 (s), −2957 (s).
Preparation of [Pb13(L6′)3O4(iPrOH)]·11MeCN (3·11MeCN)
To Pb(OiPr)2 (0.96 g, 2.94 mmol) and L6′H6 (0.5 g, 0.49 mmol) was added toluene (30 mL) and the system was refluxed for 12 h. On cooling, the volatiles were removed in vacuo, and the residue was extracted into MeCN (30 mL). On standing at room temperature for 3 days, colourless prisms formed. Yield, 0.35 g, 35%. Anal. calcd for C204H245O28Pb13 (sample dried in vacuo for 12 h, −11MeCN and iPrOH): C, 41.97; H, 4.23%; found C, 42.23; H, 4.54%; IR (nujol mull, KBr): 2726w, 2359w, 1651w, 1539w, 1462s, 1377m, 1260s, 1092s, 1018s, 871s, 799s, 722w. 1H NMR (CDCl3) δ: 6.91–7.62 (m, 36H, arylH), 4.85–5.20 (m, 12H, –OCH2–), 4.45–4.60 (m, 12H, –OCH2–), 4.02–4.22 (m, 12H, –CH2–), 3.39–3.47 (m, 6H, –CH2–), 3.22–3.30 (m, 6H, –CH2–), 1.10–1.32 (m, 162H, –C(CH3)3). 207Pb NMR (C6D6) δ: −2030 (s), −2069 (s), −3301 (bs), −3046 (bs), −3794(s), −3994 (s).
Preparation of [Pb12(L8)2O4]·8.7C7H8 (4·8.7C7H8)
To Pb(OtBu)2 (1.63 g, 4.62 mmol) and L8H8 (1.00 g, 0.77 mmol) was added toluene (30 mL) and the system was refluxed for 3 h. On cooling, the volatiles were removed in vacuo, and the residue was extracted into MeCN (30 mL). On standing at 0 °C for 2 days, colourless prisms formed. Yield, 0.68 g, 35%. Anal. calcd for C176H208O20Pb12 (sample dried in vacuo for 12 h, −8.7C7H8): C, 41.21; H, 4.09%; found C, 41.25; H, 4.50%. IR (nujol mull, KBr): 1685w, 1601w, 1493m, 1362s, 1296s, 1280s, 1260s, 1197s, 1163m, 1117s, 1094bs, 1020bs, 946w, 912w, 899w, 878m, 870m, 817s, 800s, 728s, 702w, 693m, 664w, 634w, 611w, 552w, 528m, 539m, 488m, 475m, 463s. 1H NMR (CDCl3): δ: 6.98–7.34 (m, 16H, arylH), 4.94–5.12 (m, 2H, –CH2–), 4.30–4.40 (m, 4H, –CH2–) 4.13–4.15 (m, 4H, –CH2–), 3.34–3.45 (m, 6H, –CH2–), 1.19–1.27 (m, 72H, –C(CH3)3). 207Pb NMR (C6D6) δ: −2071 (s), −2621 (s). Mass Spec (EI): 2614.7 [M + 2Na+].
Preparation of [Pb6(SiMe3)2(L8)O2Cl2] (5)
To Pb(N(TMS)2)2 (2.44 g, 4.62 mmol) and L8H8 (1.0 g, 0.77 mmol) was added toluene (30 mL) and the system was refluxed for 12 h. On cooling, the volatiles were removed in vacuo, and the residue was extracted into MeCN (30 mL). On standing at 0 °C for 3 days, colourless prisms formed. Yield, 0.71 g, 33%. C94H122Cl2O10Pb6Si2 (sample dried in vacuo for 12 h) requires C, 40.58; H, 4.42%; found C, 40.23; H, 4.85%. IR (nujol mull, KBr): 2727w, 2359m, 2341s, 1657w, 1461m, 1414s, 1377m, 1260s, 1200w, 1091s, 1019s, 908w, 867w, 799s, 722w, 703w, 667w. 1H NMR (CDCl3) δ: 6.98–7.24 (m, 16H, arylH), 5.11 (d, J = 12 Hz, 2H, –CH2), 4.87 (m, 4H, –CH2–), 4.47 (m, 4H, –CH2–), 3.97 (d, J = 12 Hz, 2H, –CH2), 3.33 (m, 4H, –CH2–), 1.13–1.34 (m, 72H, –C(CH3)3), 0.07–0.12 (m, 18H, –SiMe3). 207Pb NMR (C6D6) δ: −2918 (s). Mass Spec (EI): 2568.1 [M − 2SiMe3 − 2Cl−].
Preparation of [Pb10Li2(L6)2(OH)Cl(O)4]·9.5MeCN (6·9.5MeCN)
To [Pb(N(TMS)2)2] (1.46 mL, 3.06 mmol), generated in situ from hexamethyldisilazane (15.23 mL, 73.58 mmol), nBuLi (1.6 M in heptane, 45.99 mL, 73.58 mmol) and PbCl2 (10.23 g, 36.79 mmol) in THF (10 mL), was added L6H6 (0.5 g, 0.51 mmol) in toluene (30 mL) and the system was refluxed for 3 h. On cooling, the volatiles were removed in vacuo, and the residue was extracted into MeCN (30 mL). On standing at room temperature for 3 days, colourless prisms formed. Yield, 0.49 g, 46%. C136H163N2ClLi2O17Pb10 (sample dried in-vacuo for 12 h, −7.5MeCN) requires C, 38.73; H, 3.87; N, 0.66%; found C, 39.11; H, 3.69; N, 0.74%, IR (nujol mull, KBr): 2727w, 2359w, 2340w, 1716w, 1652w, 1505w, 1457s, 1377m, 1260s, 1199w, 1092s, 1018s, 872w, 799s, 722m. 1H NMR (CDCl3) δ: 6.98–7.24 (m, 16H, arylH), 5.23–5.29 (m, 4H, –CH2–), 4.65 (d, J = 4.8 Hz, 8H, –CH2–) 3.91–4.00 (m, 4H, –CH2–), 3.47 (d, J = 4.8 Hz, 8H, –CH2–), 1.99 (s, 6H, MeCN), 1.20–1.35 (m, 108H, –C(CH3)3). 7Li (CDCl3): δ = 4.38 (s). 207Pb NMR (C6D6) δ: −2234 (bs), −2525 (bs), −2554 (s), −2874 (bs). Mass Spec (EI): 2056.2 [(M − 9.5MeCN)/2 − Cl− + Na+].
Preparation of [Pb12(L8)2O4]·12MeCN (7·12MeCN)
The crude (Pb(N(TMS)2)2) product was distilled at 80 °C in vacuo.16 To distilled [Pb(N(TMS)2)2] (2.44 g, 4.62 mmol) and L8H8 (1.0 g, 0.77 mmol) was added toluene (30 mL) and the system was refluxed for 12 h and affording 7 as colourless prisms. Single colourless prisms were grown from a saturated MeCN (30 mL) solution at 0 °C (yield 0.61 g, 31%). Anal. cald for C176H206O20Pb12 (sample dried in vacuo for 12 h, −12MeCN): anal. cald for C, 41.22 H, 4.05%; found C, 41.62; H, 4.33%. IR (nujol mull, KBr): 2955s, 2853s, 1617w, 1540w, 1462s, 1377m, 1293w, 1259s, 1203s. 1094m, 1019m, 908w, 874w, 817m, 800s, 722w. 1H NMR (C6D6) δ: 6.89–7.46 (m, 16H, arylH), 4.76–4.98 (m, 12H –CH2–), 4.22–4.36 (m, 6H, –CH2–), 3.27–3.57 (m, 12H, –CH2–), 2.95 (d, J = 4.8 Hz, 2H, –CH2–), 1.29–1.39 (m, 144H, –C(CH3)3), 207Pb NMR (C6D6) δ: −2461 (s), −2685 (s), −2861 (s). Mass Spec (EI): 2587.2 [(M − 12MeCN)/2 + Na+].
Procedure for ROP of ε-caprolactone, δ-valerolactone and rac-lactide
A toluene solution of pre-catalyst (0.010 mmol, 1.0 mL toluene) was added into a Schlenk tube in the glove-box at room temperature. The solution was stirred for 2 min, and then the appropriate equivalent of BnOH (from a pre-prepared stock solution of 1 mmol BnOH in 100 mL toluene) and the appropriate amount of ε-CL, δ-VL or r-LA along with 1.5 mL toluene was added to the solution. The reaction mixture was then placed into an oil/sand bath pre-heated at 130 °C, and the solution was stirred for the prescribed time (8 or 24 h). The polymerization mixture was quenched on addition of an excess of glacial acetic acid (0.2 mL) into the solution, and the resultant solution was then poured into methanol (200 mL). The resultant polymer was then collected on filter paper and was dried in vacuo.
Kinetic studies
The polymerizations were carried out at 130 °C in toluene (2 mL) using 0.010 mmol of complex. The molar ratio of monomer to initiator to co-catalyst was fixed at 500
:
1
:
1, and at appropriate time intervals, 0.5 μL aliquots were removed (under N2) and were quenched with wet CDCl3. The percent conversion of monomer to polymer was determined using 1H NMR spectroscopy.
X-ray crystallography
In all cases, crystals suitable for an X-ray diffraction study were grown from a saturated MeCN solution at either ambient temperature or 0 °C. Single crystal X-ray diffraction data were collected at the UK National Crystallography service using Rigaku Oxford Diffraction ultra-high intensity instruments employing modern areas detectors. In all cases standard procedures were employed for integration and processing of data.
Crystal structures were solved using dual space methods implemented within SHELXT.21 Completion of structures was achieved by performing least squares refinement against all unique F2 values using SHELXL-201822 sometimes implemented in Olex2. All non-H atoms were refined with anisotropic displacement parameters. Hydrogen atoms were placed using a riding model. Minor disorder was treated using standard methods. SQUEEZE23 was used to model the disordered solvent in all the structures, except for 4 in which the electron density not associated with the Pb cluster, in two small pockets, was very small and this was not modelled. These are large and complicated structures, but we have been able to identify the atomic arrangements unequivocally. It has not been possible to locate all of the hydrogen atoms (e.g. attached to oxygen) but these have been inferred by the need to balance charge.
Conflicts of interest
There are no conflicts to declare.
Acknowledgements
We thank the China Scholarship Council (CSC) for a PhD Scholarship to TX. The EPSRC Mass Spectrometry Service (Swansea, UK) and the EPSRC National X-ray Crystallography Service (Southampton) are thanked for data collection. CR also thanks the EPSRC (grant EP/S025537/1) for financial support.
References
-
(a) A. Chamas, H. Moon, J. Zheng, Y. Qiu, T. Tabassum, J. H. Jang, M. Abu-Omar, S. L. Scott and S. Suh, ACS Sustainable Chem. Eng., 2020, 8, 3494–3511 CrossRef CAS;
(b) M. Labet and W. Thielemans, Chem. Soc. Rev., 2009, 38, 3484–3504 RSC;
(c) O. Dechy-Cabaret, B. Martin-Vaca and D. Bourissou, Chem. Rev., 2004, 104, 6147–6176 CrossRef CAS PubMed;
(d) K. S. Anderson, K. M. Schreck and M. A. Hillmyer, Polym. Rev., 2008, 48, 85–108 CrossRef CAS;
(e) K. M. Nampoothiri, N. R. Nair and R. P. John, Bioresour. Technol., 2010, 101, 8493–8501 CrossRef PubMed.
-
(a) D. M. Homden and C. Redshaw, Chem. Rev., 2008, 108, 5086–5130 CrossRef CAS;
(b) C. Redshaw, Dalton Trans., 2016, 45, 9018–9030 RSC;
(c) O. Santoro and C. Redshaw, Coord. Chem. Rev., 2021, 448, 214173–214209 CrossRef CAS.
- L. Wang, S.-C. Roşca, V. Poirier, S. Sinbandhit, V. Dorcet, T. Roisnel, J.-F. Carpentier and Y. Sarazin, Dalton Trans., 2014, 43, 4268–4286 RSC.
- L. Wang, S. Fadlallah, C. Bellini, C. Orione, V. Dorcet, J.-F. Carpentier and Y. Sarazin, Organometallics, 2015, 34, 1321–1327 CrossRef CAS.
-
(a) T. Xing, T. J. Prior, K. Chen and C. Redshaw, Dalton Trans., 2021, 50, 4396–4407 RSC;
(b) T. Xing, T. J. Prior, M. R. J. Elsegood, N. V. Semikolenova, I. E. Soshnikov, K. Bryliakov, K. Chen and C. Redshaw, Catal. Sci. Technol., 2021, 11, 624–636 RSC;
(c) M. J. Walton, S. J. Lancaster, J. A. Wright, M. R. J. Elsegood and C. Redshaw, Dalton Trans., 2014, 43, 18001–18009 RSC;
(d) M. Frediani, D. Sémeril, A. Mariotti, L. Rosi, P. Frediani, L. Rosi, D. Matt and L. Toupet, Macromol. Rapid Commun., 2008, 29, 1554–1560 CrossRef CAS.
-
(a) A. Bilyk, J. W. Dunlop, A. K. Hall, J. M. Harrowfield, M. W. Hosseini, G. A. Koutsantonis, B. W. Skelton and A. H. White, Eur. J. Inorg. Chem., 2010, 2089–2105 CrossRef CAS;
(b) R. Kuriki, T. Kuwabara and Y. Ishii, Dalton Trans., 2020, 49, 12234–12241 RSC.
-
(a) P. D. Beer, M. G. B. Drew, P. B. Leeson and M. I. Ogden, J. Chem. Soc., Dalton Trans., 1995, 1273–1283 RSC;
(b) F. Arnaud-Neu, G. Barrett, D. Corry, S. Cremin, G. Ferguson, J. F. Gallagher, S. J. Harris, M. A. McKervey and M.-J. Schwing-Weill, J. Chem. Soc., Perkin Trans. 2, 1997, 575–580 RSC;
(c) A. F. D. de Namor, S. Chahine, D. Kowaiska, E. E. Castellano and O. E. Piro, J. Am. Chem. Soc., 2002, 124, 12824–12836 CrossRef PubMed;
(d) N. M. Buie, V. S. Talanov, R. J. Butcher and G. G. Talanova, Inorg. Chem., 2008, 47, 3549–3558 CrossRef CAS PubMed;
(e) H. J. Kim, S. H. Kim, J. H. Kim, L. N. Anh, J. H. Lee and C.-H. Lee, Tetrahedron Lett., 2009, 50, 2782–2786 CrossRef CAS;
(f) M. Bocheńska, J. Kulesza, J. Chojnacki, F. Arnaud-Neu and V. Hubscher-Bruder, J. Inclusion Phenom. Macrocyclic Chem., 2010, 68, 75–83 CrossRef;
(g) Y.-Y. Liu, C. Chen, J.-F. Ma and J. Yang, CrystEngComm, 2012, 14, 6201–6214 RSC;
(h) D. Over, X. Zeng, C. Bornholdt, J. Marrot and O. Reinaud, Inorg. Chem., 2013, 52, 14089–14095 CrossRef CAS PubMed;
(i) B. B. Adhikari, K. Ohto and M. P. Schramm, Chem. Commun., 2014, 50, 1903–1905 RSC;
(j) E. Lee, Y. Kim, J. Hen and K.-M. Park, Cryst. Growth Des., 2015, 15, 3556–3560 CrossRef CAS.
-
(a) C. Redshaw, Coord. Chem. Rev., 2003, 244, 45–70 CrossRef CAS;
(b) S. M. Taylor, S. Sanz, R. D. McIntosh, C. M. Beavers, S. J. Teat, E. K. Brechin and S. J. Dalgarno, Chem. – Eur. J., 2012, 18, 16014–16022 CrossRef CAS PubMed.
-
(a) C. Redshaw, D. Homden, D. L. Hughes, J. A. Wright and M. R. J. Elsegood, Dalton Trans., 2009, 1231–1242 RSC;
(b) A. Arbaoui, C. Redshaw, M. R. J. Elsegood, V. E. Wright, A. Yoshizawa and T. Yamato, Chem. – Asian J., 2010, 5, 621–633 CrossRef CAS PubMed.
-
(a) J. R. Fulton, P. B. Hitchcock, N. C. Johnstone and E. C. Y. Tam, Dalton Trans., 2007, 3360–3362 RSC;
(b) B. D. Rekken, T. M. Brown, M. M. Olmstead, J. C. Fettinger and P. P. Power, Inorg. Chem., 2013, 52, 3054–3062 CrossRef CAS PubMed.
- C. Redshaw, M. Rowan, L. Warford, D. M. Homden, A. Arbaoui, M. R. J. Elsegood, S. H. Dale, T. Yamato, C. P. Casas, S. Matsui and S. Matsuura, Chem. – Eur. J., 2007, 13, 1090–1107 CrossRef CAS PubMed.
-
(a) Z. Sun, Y. Zhao, O. Santoro, M. R. J. Elsegood, E. V. Bedwell, K. Zahra, A. Walton and C. Redshaw, Catal. Sci. Technol., 2020, 10, 1619–1639 RSC;
(b) O. Santoro, M. R. J. Elsegood, E. V. Bedwell, J. A. Pryce and C. Redshaw, Dalton Trans., 2020, 49, 11978–11996 RSC.
- Y. Li, K. Zhao, C. Feng, M. R. J. Elsegood, T. J. Prior, X. Sun and C. Redshaw, Dalton Trans., 2014, 43, 13612–13619 RSC.
- T. Xing, C. Jiang, M. R. J. Elsegood and C. Redshaw, Inorg. Chem., 2021 DOI:10.1021/acs.inorgchem.1c02192.
- O. Santoro, M. R. J. Elsegood, S. J. Teat, T. Yamato and C. Redshaw, RSC Adv., 2021, 11, 11304–11317 RSC.
-
(a) Q. Hu, S.-Y. Jie, P. Braunstein and B.-G. Lia, Chin. J. Polym. Sci., 2020, 38, 240–247 CrossRef CAS;
(b) M. A. Woodruff and D. W. Hutmacher, Prog. Polym. Sci., 2010, 35, 1217–1256 CrossRef CAS;
(c) T. Wu, Z. Wei, Y. Ren, Y. Yu, X. Leng and Y. Li, Polym. Degrad. Stab., 2018, 155, 173–182 CrossRef CAS;
(d) M. T. Hunley, N. Sari and K. L. Beers, ACS Macro Lett., 2013, 2, 375–379 CrossRef CAS;
(e) Z. Sun, Y. Zhao, O. Santoro, M. R. J. Elsegood, E. V. Bedwell, K. Zahra, A. Walton and C. Redshaw, Catal. Sci. Technol., 2020, 10, 1619–1639 RSC.
-
(a) C. Ludwig and M. R. Viant, Phytochem. Anal., 2010, 21, 22–32 CrossRef CAS PubMed;
(b) M. J. Walton, S. J. Lancaster and C. Redshaw, ChemCatChem, 2014, 6, 1892–1898 CrossRef CAS.
-
(a) B. Masci, J. Org. Chem., 2001, 66, 1497–1499 CrossRef CAS PubMed;
(b) B. Dhawan and C. D. Gutsche, J. Org. Chem., 1983, 48, 1536–1539 CrossRef CAS.
- T. Heidemann and S. Mathur, Eur. J. Inorg. Chem., 2014, 3, 506–510 CrossRef.
- S. C. Goel, M. Y. Chiang and W. E. Buhro, Inorg. Chem., 1990, 29, 4640–4646 CrossRef CAS.
- G. M. Sheldrick, Acta Crystallogr., Sect. A: Found. Adv., 2015, 71, 3–8 CrossRef PubMed.
- G. M. Sheldrick, Acta Crystallogr., Sect. C: Struct. Chem., 2015, 71, 3–8 Search PubMed.
- A. L. Spek, Acta Crystallogr., Sect. C: Struct. Chem., 2015, 71, 9–18 CrossRef CAS PubMed.
|
This journal is © The Royal Society of Chemistry 2021 |
Click here to see how this site uses Cookies. View our privacy policy here.