DOI:
10.1039/D1DT01970H
(Paper)
Dalton Trans., 2021,
50, 12843-12849
Heavy-element–ligand covalence: ligand noninnocence in molybdenum and tungsten Viking-helmet Corroles†
Received
15th June 2021
, Accepted 19th August 2021
First published on 2nd September 2021
Abstract
Extensive DFT calculations with several exchange–correlation functionals indicate that molybdenum-dichlorido Viking helmet corroles are noninnocent with significant MoIV-corrole˙2− character. The effect is mediated by a Mo(4d)-corrole(π) orbital interaction similar to that postulated for MnCl, FeCl and FeNO corroles. The effect also appears to operate in tungsten-dichlorido corroles but is weaker relative to that for Mo. In contrast, MoO triarylcorroles do not exhibit a significant degree of corrole radical character. Furthermore, the Soret absorption maxima of a series of MoCl2 tris(para-X-phenyl)corrole derivatives were found to redshift dramatically with increasing electron-donating character of the para substituent X, essentially clinching the case for a noninnocent macrocycle in MoCl2 corroles.
Introduction
Over a half-century ago, the Danish chemist C. K. Jørgensen defined a noninnocent ligand as one that leaves the oxidation state of the coordinated atom uncertain or debatable.1 For ligands involving extended π-systems, ligand noninnocence typically entails partial oxidation and reduction of the π-system, i.e., π-radical character.2,3 The phenomenon has been widely studied for 3d transition metals, perhaps most famously for the C–H-activating Compound I intermediate of cytochrome P450,4,5 but far less so for 4d, 5d, and f-element systems.6–8 The rarity of well-characterized, noninnocent 4d and 5d metal systems reflects in part the rarity of stable, paramagnetic complexes involving these elements (which in turn reflects their preference for low-spin states), robbing magnetic resonance methods such as paramagnetic NMR and EPR spectroscopy of the ability to characterize the phenomenon by probing molecular spin densities.9–16 It is against this backdrop that we have chosen to reexamine paramagnetic molybdenum-17 and tungsten-18 dichlorido “Viking-helmet” corroles with density functional theory methods for possible signs of a noninnocent corrole (Scheme 1; see also Notes).
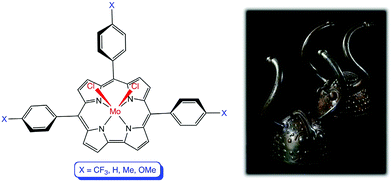 |
| Scheme 1 Molybdenum-dichlorido “Viking-helmet” corroles. Right: Bronze Age horned helmets from Veksø, Denmark. | |
In recent years, metallocorroles have provided many examples of well-characterized noninnocent systems.19–22 Key examples include MnCl,23 FeCl,9–15 FeNO,24–27 Fe2(μ-O),28 Co-py29/DMSO30 (py = pyridine), Co-PPh3,31 and Cu32–40 corrole derivatives. It is worth noting that all these involve first-row transition metals. In contrast, the overwhelming majority of 4d and 5d transition metal corroles are thought to involve an innocent corrole macrocycle.20,41 That said, both PtIV-aryl-corrole3− (ref. 42) and PtIV-aryl-corrole˙2− derivatives43 are known, as are simple ZnII-corrole˙2− radical species;44 the metal oxidation states in these systems, however, are not in doubt so these, according to the definition above, do not qualify as noninnocent. Silver corroles present a more interesting scenario: while simple, relatively planar Ag triarylcorroles, like their Au counterparts, are thought to be innocent, highly saddled Ag β-octabromo-meso-triarylcorroles, like their Cu counterparts,32–40 are thought to harbor noninnocent macrocycles.45,46
The assignment of an innocent or noninnocent description for a given system can be a tricky proposition and misassignments abound in the literature. In our own work on metallocorroles, we have regularly used half a dozen techniques as physicochemical probes of ligand noninnocence and advocated the use of at least three such probes for a credible electronic-structural assignment.22 Herein, we report three lines of evidence strongly supporting a noninnocent description for MoCl2 Viking-helmet corroles: (i) an existing X-ray structure, whose implications for ligand noninnocence have not been realized until now, and optimized DFT geometries, (ii) DFT spin density profiles, and (iii) in situ electronic absorption spectra.
Results and discussion
Molecular structure
High-quality X-ray structures commonly signal a noninnocent corrole in the form of characteristic bond length alternations within and around the bipyrrole unit of the macrocycle.22 As it happens, the reported X-ray structure of Mo[TpOCH3PC]Cl2 [where TpOCH3PC3− refers to the trianion of meso-tris(4-methoxyphenyl)corrole; CSD code: NEMMAW; Fig. 1] is indeed of high quality and shows exactly such a bond length alternation (although the point was not remarked upon in the original report).17 Somewhat less clearly, a similar bond length alternation is also observed in the X-ray structure of an electron-rich WCl2-triarylcorrole (CSD: WUNZUC; Fig. 2).18 In contrast, the X-ray structure of Mo[TPC]O (CSD: YEBTIJ; TPC3− = meso-triphenylcorrolato; Fig. 1)47 does not evince a similar bond length alternation, while terminal tungsten-oxo corroles, to our knowledge, have not been reported. Interestingly, while CrO triarylcorroles generally do not exhibit skeletal bond length alternations,48 an electron-rich CrO-triarylcorrole with a 10-p-hydroxyphenyl substituent does, emphasizing the subtlety of the matter.49
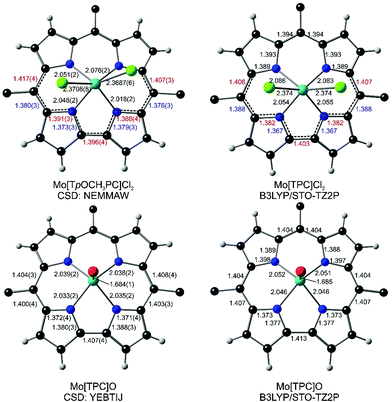 |
| Fig. 1 Selected distances in the X-ray structures of Mo[TpOCH3PC]Cl2 and Mo[TPC]O and the DFT (B3LYP/STO-TZ2P) optimized geometries of Mo[TPC]Cl2 and Mo[TPC]O. Parts of the corrole skeleton exhibiting bond length alternation are indicated with dashed lines and alternate longer and shorter bonds therein are indicated in red and blue, respectively. | |
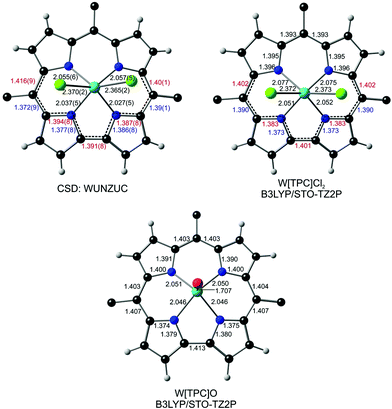 |
| Fig. 2 Selected distances in the X-ray structure of a WCl2-triarylcorrole and the DFT (B3LYP/STO-TZ2P) optimized geometry of Mo[TPC]Cl2. Parts of the corrole skeleton exhibiting bond length alternation are indicated with dashed lines and alternate longer and shorter bonds therein are indicated in red and blue, respectively. | |
To complement the above crystallographic findings, we carried out scalar-relativistic DFT calculations using the Zeroth Order Regular Approximation (ZORA) to the Dirac equation (as implemented in the ADF program system) and all-electron STO-TZ2P basis sets on four model systems – Mo[TPC]Cl2, Mo[TPC]O, W[TPC]Cl2 and W[TPC]O (where TPC = triphenylcorrolato). To derive trustworthy conclusions, we used six exchange–correlation functionals including the extensively tested, high-quality pure functionals OLYP and OPBE, the hybrid functionals B3LYP, TPSSH, and OPBE0, and the range-separated hybrid functional CAM-B3LYP. All beautifully confirmed the crystallographic results and the expected bond length alternations for Mo[TPC]Cl2 and the lack of such alternations for Mo[TPC]O (Fig. 1). The calculations also confirmed bond length alternations for W[TPC]Cl2 (Fig. 2), but careful examination of the optimized geometries showed that the alternations are smaller in magnitude than for Mo[TPC]Cl2, potentially indicating a lower degree of corrole˙2− character in the W case.
Spin density profiles and MO analysis
All six functionals yielded qualitatively similar spin density profiles (Table 1). For Mo[TPC]Cl2, the Mo Mulliken spin population was found to be 1.10–1.35 (Fig. 3 and Table 1), while a corrole spin population of ∼−0.3 was found to be distributed approximately in the form of a porphyrin-like “a2u” radical. The spin density of W[TPC]Cl2 (Fig. 4) is qualitatively similar, but displays smaller spatial separation of majority and minority spin (−0.2 on the corrole) densities. In contrast, we found minimal evidence for a similar metal(dz2)-corrole(“a2u”) interaction and hence of a corrole radical for Mo[TPC]O and W[TPC]O.
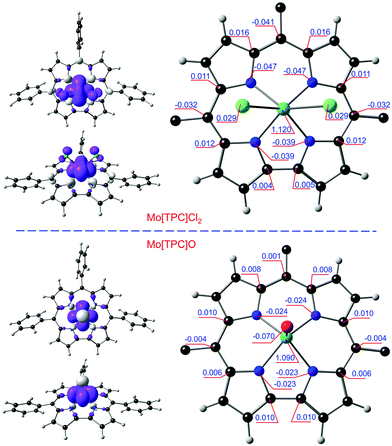 |
| Fig. 3 Top and side views of DFT (B3LYP/STO-TZ2P) spin density plots (contour = 0.002 e Å−3) for Mo[TPC]Cl2 and Mo[TPC]O. Majority and minority spin densities are indicated in purple and ivory, respectively. Mulliken spin populations are shown for selected atoms. | |
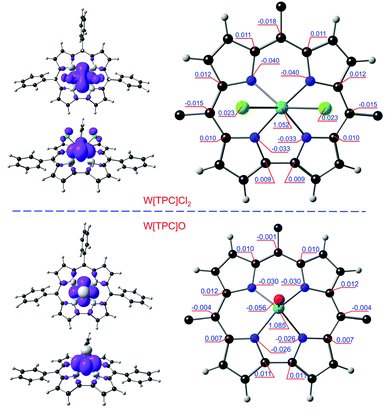 |
| Fig. 4 Top and side views of DFT (B3LYP/STO-TZ2P) spin density plots (contour = 0.002 e Å−3) for W[TPC]Cl2 and W[TPC]O. Majority and minority spin densities are indicated in purple and ivory, respectively. Mulliken spin populations are shown for selected atoms. | |
Table 1 Mulliken spin populations for selected atoms in Mo[TPC]Cl2 for different exchange–correlation functionals. (Note that only half the molecule is effectively symmetry-distinct.)
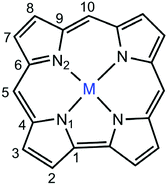
|
|
OLYP |
OPBE |
B3LYP |
TPSSH |
OPBE0 |
CAM-B3LYP |
Mo |
1.101 |
1.170 |
1.120 |
1.136 |
1.342 |
1.226 |
Cl1 |
0.032 |
0.022 |
0.029 |
0.026 |
0.009 |
0.025 |
C1 |
0.005 |
0.004 |
0.005 |
0.005 |
0.002 |
0.001 |
C2 |
−0.001 |
−0.002 |
0.001 |
0.000 |
0.004 |
0.005 |
C3 |
0.004 |
0.004 |
0.002 |
0.003 |
0.000 |
−0.002 |
C4 |
0.008 |
0.009 |
0.012 |
0.013 |
0.021 |
0.019 |
C5 |
−0.022 |
−0.026 |
−0.032 |
−0.033 |
−0.062 |
−0.056 |
C6 |
0.008 |
0.009 |
0.011 |
0.011 |
0.016 |
0.013 |
C7 |
0.002 |
0.002 |
0.005 |
0.004 |
0.011 |
0.018 |
C8 |
−0.001 |
−0.001 |
−0.002 |
−0.002 |
−0.006 |
−0.010 |
C9 |
0.008 |
0.008 |
0.016 |
0.015 |
0.031 |
0.040 |
C10 |
−0.025 |
−0.031 |
−0.041 |
−0.042 |
−0.086 |
−0.092 |
N1 |
−0.038 |
−0.045 |
−0.039 |
−0.041 |
−0.069 |
−0.052 |
N2 |
−0.044 |
−0.053 |
−0.047 |
−0.050 |
−0.086 |
−0.069 |
It is instructive to interpret the spin density profile of Mo[TPC]Cl2 in terms of Mo(4d)-corrole(π) interactions as reflected in the frontier Kohn–Sham molecular orbitals (MOs), several of which are depicted in Fig. 5. The topology of the spin density on the Mo is consistent with an unpaired electrons in the dxy orbital (HOMO−4, 173α) and fractional occupancy of the Mo 4dyz (HOMO−3, 174α) and 4dz2 orbitals (HOMO−2, 173β), the z direction being normal to the mean plane of the corrole. Note that the HOMO−2 and HOMO−3 are the majority- and minority-spin correspondents of what is essentially the “porphyrin a2u-like” corrole-HOMO. The latter, minority-spin MO is slightly more localized on the corrole than on the MoCl2 unit, relative to the former, accounting for the net a2u-type radical character evident in the molecule's spin density profile (Fig. 3). The Mo(4dz2)-corrole(“a2u”) interaction evident in the HOMO−2 and LUMO (Fig. 5) is, topologically, exceedingly similar to analogous interactions involving the 3dz2 orbital in MnCl,23 FeCl,10–15 FeNO,27 and Co-PPh331 corroles. Importantly, this picture was fully borne out by an examination of the 4d-based natural bond orbitals (NBOs) of the molecule.
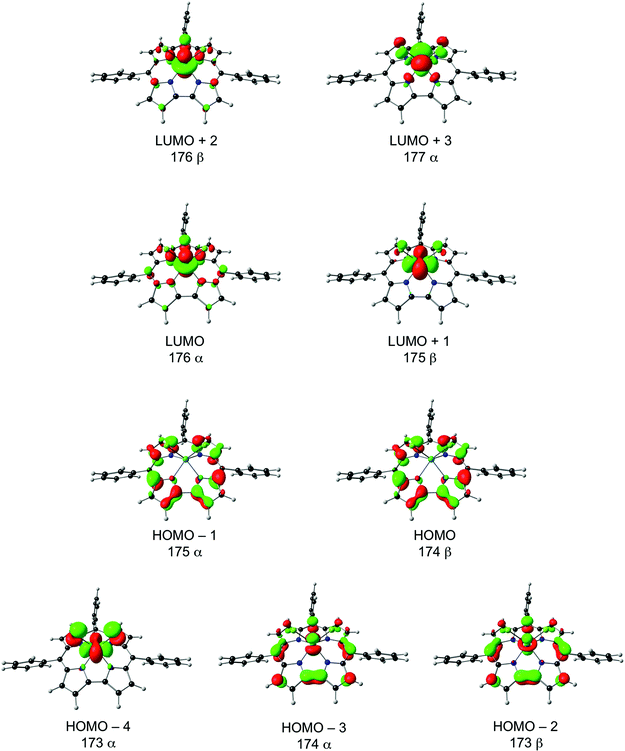 |
| Fig. 5 Selected frontier Kohn–Sham MOs of Mo[TPC]Cl2. | |
The electronic-structural difference between MoCl2 and MoO corroles may be likened to similar differences within MnCl/MnPh and FeCl/FePh corroles. In each of these cases, a stronger-field ligand (whether strongly σ-donating like Ph or both strongly σ- and π-donating like oxo) stabilizes a higher-valent metal center and concomitantly an innocent corrole. A weaker axial ligands such as chloride behaves oppositely, resulting in a noninnocent corrole.
To gain an estimate of the strength of the Mo(4dz2)-corrole˙2− antiferromagnetic coupling, we optimized the corresponding ferromagnetically-coupled (S = 3/2) state of Mo[TPC]O and found its energy to be ∼0.9 eV above the ground state (for the B3LYP functional). In stark contrast, the ferromagnetically coupled states of MnCl,23 FeCl,10–15 FeNO,27 and Co-PPh331 corroles were invariably found to occur only a few tenths of an eV higher in energy relative to the antiferromagnetically-coupled ground state, a testament to much stronger metal–ligand orbital interactions for the heavier transition elements. This result provides a rare measure of metal–ligand magnetic coupling for a 4d transition metal.
In situ electronic absorption spectroscopy
The optical spectra of a series of meso-tris(p-X-phenyl)corrole (TpXPC) derivatives provide one of the simplest yet most reliable indications of the innocence or otherwise of the corrole macrocycle.22 If the Soret maximum is essentially invariant with respect to the electron-donating/withdrawing power of the para substituent X, the metallocorrole series in question may be expected to be innocent. On the other hand, if the Soret band redshifts markedly in response to increasing electron-donating character of X, then the corrole is noninnocent. To apply this optical probe to MoCl2 corroles, we generated a series of Mo[TpXPC]Cl2 (X = CF3, H, CH3, and OCH3) derivatives from the corresponding MoO complexes and recorded their optical spectra in situ (on account of their high reactivity). Of these, Mo[TpOCH3PC]Cl2 is a known compound and the similarity of the spectral profiles and extinction coefficients strongly indicated an analogous formulation for the other compounds. Gratifyingly, the spectra indeed exhibited dramatic substituent-induced shifts of the Soret maximum (Fig. 6). As shown in Table 1, the Soret maxima of the MoCl2 complexes redshift by 37 nm between X = CF3 and X = OCH3, while the Q maxima remain essentially unchanged. The Soret maxima of the MoO series, in contrast, are known to be invariant with respect to the substituent X.50
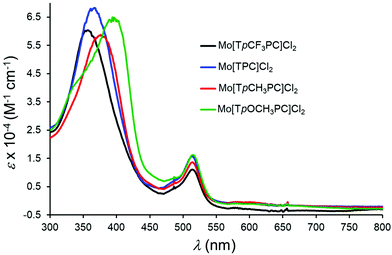 |
| Fig. 6 UV-vis spectra of Mo[TpXPC]Cl2, where X = CF3, H, CH3 and OCH3, in dry dichloromethane. | |
Conclusion
Three lines of evidence – crystallographic and DFT optimized geometries, DFT spin density profiles, and in situ electronic absoprtion spectroscopy – indicate that Viking-helmet MoCl2 triarylcorroles are noninnocent with significant MoIV-corrole˙2− character. The crystallographic structure in question, Mo[TpOCH3PC]Cl2 (CSD: NEMMAW), exhibits characteristic bond length alternations within and around the bipyrrole part of the molecule. Six different functionals reproduce this geometrical attribute with high fidelity. The six functionals also yield mutually consistent broken-symmetry spin density profiles with characteristic spatial separation of α and β spin densities. Finally, the UV-vis optical probe, which has been successfully used to identify ligand noninnocence in >15 families of metallocorroles (as well as to rule out ligand noninnocence in another >20 families), strongly suggests that MoCl2 triarylcorroles are noninnocent, while the analogous MoO complexes are not. (A family here refers to a series of metallocorroles in which the substituents vary systematically, but the metal and axial ligands, if any, are constant.) In this study, we have not obtained a reliable estimate of the percent radical character of MoCl2 corroles. UV-vis spectral shifts, however, suggest a fairly substantial fraction, on the order of 40% or so, based on similar shifts observed for 3d-based noninnocent corroles,22 which have been analyzed with CASSCF/DMRG-CASPT2 calculations.27 The overall evidence for a noninnocent macrocycle in MoCl2 Viking-helmet corroles, in our opinion, thus, is incontrovertible.
Computational methods
All DFT calculations were carried out with the ADF 2018 program system.51 Relativistic effects were taken into account with the zeroth-order regular approximation (ZORA52–54) to the Dirac equation, applied as a scalar correction. Large all-electron ZORA STO-TZ2P basis sets were used throughout. Six exchange–correlation functionals were used: OLYP,55,56 OPBE,55,57,58 B3LYP (with 15% Hartree–Fock exchange),56,59,60 TPSSH,61 OPBE0 (with 25% Hartree–Fock exchange),58 and CAM-B3LYP.62 Fine integration meshes and tight convergence criteria were used throughout.
Experimental methods
In situ UV-Vis measurements
To a 50-mL round-bottom flask equipped with a stirring bar was added a carefully weighed sample of an MoO triarylcorrole (∼2.5–3.0 mg or 0.004 mmol) dissolved in dry dichloromethane (2.0 mL). The solution was degassed with a flow of argon for five minutes, following which silicon tetrachloride (0.01 mL, 0.087 mmol), dissolved in dry dichloromethane (0.5 mL), was added. After stirring for 10 min, the red color of the starting material changed to the light brown color of Mo[TpXPC]Cl2, and UV-vis analysis of an aliquot of the reaction mixture proved that the reaction was complete. The reaction mixture was diluted to a known volume for quantitative UV-vis analysis. In view the high reactivity of the products, we assumed complete conversion of the starting materials and the extinction coefficients were calculated based on the mass of the starting materials (Fig. 6 and Table 2).
Table 2
In situ UV-vis absorption data for Mo[TpXPC]Cl2
Compound |
λ
max [nm, ε x 10−4 (M−1 cm−1)] |
Soret |
Q
|
Mo[TpCF3PC]Cl2 |
357 (6.03) |
514 (1.11) |
Mo[TPC]Cl2 |
366 (6.08) |
513 (1.57) |
Mo[TpCH3PC]Cl2 |
376 (5.87) |
514 (1.36) |
Mo[TpOCH3PC]Cl2 |
394 (6.48) |
514 (1.62) |
Notes
There is no archeological evidence that Vikings used horned helmets similar to those depicted in popular culture.63 On the other hand, horned helmets from the Bronze Age (depicted in Scheme 1) have been discovered at Veksø, Denmark.64
Conflicts of interest
There are no conflicts to declare.
Acknowledgements
This work was supported by the Research Council of Norway (grant no. 262229 to AG), the South African National Research Foundation (grant no. 113327 and 96111 to JC), and the Central Research Fund of the University of the Free State (JC). The High Performance Computing facility of the UFS and the CHPC of South Africa is acknowledged for computer time (JC).
References
- C. K. Jørgensen, Coord. Chem. Rev., 1966, 1, 164–178 CrossRef.
- W. Kaim and B. Schwederski, Coord. Chem. Rev., 2010, 254, 1580–1588 CrossRef CAS.
- R. Eisenberg and H. B. Gray, Inorg. Chem., 2011, 50, 9741–9751 CrossRef CAS PubMed.
- J. Rittle and M. T. Green, Science, 2010, 330, 933–937 CrossRef CAS PubMed.
- C. Jung, S. de Vries and V. Schünemann, Arch. Biochem. Biophys., 2011, 507, 44–55 CrossRef CAS PubMed.
- S. K. Singh, J. Eng, M. Atanasov and F. Neese, Coord. Chem. Rev., 2017, 344, 2–25 CrossRef CAS.
- J.-P. Dognon, Coord. Chem. Rev., 2017, 344, 150–162 CrossRef CAS.
- G. A. Bailey, J. A. Buss, P. H. Oyala and T. Agapie, J. Am. Chem. Soc., 2021, 143, 13091–13102 CrossRef PubMed.
- E. Steene, T. Wondimagegn and A. Ghosh, J. Phys. Chem. B, 2001, 105, 11406–11413 CrossRef CAS
Erratum: J. Phys. Chem. B 2002, 106, 5312–5312.
- O. Zakharieva, V. Schünemann, M. Gerdan, S. Licoccia, S. Cai, F. A. Walker and A. X. Trautwein, J. Am. Chem. Soc., 2002, 124, 6636–6648 CrossRef CAS PubMed.
- S. Cai, S. Licoccia, C. D'Ottavi, R. Paolesse, S. Nardis, V. Bulach, B. Zimmer, T. Kh. Shokhireva and F. A. Walker, Inorg. Chim. Acta, 2002, 339C, 171–178 CrossRef.
- E. Steene, A. Dey and A. Ghosh, J. Am. Chem. Soc., 2003, 125, 16300–16309 CrossRef CAS PubMed.
- F. A. Walker, S. Licoccia and R. Paolesse, J. Inorg. Biochem., 2006, 100, 810–837 CrossRef CAS PubMed.
- S. Ganguly, K. E. Thomas, R. Sarangi and A. Ghosh, Chem. – Eur. J., 2017, 23, 15098–15106 CrossRef CAS PubMed.
- K. P. Caulfield, J. Conradie, H. D. Arman, A. Ghosh and Z. J. Tonzetich, Iron(II) Corrole Anions, Inorg. Chem., 2019, 58, 15225–15235 CrossRef CAS PubMed.
- J. Krzystek, A. Schnegg, A. Aliabadi, K. Holldack, S. A. Stoian, A. Ozarowski, S. D. Hicks, M. M. Abu-Omar, K. E. Thomas, A. Ghosh, K. P. Caulfield, Z. J. Tonzetich and J. Telser, Inorg. Chem., 2020, 59, 1075–1090 CrossRef CAS PubMed.
- P. Schweyen, K. Brandhorst, M. Hoffmann, B. Wolfram, M.-K. Zaretzke and M. Bröring, Chem. – Eur. J., 2017, 23, 13897–13900 CrossRef CAS PubMed.
- R. Padilla, H. L. Buckley, A. L. Ward and J. Arnold, J. Porphyrins Phthalocyanines, 2015, 19, 150–153 CrossRef CAS.
- K. E. Thomas, A. B. Alemayehu, J. Conradie, C. M. Beavers and A. Ghosh, Acc. Chem. Res., 2012, 45, 1203–1214 CrossRef CAS PubMed.
- A. Ghosh, Chem. Rev., 2017, 117, 3798–3881 CrossRef CAS PubMed.
- S. Nardis, F. Mandoj, M. Stefanelli and R. Paolesse, Coord. Chem. Rev., 2019, 388, 360–405 CrossRef CAS.
- S. Ganguly and A. Ghosh, Acc. Chem. Res., 2019, 52, 2003–2014 CrossRef CAS PubMed.
- S. Ganguly, L. J. McCormick, J. Conradie, K. J. Gagnon, R. Sarangi and A. Ghosh, Inorg. Chem., 2018, 57, 9656–9669 CrossRef CAS PubMed.
- H. Vazquez-Lima, H. K. Norheim, R. F. Einrem and A. Ghosh, Dalton Trans., 2015, 44, 10146–10151 RSC.
- H. K. Norheim, J. Capar, R. F. Einrem, K. J. Gagnon, C. M. Beavers, H. Vazquez-Lima and A. Ghosh, Dalton Trans., 2016, 45, 681–689 RSC.
- M. H. Rahmann, M. D. Ryan, H. Vazquez-Lima, A. Alemayehu and A. Ghosh, Inorg. Chem., 2020, 59, 3232–3238 CrossRef PubMed.
- K. Pierloot, Q. M. Phung and A. Ghosh, Inorg. Chem., 2020, 59, 11493–11502 CrossRef CAS PubMed.
- S. Ganguly, H. Vazquez-Lima and A. Ghosh, Chem. – Eur. J., 2016, 22, 10336–10340 CrossRef CAS PubMed.
- S. Ganguly, J. Conradie, J. Bendix, K. J. Gagnon, L. J. McCormick and A. Ghosh, J. Phys. Chem. A, 2017, 121(50), 9589–9598 CrossRef CAS PubMed.
- W. R. Osterloh, V. Quesneau, N. Desbois, S. Brandès, W. Shan, V. Blondeau-Patissier, R. Paolesse, C. P. Gros and K. M. Kadish, Inorg. Chem., 2020, 59, 595–611 CrossRef CAS PubMed.
- S. Ganguly, D. Renz, L. J. Giles, K. J. Gagnon, L. J. McCormick, J. Conradie, R. Sarangi and A. Ghosh, Inorg. Chem., 2017, 56, 14788–14800 CrossRef CAS PubMed.
- I. H. Wasbotten, T. Wondimagegn and A. Ghosh, J. Am. Chem. Soc., 2002, 124, 8104–8116 CrossRef CAS PubMed.
- M. Bröring, F. Bregier, E. C. Tejero, C. Hell and M. C. Holthausen, Angew. Chem., Int. Ed., 2007, 46, 445–448 CrossRef PubMed.
- A. B. Alemayehu, L. K. Hansen and A. Ghosh, Inorg. Chem., 2010, 49, 7608–7610 CrossRef CAS PubMed.
- S. Berg, K. E. Thomas, C. M. Beavers and A. Ghosh, Inorg. Chem., 2012, 51, 9911–9916 CrossRef CAS PubMed.
- K. E. Thomas, I. H. Wasbotten and A. Ghosh, Inorg. Chem., 2008, 47, 10469–10478 CrossRef CAS PubMed.
- K. E. Thomas, J. Conradie, L. K. Hansen and A. Ghosh, Eur. J. Inorg. Chem., 2011, 1865–1870 CrossRef CAS.
- I. K. Thomassen, L. J. McCormick and A. Ghosh, ACS Omega, 2018, 3, 5106–5110 CrossRef CAS PubMed.
- K. E. Thomas, N. S. Settineri, S. J. Teat, E. Steene and A. Ghosh, ACS Omega, 2020, 5, 10176–10182 CrossRef CAS PubMed.
- H. Lim, K. E. Thomas, B. Hedman, K. O. Hodgson, A. Ghosh and E. I. Solomon, Inorg. Chem., 2019, 58, 6722–6730 CrossRef CAS PubMed.
- A. B. Alemayehu, K. E. Thomas, R. F. Einrem and A. Ghosh, Acc. Chem. Res., 2021, 54, 3095–3107 CrossRef CAS PubMed.
- A. B. Alemayehu, L. J. McCormick, K. J. Gagnon, S. M. Borisov and A. Ghosh, ACS Omega, 2018, 3, 9360–9368 CrossRef CAS PubMed.
- A. B. Alemayehu, H. Vazquez-Lima, C. M. Beavers, K. J. Gagnon, J. Bendix and A. Ghosh, Chem. Commun., 2014, 50, 11093–11096 RSC.
- P. Schweyen, K. Brandhort, R. Wicht, B. Wolfram and M. Bröring, Angew. Chem., Int. Ed., 2015, 54, 8213–8216 CrossRef CAS PubMed.
- K. E. Thomas, H. Vazquez-Lima, Y. Fang, Y. Song, K. J. Gagnon, C. M. Beavers, K. M. Kadish and A. Ghosh, Chem. – Eur. J., 2015, 21, 16839–16847 CrossRef CAS PubMed.
- R. Sarangi, L. J. Giles, K. E. Thomas and A. Ghosh, Eur. J. Inorg. Chem., 2016, 3225–3227 CrossRef CAS.
- I. Luobeznova, M. Raizman, I. Goldberg and Z. Gross, Inorg. Chem., 2006, 45, 386–394, DOI:10.1021/ic051483g.
- A. E. Meier-Callahan, H. B. Gray and Z. Gross, Inorg. Chem., 2000, 39, 3605–3607 CrossRef CAS PubMed.
- A. Garai, S. Sobottka, R. Schepper, W. Sinha, M. Bauer, B. Sarkar and S. Kar, Chem. – Eur. J., 2018, 24, 12613–12622 CrossRef CAS PubMed.
- I. Johansen, H.-K. Norheim, S. Larsen, A. B. Alemayehu, J. Conradie and A. Ghosh, J. Porphyrins Phthalocyanines, 2011, 15, 1335–1344 CrossRef CAS.
- G. T. Velde, F. M. Bickelhaupt, E. J. Baerends, C. F. Guerra, S. J. A. van Gisbergen, J. G. Snijders and T. Ziegler, J. Comput. Chem., 2001, 22, 931–967 CrossRef.
- E. van Lenthe, E. J. Baerends and J. G. Snijders, J. Chem. Phys., 1993, 99, 4597–4610 CrossRef CAS.
- E. van Lenthe, E. J. Baerends and J. G. Snijders, J. Chem. Phys., 1994, 101, 9783–9792 CrossRef CAS.
- E. van Lenthe, A. Ehlers and E. J. Baerends, J. Chem. Phys., 1999, 110, 8943–8953 CrossRef CAS.
- N. C. Handy and A. J. Cohen, Mol. Phys., 2001, 99, 403–412 CrossRef CAS.
- C. Lee, W. Yang and R. G. Parr, Phys. Rev. B: Condens. Matter Mater. Phys., 1988, 37, 785–789 CrossRef CAS PubMed.
- J. P. Perdew, M. Ernzerhof and K. Burke, J. Chem. Phys., 1996, 105, 9982–9985 CrossRef CAS.
- M. Swart, A. W. Ehlers and K. Lammertsma, Mol. Phys., 2004, 102, 2467–2474 CrossRef CAS.
- A. D. Becke, Phys. Rev. A, 1988, 38, 3098–3100 CrossRef CAS PubMed.
- P. J. Stephens, F. J. Devlin, C. F. Chabalowski and M. J. Frisch, J. Phys. Chem., 1984, 98, 11623–11627 CrossRef.
- J. Tao, J. P. Perdew, V. N. Staroverov and G. E. Scuseria, Phys. Rev. Lett., 2003, 91, 146401 CrossRef PubMed.
- T. Yanai, D. P. Tew and N. C. Handy, Chem. Phys. Lett., 2004, 393, 51–57 CrossRef CAS.
-
H. Hencken, The Earliest European Helmets: Bronze Age and Early Iron Age. Harvard University, Cambridge, MA, 1971, 220 pp Search PubMed.
- H. Norling-Christensen, Acta Archaeol., 1946, 17, 99–115 Search PubMed.
Footnote |
† Electronic supplementary information (ESI) available. See DOI: 10.1039/d1dt01970h |
|
This journal is © The Royal Society of Chemistry 2021 |