DOI:
10.1039/D1DT02681J
(Paper)
Dalton Trans., 2021,
50, 14864-14871
NIR emission of lanthanides for ultrasensitive luminescence manometry—Er3+-activated optical sensor of high pressure†
Received
11th August 2021
, Accepted 10th September 2021
First published on 11th September 2021
Abstract
Pressure is an important physical parameter and hence its monitoring is very important for different industrial and scientific applications. Although commonly used luminescent pressure sensors (ruby—Al2O3:Cr3+ and SrB4O7:Sm2+) allow optical monitoring of pressure in compressed systems (usually in a diamond anvil cell; DAC), their detection resolution is limited by sensitivity, i.e., pressure response in a form of the detected spectral shift. Here we report, a breakthrough in optical pressure sensing by developing an ultra-sensitive NIR pressure sensor (dλ/dP = 1.766 nm GPa−1). This luminescent manometer is based on the optically active YVO4:Yb3+–Er3+ phosphor material which exhibits the largest spectral shift as a function of pressure compared to other luminescent pressure gauges reported elsewhere. In addition, thanks to the locations of excitation and emission in the NIR range, the developed optical manometer allows high-pressure measurements (without spectral overlapping/interferences) of various luminescent organic and inorganic materials, which are typically excited and can emit in the UV-vis spectral ranges.
1. Introduction
High-pressure compression of materials can lead to various interesting alterations of their physicochemical properties, including shrinking of unit cell volume (shortening of bond lengths), structural symmetry variations, defect formation, the emergence of new structures/phases, formation of novel materials, etc.1–5 The development of noninvasive precise and accurate optical manometers has been stimulated by the challenging requirements of higher sensitivity, arising from the scientific research studies in diverse pressure regimes, from vacuum to high-pressure regions (over 100 GPa).6,7 The spectroscopic properties of materials under high-pressure conditions can be monitored by performing experiments in a diamond anvil cell (DAC) based on the assembly setup of a metal housing, two highly transparent diamond anvils, pressure transmitting medium (PTM), metal gasket and screws to squeeze and compress the material studied.8 For determination of the hydrostatic pressure in a DAC chamber, inorganic materials doped with lanthanide and d-block metal ions are usually used as optical manometers.6,7,9 Currently, at very high pressure (above 10–20 GPa), the sensitivities of the commonly used ruby (Al2O3:Cr3+; dλ/dP ≈ 0.35 nm GPa−1)8,10 and SrB4O7:Sm2+ (dλ/dP ≈ 0.25 nm GPa−1) pressure sensors11,12 may be fairly enough due to the hydrostatic limits of the commonly used PTM, leading to the occurrence of some pressure gradient in the chamber of DAC. However, in the relatively low pressure range (up to few GPa), where numerous phase transitions of soft, organic materials may occur, new manometers with higher sensitivity (large spectral shift) are continuously pursued in order to realize accurate pressure determination (resolve the similar pressure values in the investigated system) for phase transition detection, monitoring physical and chemical behaviour under high-pressure conditions, and so forth.
Lanthanide (Ln3+/2+) doped inorganic compounds are versatile functional materials, which are applied in diverse fields, such as bio-imaging, anti-counterfeiting, pressure and temperature sensing, modern lighting techniques, microscopy, etc.13–16 This is due to the specific spectroscopic features that originated from their ladder-like electronic structure, including multi-range absorption/emission (UV-vis-NIR); absence of autofluorescence; the forbidden (by Laporte selection rules) character of the intrinsic 4f–4f transitions, leading to long emission lifetimes;17 shielding of the 4f electrons by 5s and 5p ones, resulting in sharp emission/absorption lines; crystal-field effects, etc.18,19 The Stokes (down-shifting) and anti-Stokes type (up-conversion) emissions can be triggered by the use of different excitation light sources, from UV to NIR, depending on the host matrix type and the incorporated Ln3+/2+ ions.8,20,21 In the case of optical pressure sensing, the NIR excitation/emission may be very beneficial because it can eliminate some interference factors, namely fluorescence of diamonds and PTM; autofluorescence of particular organic/inorganic compounds studied; and most importantly intense emission of Cr3+ and Sm2+ in the visible range, which may sometimes overlap with the luminescence of optically active samples, thus hampering spectroscopic measurements under pressure.22 In fact, some recent reports showed good pressure sensitivity of NIR optical manometers, such as Er3+-doped YPO4 (dλ/dP ≈ 0.539 nm GPa−1)23 and NaBiF4 (dλ/dP ≈ −0.8 nm GPa−1)24 materials. In general, the pressure-induced variations of the electronic structure of Ln3+/2+ ions in compressed materials allow the determination of different spectroscopic parameters, such as the spectral shift of the emission bands, changes in intensity ratios, bandwidth, shortening of luminescence lifetimes, etc., which can be further utilized for optical manometry.
Up to now, to the best of our knowledge, the most sensitive optical manometer reported was BaLi2Al2SiN6:0.01Eu2+, which shows very good pressure sensitivity, i.e., dλ/dP = 1.58 nm GPa−1.25 In this work, we report an ultra-sensitive pressure sensor based on the YVO4:Yb3+–Er3+ luminescent material, operating in the NIR region (≈1500–1600 nm), based on the Stark components of the 4I13/2 → 4I15/2 transition of Er3+, exhibiting pressure-induced spectral shift dλ/dP = 1.766 nm GPa−1—a new record in pressure sensitivity. The developed optical manometer is especially beneficial for resolving similar pressure values under mild-pressure conditions (up to a few GPa), where the phase transitions of most organic compounds take place. Moreover, due to the possibility of NIR laser excitation and the location of the emission signal in the NIR region, using the developed sensor, one can avoid potential interferences of background autofluorescence of some organic species and inorganic compounds studied to effectively realize phase transition detection and physicochemical characterization under high-pressure conditions.
2. Results and discussion
2.1. Characteristics of the sensor material
The luminescent pressure sensor is based on inorganic, submicron-sized particles of YVO4 co-doped with 20 mol% of Yb3+ and 2 mol% of Er3+ ions. The exact elemental composition of the synthesized compound, determined by the inductively coupled plasma-optical emission spectroscopy (ICP-OES) method is: Y0.748Yb0.228Er0.024VO4. The particles crystallize in the structure of zircon-type tetragonal YVO4, the space group I41/amd (bulk modulus, B0 = 130(3) GPa), where the part of Y3+ ions is substituted with Yb3+ and Er3+ ions, with local point symmetry D4h. The material was synthesized using a hydrothermal approach and further calcination (≈1273 K), ensuring its high thermal stability and very good crystallinity of the final particles.26
Combining the abovementioned benefits with a relatively low phonon energy of the vanadate crystal lattice (≈900 cm−1), the lanthanide-doped YVO4 phosphors (luminophores) are generally considered as good and effective (down-shifting) emitters.27–32 Moreover, in the case of the investigated material YVO4:Yb3+–Er3+, due to the high absorption cross-section of Yb3+ and good emitting properties of Er3+ (with rich energy level structure), this compound is known as a good up-converting emitter upon 975 nm excitation.33 The synthesis details, structural and morphological characteristics, and upconverting (λex = 975 nm) luminescence properties (including its upconversion emission as a function of temperature, vacuum and excitation power) are given and discussed in detail in our previous study.26 Moreover, in our other report, we investigated the upconverting properties of the nano-sized (non-calcined) YVO4:Yb3+–Er3+ material under high-pressure conditions (up to ≈11 GPa).34 Importantly, in that report, using high-pressure luminescence spectroscopy, we have observed around 8 GPa phase transitions of the material that were also clearly documented by the others and confirmed with XRD and Raman spectroscopy (phase transition to a tetragonal, scheelite-type structure, space group I41/m, local point symmetry C4h, and B0 = 138(8) Gpa).35–37
2.2. High-pressure structural studies—Raman spectroscopy
First, it is worth noting that in this work, we have performed the spectroscopic measurements for the material studied up to ≈6.5 GPa, i.e., below the pressure value of the mentioned phase transition in order to avoid the potential nonmonotonic behavior of the determined spectroscopic parameters and ensure the reversibility of the monitored changes, which are important for pressure sensing applications. In order to confirm the structural stability of the investigated material and investigate the behavior of the phonon energies of the crystal lattice under high-pressure conditions, we have measured the Raman spectrum of the YVO4:Yb3+–Er3+ material in compression–decompression cycles. The spectra presented in Fig. 1 start from 600 cm−1 because in the lower energy region (smaller wavenumber values), the artificial luminescence contributions biased the recorded spectra obscuring the low-intensity and low-energy Raman modes, typically observed for the pure (undoped) YVO4 materials at around 154, 259, 376 and 487 cm−1.37,38 However, from the luminescence point of view, the most important are the recorded high-energy phonon modes that are responsible for the phonon-assisted energy transfer and luminescence quenching processes. In all spectra, three high-energy bands are clearly observed, being initially located at around 819, 840 and 894 cm−1. These bands correspond to the Raman active modes ν3(Bg), ν3(Eg) and ν1(Ag), respectively.37 With increasing pressure, all the mentioned peaks shift toward higher energies (higher wavenumber values) with shift rates of about 5.40, 5.08 and 5.55 cm−1 GPa−1, respectively, which can be clearly observed in Fig. 1. Importantly, the spectral shifts observed with pressure are fully reversible as the peak centroids determined during the decompression process (the empty symbols in Fig. 1b) are consistent with the data from the compression cycle. The decreasing signal-to-noise ratio and broadening of the peaks with pressure are typical, reversible effects observed in the Raman spectra of the compressed materials, which are related to the decreasing crystallinity level and increasing strains of the crystals, in respect to the material studied at ambient conditions.39 The obtained results agree with the literature data reported for pure YVO4 materials.37,38
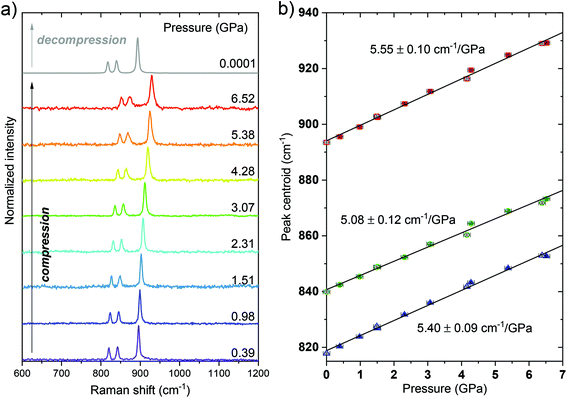 |
| Fig. 1 (a) Raman spectra and (b) the determined energies of the observed high-energy phonon modes as a function of pressure; the continuous lines represent the applied linear fits; the filled and empty symbols are the data points determined based on the measurements performed during the compression and decompression cycles, respectively. | |
2.3. High-pressure luminescence studies
Fig. 2a presents the down-shifting emission spectra of the material studied in the NIR range, corresponding to the 4I13/2 → 4I15/2 radiative transition of Er3+ (centered around 1550 nm), as measured under the high-pressure conditions upon the 975 nm laser excitation. The observed emission is a consequence of the effective excitation of Yb3+ ions in resonance to the 2F7/2 → 2F5/2 transition, a subsequent resonant energy transfer to the excited level 4I11/2 of Er3+ and further non-radiative relaxation to the emitting level 4I13/2 of Er3+ (see the energy level diagram in Fig. 3). It is clearly seen from the spectra that the high-pressure compression of the material induces a spectral shift of the crystal-field components (Stark sub-levels) of the 4I13/2 → 4I15/2 transition of Er3+ as well as changes the shape of the spectra, i.e., the relative intensities of the components and the level of their overlapping and spectral width. Two main effects are responsible for the observed changes, namely: (I) pressure-enhanced nephelauxetic effect, i.e. increased covalency of bonding in the compressed material, resulting in a decreasing energy separation between the ground and excited levels of Er3+ that leads to the observed red-shift of the crystal field components on pressure; (II) pressure-enhanced crystal-field strength, due to stronger interactions between Er3+ ions and shorter interionic distances (Er3+–O2−) in the compressed structure, which leads to larger splitting of the observed Stark sub-levels.8,25,40,41 Due to the fact, that these two effects are superimposed, in general, the low-energy emission lines exhibit larger spectral shifts with pressure compared to the high-energy Stark sub-levels of the transition (see the crystal-field splitting scheme in Fig. 3). In fact, such a situation occurs in our case, as clearly observed in Fig. 2b, demonstrating the spectral positions of the peak centroids for different crystal-field components and the shift rates determined on pressure (by linear fits). The high-energy Stark sub-level located around 1500 nm reveals a significant blue-shift (dλ/dP = −0.740 nm GPa−1), whereas the low-energy peaks located around 1600 nm exhibit large red-shifts (dλ/dP = 1.282 and 1.766 nm GPa−1). The determined shift rates, currently classify the developed sensor as the most sensitive luminescent manometer (see Table 1). The details, i.e., fitting parameters for the analysed seven crystal-field components are given in Table S1 in the ESI data.† Whereas the energies for the determined peak centroids as a function of pressure and the calculated shift rates (cm−1 GPa−1) are given in Fig. S1.† Please note that we correlated with pressure only those peaks that were present in the whole measured pressure range and did not overlap too much with each other, allowing the determination of their centroids. In addition, based on the recorded luminescence decay curve (at ambient conditions) presented in Fig. S2† (λex = 975 nm; λem = 1550 nm), we have determined the effective lifetime
for the discussed NIR emission of Er3+, i.e., τ ≈ 1.42 ms, which defines the temporal response of the sensor.
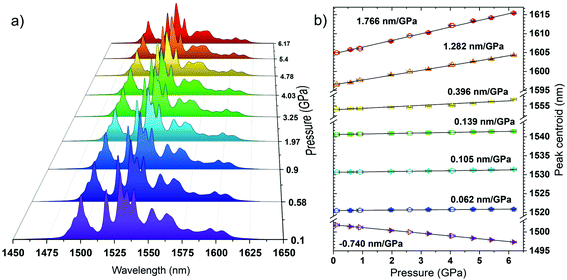 |
| Fig. 2 (a) The NIR luminescence spectra of the YVO4:Yb3+–Er3+ sample (λex = 975 nm), measured as a function of pressure. (b) Determined spectral positions of the seven Stark sub-levels, corresponding to the 4I13/2 → 4I15/2 transition of Er3+ as a function of pressure; the continuous lines represent the applied linear fits; the filled and empty symbols are the data points determined based on the measurements performed during the compression and decompression cycles, respectively. | |
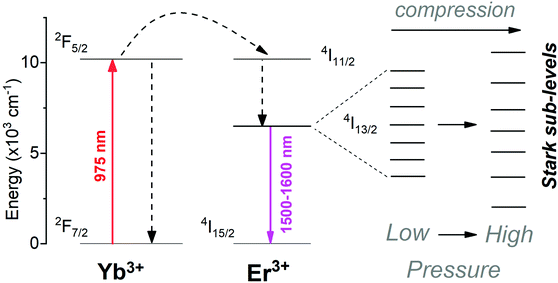 |
| Fig. 3 Energy level diagram representing the radiative and nonradiative processes occurring in the studied Yb3+–Er3+ system, emphasizing the crystal field splitting of the 4I13/2 level of Er3+ at ambient and high-pressure conditions, resulting in the formation of several Stark sub-levels for the 4I13/2 → 4I15/2 transition. | |
Table 1 Comparison of high-pressure sensitivities of different luminescent manometers used for optical pressure sensing
Host |
Emitting ion |
Transitions |
λ (nm) |
T-shift (nm K−1) |
Sensitivity line shift (nm GPa−1) |
Ref. |
YVO4 |
Er3+ |
4I13/2 → 4I15/2 (stark) |
1605 |
−5.16 × 10−3 |
1.766 |
This work |
4I13/2 → 4I15/2 (stark) |
1596 |
−4.04 × 10−3 |
1.282 |
4I13/2 → 4I15/2 (stark) |
1502 |
3.63 × 10−3 |
−0.740 |
YPO4 |
Er3+ |
4I13/2 → 4I15/2 (stark) |
1589 |
−1.78 × 10−3 |
0.539 |
23
|
Al2O3 (ruby) |
Cr3+ |
2E → 4A2 |
694 |
≈7 × 10−3 |
0.365 |
10,44 and 48 |
YAlO3 |
Cr3+ |
2E → 4A2 |
723 |
7.6 × 10−3 |
0.70 |
48
|
YF3 |
Er3+ |
4F9/2 → 4I15/2 (stark) |
665 |
−3 × 10−4 |
0.1855 |
49
|
NaBiF4 |
Er3+ |
4I13/2 → 4I15/2 (stark) |
1503 |
— |
−0.8 |
24
|
YAlO3 |
Nd3+ |
4F3/2 → 4I9/2 (stark) |
875 |
1 × 10−6 |
−0.13 |
48
|
Gd3Sc2Ga3O12 |
Nd3+ |
4F3/2 → 4I9/2 (stark) |
935 |
— |
∼0.632 |
50
|
Y3Al5O12 |
Eu3+ |
5D0 → 7F1 |
591 |
−5.4 × 10−4 |
0.197 |
51
|
EuPO4 |
Eu3+ |
5D0 → 7F0 |
580 |
— |
∼0.27 |
52
|
Y3Al5O12 |
Sm3+ |
4G5/2 → 6H7/2 (stark) |
618 |
2.3 × 10−4 |
0.30 |
53
|
SrFCl |
Sm2+ |
5D0 → 7F0 |
690 |
−2.3 × 10−3 |
1.11 |
54
|
SrB4O7 |
Sm2+ |
5D0 → 7F0 |
685 |
−1 × 10−4 |
0.255 |
55
|
SrB2O4 |
Sm2+ |
5D0 → 7F0 |
685 |
−1 × 10−4 |
0.244 |
56
|
BaLi2Al2Si2N6 |
Eu2+ |
5d → 4f |
532 |
— |
1.58 |
25
|
KMgF3 |
Eu2+ |
5d → 4f |
360 |
— |
∼0.13 |
57
|
CeN-PVDF |
Ce3+ |
5d → 4f |
327 |
— |
0.28 |
58
|
CeS-PVDF |
Ce3+ |
5d → 4f |
340 |
— |
0.1 |
58
|
LaPO4 |
Tm3+ |
1G4 → 3H6 |
475 |
−2 × 10−3 |
0.1 |
59
|
3H4 → 3H6/1G4 → 3H6 |
800/475 |
— |
8% (band ratio) |
Y6Ba4(SiO4)6F2 |
Ce3+ |
2DJ → 2FJ (5d → 4f) emission |
466 |
— |
0.63 |
8
|
1.5% (FWHM) |
2FJ → 2DJ (4f → 5d) excitation |
342 |
— |
2.5% (FWHM) |
SrF2 |
Er3+ |
4F9/2 → 4I15/2 |
653 |
— |
7.7% (lifetime) |
60
|
4S3/2 → 4I15/2 |
538 |
6.4% (lifetime) |
2H11/2 → 4I15/2 |
516 |
6.2% (lifetime) |
The non-normalized emission spectra presented in Fig. S3 and S4† (recorded during compression and decompressions runs, respectively) show how the intensity of the bands changes with pressure. It can be observed that around 6 GPa, the intensity of the bands is approximately a half of the intensity of the initial spectrum. Importantly, these changes are reversible as the intensity is almost completely recovered after the decompression process. Here, it is worth noting that, in general, there are three main mechanisms responsible for luminescence quenching of lanthanide ions under high-pressure conditions, namely: (I) pressure-induced plastic deformations, leading to the formation of crystal defects; (II) multi-phonon relaxation enhanced with pressure, due to the increasing phonon energies of the compressed host matrices; and (III) improved cross-relaxation processes, as a result of decreasing interionic distances in the compressed materials.8,40–42 However, only the first mechanism may have a minor contribution to the signal deterioration with pressure (because after the decompression process, the signal intensity is recovered roughly in 90%, as can be observed in Fig. S4†) and the second and third mechanisms are not expected to occur in the system studied. This is due to the following reasons: (I) the observed intensity changes are almost completely reversible; (II) the considered NIR emission occurs from the lowest excited level, situated around 6500 cm−1 above the ground level, and the highest-energy phonon mode has an energy of around 930 cm−1 (at ≈6.5 GPa), so for the multi-phonon relaxation the required number of phonons is 7 (in general, the maximum number of phonons leading to the effective multiphonon relaxation is considered to be 3–5);26 and (III) below the energy of the emitting level 4I13/2, there are no other lower lying excited states in the system studied (neither in Yb3+ nor in Er3+ ions), so the cross-relaxation processes should not affect that level. That is why the observed emission is not significantly quenched at pressure (compared to other luminescent materials) and the probable mechanisms responsible for quenching may be related to the decreasing crystallinity and increasing strains in the crystals, as well as the increased energy migration to traps and defect states, leading to the less efficient Yb3+ → Er3+ energy transfer and lower probability of radiative relaxation from the excited state 4I13/2 in the compressed material.
2.4. Temperature effect on luminescence properties
In developing new luminescent pressure-sensors, one should not neglect the impact of temperature on the spectral position of the emission line used for sensing purposes, i.e., the so-called temperature correction or thermal drift. This is because more and more experiments are performed simultaneously under extreme conditions of both pressure and temperature (the compressed and heated-cooled systems). Hence, the optimal sensor should exhibit a negligible temperature-dependence or only small (compared to the corresponding shift in pressure) and well-defined spectral shifts.41,43 Fortunately, for the emission lines of the developed sensor, the determined thermal drifts are small and linear, e.g., dλ/dT = −5.16 × 10−3, −4.04 × 10−3 and 3.63 × 10−3 nm K−1, for the most pressure-sensitive Stark sub-levels located initially at ≈1605, 1596 and 1502 nm, respectively (see Fig. 4 and S5†). Importantly, the determined temperature drifts are smaller than that in the commonly used pressure sensor—ruby (dλ/dT = 7 × 10−3 nm K−1)44 and they are similar to those of other reported luminescent pressure sensors (see Table 1). In addition, the energies for the determined peak centroids as a function of temperature and the calculated shift rates (cm−1 K−1) are given in Fig. S6.†
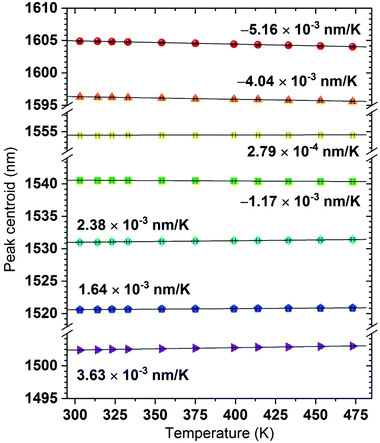 |
| Fig. 4 Determined spectral positions, i.e., peak centroids of the Stark sub-levels (initially located at ≈1502, 1521, 1531, 1541, 1554, 1596 and 1605 nm) as a function of temperature, corresponding to the 4I13/2 → 4I15/2 transition of Er3+ in the YVO4:Yb3+–Er3+ material (λex = 975 nm). | |
Besides, the often underestimated thermal effect is signal deterioration at elevated temperatures, which may affect the precision and accuracy of pressure sensing under extreme conditions of both factors. The main mechanism responsible for luminescence quenching of lanthanide ions at elevated temperatures is multiphonon relaxation as the phonon energy increases with temperature as well.45–47 However, in the case of the developed sensor material, the required number of phonons is too high to allow effective multiphonon relaxation (as was described in the previous paragraph concerning signal intensity changes with pressure), that is why the mentioned quenching mechanism should have a negligible contribution. The analysis of signal intensity as a function of temperature confirms this assumption, as the absolute luminescence intensity almost does not change with temperature, as can be observed in the emission spectra presented in Fig. S5.†
3. Conclusions
In this work, we presented a development of the ultrasensitive (dλ/dP = 1.766 nm GPa−1), optical pressure sensor (current leader in sensitivity) based on the luminescent lanthanide-doped material YVO4:Yb3+–Er3+, which is five times more sensitive than the commonly used ruby-based sensors. High-sensitivity of the sensor was achieved by monitoring its NIR emission, namely the crystal-field components (Stark sub-levels) of the 4I13/2 → 4I15/2 transition of Er3+, centred around 1550 nm. Another benefit of this luminescent manometer is the possibility of excitation in the NIR range (thanks to energy transfer Yb3+ → Er3+), utilizing commercially available and low-cost diode lasers, emitting at around 975/980 nm. It is worth noting that the applied low-energy NIR light does not damage and easily penetrates many materials/media. Moreover, in contrast to blue-green lasers (used for ruby and Sm2+ excitation), NIR light does not produce background autofluorescence, because, in general, most of the organic species and inorganic compounds do not produce luminescence upon excitation in the NIR spectral range. Importantly, thanks to the high sensitivity of the developed sensor material, it allows very accurate pressure sensing (depending on the resolution of the detection system, pressure range studied and the pressure transmitting medium used). The developed sensor might be especially useful for the relatively low-pressure sensing (up to a few GPa), especially in the case of the experiments performed with soft, organic materials, where multiple phase transitions may occur in a narrow pressure range. In such a case, a very sensitive material (e.g. showing large spectral shift) is required to resolve the similar pressure values of the studied system, allowing the detection of phase transitions and monitoring the changes of various physical and chemical parameters during the compression–decompression cycles.
4. Experimental section
As was already mentioned, the detailed synthesis protocol of the material studied can be found in our previous article.26 Raman spectra were recorded in backscattering geometry by using a Renishaw InVia confocal micro-Raman system, using a grating with 1800 grooves per mm (spectral resolution better than 1 cm−1) and a power-controlled 785 nm laser diode. The laser beam was focused by using an Olympus ×20 SLMPlan N long working distance objective. Emission spectra in the NIR range were recorded by using an Andor Shamrock 500 spectrometer equipped with an InGaAs CCD camera (Andor). The excitation light source was a tunable CW Ti:sapphire laser (Spectra Physics 3900-S) adjusted at 975 nm and pumped with a 15 W 532 nm laser (Spectra Physics Millenia). Emission decay curve was recorded using a 200 MHz LeCroy WS424 oscilloscope coupled with a photomultiplier tube (PMT)—Hamamatsu H10330C-75—and a tunable EKSPLA/NT342/3/UVE 10 ns pulsed laser, i.e., optical parametric oscillator (OPO), operating at 10 Hz repetition rate. Luminescence measurements under high-pressure conditions were performed in a diamond anvil cell (DAC), using a back illuminated configuration with 180° detection geometry. The laser beam was focused on the sample placed in a gasket hole and the emission signal was collected from the opposite side of the DAC.
The DAC device was made at Universität Paderborn, in Germany. The culete size (diameter) of the diamond anvils was 400 μm. The pressure inside the DAC chamber was adjusted with four metal screws. The metal gasket used for DAC was made of a stainless-steel sheet, with a thickness of 200 μm, which was pre-indented down to ≈70 μm (sample thickness) and then a hole of size ≈100 μm was made with an electro-driller device. The sample and a single micron-sized ruby ball (<10 μm in diameter) were loaded in the DAC chamber. Subsequently, the pressure transmitting medium (solvent system of methanol/ethanol/water = 16
:
3
:
1(vol.)) was then filled in the DAC chamber to maintain hydrostatic conditions during the compression process. The pressure values were determined with a ruby R1 fluorescence line shift, using 532 nm laser excitation and standard ruby calibration curve available at http://kantor.50webs.com/ruby.htm.
Conflicts of interest
The authors declare no competing financial interest.
Acknowledgements
This work was supported by the Polish National Science Centre, grant no. 2016/23/D/ST4/00296 and 2018/31/N/ST5/00636, and by the grant no. POWR.03.02.00-00-I023/17 and POWR.03.02.00-00-i020/17 co-financed by the European Union through the European Social Fund under the Operational Program Knowledge Education Development.
References
- J. Sun, Q. Li, H. Zhu, Z. Liu, K. Lin, N. Wang, Q. Zhang, L. Gu, J. Deng, J. Chen and X. Xing, Adv. Mater., 2020, 32, 1–6 Search PubMed
.
- F. Bai, K. Bian, X. Huang, Z. Wang and H. Fan, Chem. Rev., 2019, 119, 7673–7717 CrossRef CAS PubMed
.
- L. Zhang, C. Liu, L. Wang, C. Liu, K. Wang and B. Zou, Angew. Chem., Int. Ed., 2018, 57, 11213–11217 CrossRef CAS PubMed
.
- S. Liu, S. Sun, C. K. Gan, A. G. Del Águila, Y. Fang, J. Xing, T. Thu Ha Do, T. J. White, H. Li, W. Huang and Q. Xiong, Sci. Adv., 2019, 5, 1–11 Search PubMed
.
- Y. N. Zhuravlev and V. V. Atuchin, Sensors, 2021, 21, 3644 CrossRef CAS PubMed
.
- S. M. Peak and A. N. Watkins, ACS Appl. Nano Mater., 2020, 3, 9813–9821 CrossRef CAS
.
- G. Pacchioni, Nat. Rev. Mater., 2021, 6, 108 CrossRef CAS
.
- M. Runowski, P. Woźny, N. Stopikowska, Q. Guo and S. Lis, ACS Appl. Mater. Interfaces, 2019, 11, 4131–4138 CrossRef CAS PubMed
.
- J. W. Gregory, K. Asai, M. Kameda, T. Liu and J. P. Sullivan, Proc. Inst. Mech. Eng., Part G, 2008, 222, 249–290 CrossRef CAS
.
- H. K. Mao, J. Xu and P. M. Bell, J. Geophys. Res., 1986, 91, 4673–4676 CrossRef CAS
.
- C. Zhao, H. Li, Y. Wang, J. Jiang and Y. He, High Press. Res., 2017, 37, 18–27 CrossRef CAS
.
- Q. Jing, Q. Wu, L. Liu, J. Xu, Y. Bi, Y. Liu, H. Chen, S. Liu, Y. Zhang, L. Xiong, Y. Li and J. Liu, J. Appl. Phys., 2013, 113, 023507 CrossRef
.
-
C. D. S. Brites, A. Millán and L. D. Carlos, in Handbook on the Physics and Chemistry of Rare Earths, 2016, vol. 49, pp. 339–427 Search PubMed
.
- R. Marin, D. Jaque and A. Benayas, Nanoscale Horiz., 2021, 6, 209–230 RSC
.
- H. Tang, M. F. Zhang, S. J. Zhang, Y. J. Feng, F. Li and T. R. Shrout, J. Eur. Ceram. Soc., 2013, 33, 2491–2497 CrossRef CAS
.
- Z. Xia, Y. Zhang, M. S. Molokeev and V. V. Atuchin, J. Phys. Chem. C, 2013, 117, 20847–20854 CrossRef CAS
.
- K. Binnemans, Chem. Rev., 2009, 109, 4283–4374 CrossRef CAS PubMed
.
- F. Auzel, Chem. Rev., 2004, 104, 139–173 CrossRef CAS PubMed
.
- M. D. Dramićanin, Methods Appl. Fluoresc., 2016, 4, 042001 CrossRef PubMed
.
- C. S. Lim, A. S. Aleksandrovsky, M. S. Molokeev, A. S. Oreshonkov, D. A. Ikonnikov and V. V. Atuchin, Dalton Trans., 2016, 45, 15541–15551 RSC
.
- Y. G. Denisenko, V. V. Atuchin, M. S. Molokeev, N. Wang, X. Jiang, A. S. Aleksandrovsky, A. S. Krylov, A. S. Oreshonkov, A. E. Sedykh, S. S. Volkova, Z. Lin, O. V. Andreev and K. Müller-Buschbaum, J. Mater. Sci. Technol., 2021, 76, 111–121 CrossRef
.
-
R. Reisfeld and C. K. Jørgensen, Lasers and Excited States of Rare Earths, Springer Berlin Heidelberg, Berlin, 1977, vol. 1, DOI:10.1007/978-3-642-66696-4
.
- M. Runowski, P. Woźny and I. R. Martín, J. Mater. Chem. C, 2021, 9, 4643–4651 RSC
.
- M. A. Antoniak, S. J. Zelewski, R. Oliva, A. Żak, R. Kudrawiec and M. Nyk, ACS Appl. Nano Mater., 2020, 3, 4209–4217 CrossRef CAS
.
- Y. Wang, T. Seto, K. Ishigaki, Y. Uwatoko, G. Xiao, B. Zou, G. Li, Z. Tang, Z. Li and Y. Wang, Adv. Funct. Mater., 2020, 30, 2001384 CrossRef CAS
.
- M. Runowski, P. Woźny, S. Lis, V. Lavín and I. R. Martín, Adv. Mater. Technol., 2020, 5, 1901091 CrossRef CAS
.
- I. E. Kolesnikov, A. A. Kalinichev, M. A. Kurochkin, E. V. Golyeva, A. S. Terentyeva, E. Y. Kolesnikov and E. Lähderanta, Sci. Rep., 2019, 9, 2043 CrossRef CAS PubMed
.
- I. E. Kolesnikov, E. V. Golyeva, A. A. Kalinichev, M. A. Kurochkin, E. Lähderanta and M. D. Mikhailov, Sens. Actuators, B, 2017, 243, 338–345 CrossRef CAS
.
- G. Chen, N. A. Stump, R. G. Haire, J. R. Peterson and M. M. Abraham, J. Phys. Chem. Solids, 1992, 53, 1253–1257 CrossRef CAS
.
- G. Jia, Y. Song, M. Yang, Y. Huang, L. Zhang and H. You, Opt. Mater., 2009, 31, 1032–1037 CrossRef CAS
.
- Y. Zuo, W. Ling and Y. Wang, J. Lumin., 2012, 132, 61–63 CrossRef CAS
.
- R. J. Wiglusz, A. Bednarkiewicz and W. Strek, Inorg. Chem., 2012, 51, 1180–1186 CrossRef CAS PubMed
.
- M. Kumar Mahata, T. Koppe, K. Kumar, H. Hofsäss and U. Vetter, Sci. Rep., 2016, 6, 36342–36352 CrossRef CAS PubMed
.
- P. Woźny, M. Runowski and S. Lis, J. Lumin., 2019, 209, 321–327 CrossRef
.
- J. Ruiz-Fuertes, O. Gomis, S. F. León-Luis, N. Schrodt, F. J. Manjón, S. Ray, D. Santamaría-Pérez, J. A. Sans, H. M. Ortiz, D. Errandonea, C. Ferrer-Roca, A. Segura, D. Martínez-García, V. Lavín, U. R. Rodríguez-Mendoza and A. Muñoz, Nanotechnology, 2016, 27, 025701 CrossRef CAS PubMed
.
- X. Wang, I. Loa, K. Syassen, M. Hanfland and B. Ferrand, Phys. Rev. B: Condens. Matter Mater. Phys., 2004, 70, 064109 CrossRef
.
- F. J. Manjón, P. Rodríguez-Hernández, A. Muñoz, A. H. Romero, D. Errandonea and K. Syassen, Phys. Rev. B: Condens. Matter Mater. Phys., 2010, 81, 075202 CrossRef
.
- A. Jayaraman, G. A. Kourouklis, G. P. Espinosa, A. S. Cooper and L. G. Van Uitert, J. Phys. Chem. Solids, 1987, 48, 755–759 CrossRef CAS
.
- M. Runowski, A. Bartkowiak, M. Majewska, I. R. Martín and S. Lis, J. Lumin., 2018, 201, 104–109 CrossRef CAS
.
-
K. L. Bray, M. Glasbeek, H. Kunkely and A. Vogler, Transition Metal and Rare Earth Compounds Excited States, Transitions, Interactions I, ed. H. Yersin, Springer, New York, 2001 Search PubMed
.
-
T. Tröster, in Handbook on the Physics and Chemistry of Rare Earths, ed. K. A. Gschneidner, J.-C. G. Bünzli and V. K. Pecharsky, Elsevier, North-Holland, 2003, vol. 33, pp. 515–589 Search PubMed
.
- M. D. Wisser, M. Chea, Y. Lin, D. M. Wu, W. L. Mao, A. Salleo and J. a Dionne, Nano Lett., 2015, 15, 1891–1897 CrossRef CAS PubMed
.
-
M. Runowski, in Handbook of Nanomaterials in Analytical Chemistry, ed. C. M. Hussain, Elsevier, 2020, pp. 227–273 Search PubMed
.
- F. Datchi, A. Dewaele, P. Loubeyre, R. Letoullec, Y. Le Godec and B. Canny, High Press. Res., 2007, 27, 447–463 CrossRef CAS
.
- R. G. Geitenbeek, H. W. De Wijn and A. Meijerink, Phys. Rev. Appl., 2018, 10, 1 Search PubMed
.
- M. Suta and A. Meijerink, Adv. Theory Simul., 2020, 3, 2000176 CrossRef CAS
.
- T. P. Swieten, D. Yu, T. Yu, S. J. W. Vonk, M. Suta, Q. Zhang, A. Meijerink and F. T. Rabouw, Adv. Opt. Mater., 2021, 9, 2001518 CrossRef
.
- J. D. Barnett, S. Block and G. J. Piermarini, Rev. Sci. Instrum., 1973, 44, 1–9 CrossRef
.
- S. Goderski, M. Runowski, P. Woźny, V. Lavín and S. Lis, ACS Appl. Mater. Interfaces, 2020, 12, 40475–40485 CrossRef CAS PubMed
.
- S. F. León-Luis, J. E. Muñoz-Santiuste, V. Lavín and U. R. Rodríguez-Mendoza, Opt. Express, 2012, 20, 10393 CrossRef PubMed
.
- H. Arashi and M. Ishigame, Jpn. J. Appl. Phys., 1982, 21, 1647–1649 CrossRef CAS
.
- G. Chen, J. Hölsä and J. R. Peterson, J. Phys. Chem. Solids, 1997, 58, 2031–2037 CrossRef CAS
.
- N. J. Hess and G. J. Exarhos, High Press. Res., 1989, 2, 57–64 CrossRef
.
- Y. R. Shen and W. B. Holzapfel, Phys. Rev. B: Condens. Matter Mater. Phys., 1995, 51, 15752–15762 CrossRef CAS PubMed
.
- F. Datchi, R. LeToullec and P. Loubeyre, J. Appl. Phys., 1997, 81, 3333–3339 CrossRef CAS
.
- M. Runowski, P. Woźny, V. Lavín and S. Lis, Sens. Actuators, B, 2018, 273, 585–591 CrossRef CAS
.
- J. Barzowska, T. Lesniewski, S. Mahlik, H. J. Seo and M. Grinberg, Opt. Mater., 2018, 84, 99–102 CrossRef CAS
.
- C. Hernandez, S. K. Gupta, J. P. Zuniga, J. Vidal, R. Galvan, M. Martinez, H. Guzman, L. Chavez, Y. Mao and K. Lozano, Sens. Actuators, A, 2019, 298, 111595 CrossRef CAS
.
- M. Runowski, A. Shyichuk, A. Tymiński, T. Grzyb, V. Lavín and S. Lis, ACS Appl. Mater. Interfaces, 2018, 10, 17269–17279 CrossRef CAS PubMed
.
- M. Runowski, J. Marciniak, T. Grzyb, D. Przybylska, A. Shyichuk, B. Barszcz, A. Katrusiak and S. Lis, Nanoscale, 2017, 9, 16030–16037 RSC
.
Footnote |
† Electronic supplementary information (ESI) available: Fitting parameters; determined spectral positions of Stark sublevels for Er3+ 4I13/2 → 4I15/2 transition; energies of the peak centroids; luminescence decay curve; non-normalized spectra as a function of pressure and temperature. See DOI: 10.1039/d1dt02681j |
|
This journal is © The Royal Society of Chemistry 2021 |
Click here to see how this site uses Cookies. View our privacy policy here.