DOI:
10.1039/D1DT00711D
(Paper)
Dalton Trans., 2021,
50, 8057-8069
Coordination chemistry of [2 + 2] Schiff-base macrocycles derived from the dianilines [(2-NH2C6H4)2X] (X = CH2CH2, O): structural studies and ROP capability towards cyclic esters†
Received
2nd March 2021
, Accepted 4th May 2021
First published on 4th May 2021
Abstract
Reaction of the [2 + 2] Schiff-base macrocycles {[2-(OH)-5-(R)-C6H2-1,3-(CH)2][CH2CH2(2-C6H4N)2]}2 (R = Me, L1H2; tBu, L2H2) with FeBr2 afforded the complexes [FeBr(L1H2)]2[(FeBr3)2O]·2MeCN (1·2MeCN), [FeBr(L2H2)][X] (X = 0.5(FeBr3)2O, 2·0.5MeCN, X = Br, 3·5.5MeCN), respectively. Reaction of L2H2 with [KFe(OtBu)3(THF)] (formed in situ from FeBr2 and KOtBu), following work-up, led to the isolation of the complex [Fe(L2)(L2H)]·3MeCN (4·3MeCN), whilst with [CuBr2] afforded [CuBr(L2H2)][CuBr2]·2MeCN (5·2MeCN). Attempts to form mixed Co/Ti species by reaction of [CoBrL2][CoBr3(NCMe)] with TiCl4 resulted in [L2H4][CoBr4]·2MeCN (6·2MeCN). Use of the related oxy-bridged Schiff-base macrocycles {[2-(OH)-5-(R)-C6H2-1,3-(CH)2][O(2-C6H4N)2]}2 (R = Me, L3H2; tBu, L4H2) with CoBr2 led to the isolation of the complexes [(CoBr)2(L3)]·2C3H6O (7·2C3H6O), [Co(NCMe)2(L4H2)][CoBr4]·5MeCN (8·5MeCN), [Co(NCMe)6][CoBr3(MeCN)]2·2MeCN (9·2MeCN). For comparative structural/polymerisation studies, the complexes {CoBr(NCMe)L5}2·2MeCN (10·2MeCN) and [Co(NCMe)2L5]2[CoBr3(NCMe)]2 (11), [FeBr(NCMe)L5]2·2MeCN (12·2MeCN) where L5H = 2,6-(CHO)2-4-tBu-C6H2OH, as well as the chelate-free salt [Fe(NCMe)6][FeBr3OFeBr3] (13) have been isolated and structurally characterized. The ability of these complexes to act as catalysts for the ring opening polymerisation (ROP) of ε-caprolactone (ε-CL) and δ-valerolactone (δ-VL) was investigated, as well as co-polymerisation of ε-CL with rac-lactide (r-LA) and vice versa.
Introduction
Global issues over plastic pollution continue to drive the search for alternative, more environmentally-friendly materials.1 As part of our search for new catalysts capable of affording biodegradable polymers via the ring opening polymerisation (ROP) of cyclic esters, we have initiated investigations into the coordination chemistry of Schiff-base macrocycles derived from the [2 + 2] condensation of the dianilines [(X)(2-C6H4NH2)2] (X = CH2CH2, O) with the diformylphenols 2,6-(CHO)2-4-R-C6H2OH (R = Me, tBu) (Chart 1).2 To date, we have reported how remote alkylaluminium centres bound to the macrocycle (X = CH2CH2) exhibited beneficial cooperative effects in the ROP of ε-caprolactone (ε-CL), whereas aluminoxane type (Al–O–Al) bonding proved to be detrimental.3 Manganese complexes bearing these macrocycles were far less active (conversions <15%).4 However, studies of mixed cobalt/zinc systems revealed interesting catalytic properties, with homo-dinuclear systems exhibiting inactivity while mixed-metal systems proved to be efficient for the ROP of ε-CL and δ-valerolactone (δ-VL).5 It is also noteworthy that the structural chemistry of macrocycles of this type remains underexplored; a search of the CSD revealed no hits,6 other than our reported aluminium, manganese and cobalt systems.3–5 Given this, we have re-focused our efforts on such Schiff-base systems and have extended our studies to iron, cobalt and copper complexes bearing [2 + 2] macrocycles derived from the dianilines [(X)(2-C6H4NH2)2] (X = CH2CH2, O, Chart 2). Herein, we report the molecular structures of these complexes, and have screened a number of them for their capability in the ROP of ε-CL, δ-VL and rac-lactide (r-LA), and for the co-polymerisation of ε-CL with r-LA and vice versa. Poly(ε-caprolactone), PCL, and poly(lactide), PLA, are favoured polymers given their biodegradability properties, and their co-polymers are considered as potential environmentally-friendly commodity plastic.7
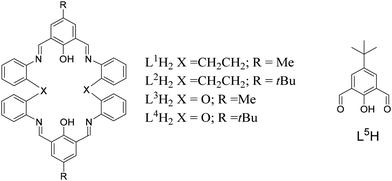 |
| Chart 1 Synthesis of ligands L1H2–L5H prepared herein. | |
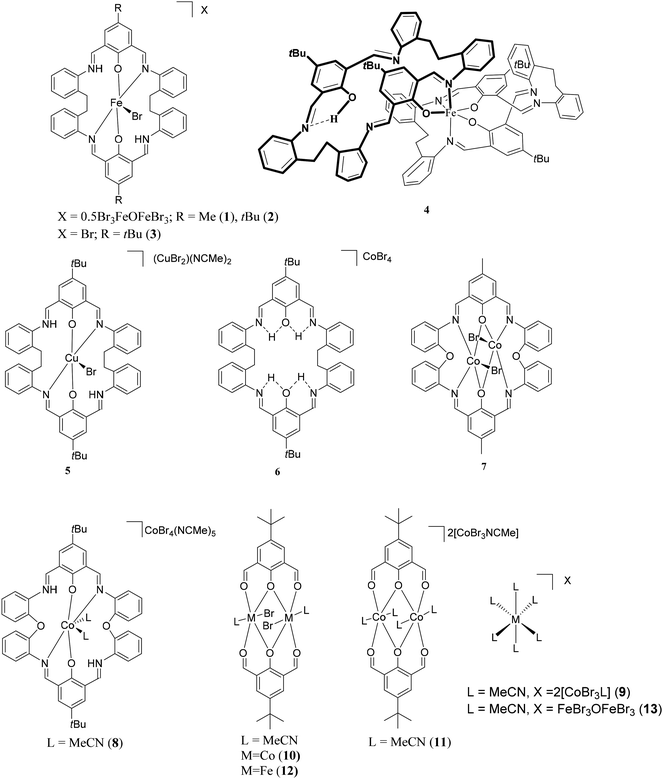 |
| Chart 2 Structures of iron and cobalt complexes 1–13 prepared herein. | |
Results and discussion
–CH2CH2– bridged systems
Iron.
Use R = Me L1H2.
Iron is a cheap, earth abundant metal and its complexes have shown potential in the ROP of cyclic esters.8 Given this, we have initiated studies on the iron chemistry of our [2 + 2] macrocyclic systems. The reaction of the macrocycle {[2-(OH)-5-Me-C6H2-1,3-(CH)2][CH2CH2(2-C6H4N)2]}2 (L1H2) with two equivalents of FeBr2 in refluxing toluene afforded, following work-up (MeCN), a brown crystalline solid in moderate yield. Single crystals were grown from a saturated solution of acetonitrile on standing at ambient temperature for 3 days. The molecular structure is shown in Fig. 1, with selected bond lengths and angles given in the caption. There are two macrocyclic iron complexes in the asymmetric unit, related by a pseudosymmetric translation of c/2 (see ESI†), plus an anion of [Br3FeOFeBr3] and two molecules of acetonitrile. Of the macrocyclic bound iron centres, both Fe1 and Fe2 adopt a distorted trigonal bipyramidal geometry (τ = 0.69),9 bound by a bromide and two nitrogen atoms and two oxygen atoms of the macrocycle, apical sites are occupied by O atoms, with the bromide and N atoms in the equatorial sites. The composition is thus [FeBr(L1)]2[Br3FeOFeBr3]·2(MeCN) (1·2MeCN). In terms of charge, the 2+ available from the anion which contains two Fe(III) centres, is balanced by the two cations (2× + 1), each of which contains an Fe(II) centre.
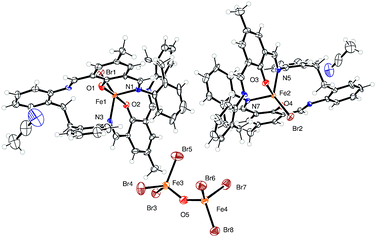 |
| Fig. 1 Asymmetric unit for the structure of [FeBr(L1)]2[Br3FeOFeBr3]·2(MeCN) (1·2MeCN). Thermal ellipsoids are drawn at the 50% probability level. Selected bond lengths (Å) and angles (°): Fe(1)–O(1) 2.002(6), Fe(1)–O(2) 2.020(6), Fe(1)–N(1) 2.129(7), Fe(1)–N(3) 2.138(7), Fe(1)–Br(1) 2.5070(16), Fe(2)–O(3) 2.031(6), Fe(2)–N(5) 2.150(7), Fe(2)–N(7) 2.135(7), Fe(2)–Br(2) 2.5104(15); O(1)–Fe(1)–O(2) 177.0(2), N(1)–Fe(1)–N(3) 103.8(3), Br(1)–Fe(1)–N(1) 120.26(19), N(5)–Fe(2)–N(7) 102.9(3). | |
Use R = tBu L2H2.
Similar reaction of {[2-(OH)-5-(tBu)-C6H2-1,3-(CH)2][CH2CH2(2-C6H4N)2]}2 (L2H2) with 2.1 equivalents of FeBr2 afforded, following work-up, red needles in good yield. Crystals suitable for X-ray diffraction were grown from a saturated solution of acetonitrile at ambient temperature. A view of the molecular structure is shown in Fig. 2, and bond lengths and angles are given in the caption. The crystal comprises FeBr(L2H2) cations, (FeBr3)2O anions and MeCN solvent molecules. The anions lie about a centre of symmetry and the solvent molecule sites refine best with half-occupancy; the molecular formula is therefore [FeBr(L2H2)], 0.5[(FeBr3)2O]·0.5(MeCN) (2·0.5MeCN), and like 1·2MeCN, is a mixed oxidation state Fe(II)/Fe(III) system. The iron atom in the cation is five-coordinate with a trigonal bipyramidal pattern (τ = 0.82);9 the apical sites are occupied by O atoms, with the bromide and N atoms in the equatorial sites. There is a pseudo two-fold symmetry axis along the Fe–Br bond. In the macrocyclic ligand, there are four N atoms, each involved in a double bond, viz. C11
N2 1.298(5) C52
N1 1.287(6) C26
N3 1.296 (6) C37
N4 1.308 (7), N1 and N3 are coordinated to the Fe atom, while N2 and N4 are bonded to two C atoms (one with a double bond) and a hydrogen atom. The hydrogen atoms were included in the structure factor calculations in a planar, trigonal fashion, and their Uiso values were refined freely and satisfactorily; it is believed that both these groups are charged C–N+H
C groups. Both these hydrogen atoms are involved in intramolecular hydrogen bonds.
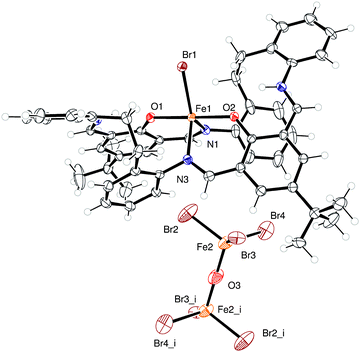 |
| Fig. 2 View of the [FeBr(L2)][(FeBr3)2O]0.5·0.5(MeCN) (2·0.5MeCN) complex ion, indicating the atom numbering scheme. Thermal ellipsoids are drawn at the 50% probability level. Symmetry equivalent atoms are generated by i = 1 − x, 1 − y, 1 − z. Selected bond lengths (Å) and angles (°): Fe(1)–O(1) 2.052(3), Fe(1)–O(2) 2.059(3), Fe(1)–N(1) 2.131(4), Fe(1)–N(3) 2.124(4), Fe(1)–Br(1) 2.4967(8); Br(1)–Fe(1)–O(1) 89.71(9), O(1)–Fe(1)–N(3) 94.83(14). | |
The (FeBr3)2O anion lies about a centre of symmetry. One of the bromide ligands is disordered over two sites, in an 82
:
18 occupancy ratio. The nearest neighbours of the bromide atoms are atoms of the disordered t-butyl group.
Similar treatment of {[2-(OH)-5-(tBu)-C6H2-1,3-(CH)2][O(2-C6H4N)2]}2, but with limited FeBr2 (1.1 equivalents), led to the isolation of the Fe(II) salt [FeBr(L2H2)]Br·3MeCN (5.5MeCN). The molecular structure is provided in the ESI (Fig. S2†).
Use of [KFe(OtBu)3(THF)].
Given alkoxide species play a central role in metal-catalysed ROP, we attempted to generate an iron alkoxide species. Reaction of L2H2 with in situ generated [KFe(OtBu)3(THF)] in refluxing toluene resulted, after work-up, in the isolation of a brown crystalline material. Crystals grown from a saturated solution of acetonitrile were found to be a bis-chelate structure [Fe(L2)(L2H)]·3MeCN (4·3MeCN) (see Fig. 3), in which a distorted octahedral iron(III) centre is bound to two of the macrocyclic ligands. The asymmetric unit contains one iron complex and 3 molecules of acetonitrile. The coordination at the iron is such that one macrocycle is bound only in chelate fashion via N,O-type ligation, whilst the second macrocycle utilizes four atoms to bind in 2× N,O-type fashion. We have observed similar binding modes recently for aluminium.3c
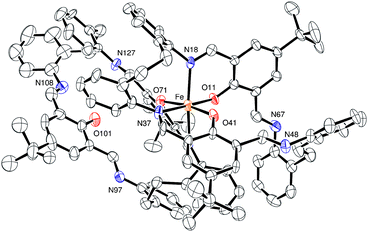 |
| Fig. 3 View of [Fe(L2)(L2H)]·3MeCN (4·3MeCN), indicating the atom numbering scheme. Thermal ellipsoids are drawn at the 50% probability level. For clarity hydrogen atoms are not shown. Selected bond lengths (Å) and angles (°): Fe(1)–O(11) 1.936(4), Fe–O(41) 1.931(4), Fe–O(71) 1.905(4), Fe–N(18) 2.234(5), Fe–N(37) 2.189(5), Fe–N(78) 2.240(5); O(11)–Fe–O(41) 92.1(2), O(11)–Fe–N(37) 176.6(2), N(18)–Fe–N(78) 164.0(2). | |
Use of copper bromide.
Copper is also an earth abundant metal, though it has had only limited success in the ROP of cyclic esters.10 For the successful systems, Schiff-base ligation appears beneficial, suggesting that interaction with the macrocyclic systems of the type herein could lead to a ROP active complex. Reaction of L2H2 with two equivalents of CuBr2 afforded, following work-up, the orange/brown Cu salt complex [CuBr(L2H2)][CuBr2]·2MeCN (5·2MeCN) in good yield. In the cation, the copper centre is distorted trigonal bipyramidal with oxygens at the apex and two nitrogens and a bromide in the equatorial plane (τ = 0.90).9 The anion Cu(I)Br2− resides close to a pocket formed by the macrocycle, see Fig. 4.
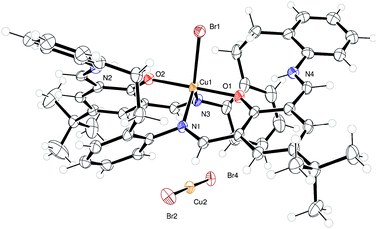 |
| Fig. 4 Molecular structure of [CuBr(L2H2)][CuBr2]·2MeCN (5·2MeCN) with atoms drawn as 50% probability ellipsoids. Solvent molecules are not shown. Selected bond lengths (Å) and angles (°): Cu(1)–O(1) 1.955(3), Cu(1)–O(2) 1.948(3), Cu(1)–N(1) 2.106(3), Cu(1)–N(3) 2.074(3), Cu(1)–Br(1) 2.4790(6); O(1)–Cu(1)–O(2) 179.8(1), N(1)–Cu(1)–Br(1) 119.58(9), N(3)–Cu(1)–Br(1) 125.8(1). | |
Attempted mixed-metal systems
Having successfully isolated mixed cobalt/zinc systems, which were active for ring opening polymerization whereas the analogous homodinuclear species were not,5 we attempted to prepare mixed cobalt/titanium complexes. Our entry point was again the cobalt complex [CoBrL2H2][CoBr3(NCMe)], and reaction with [TiCl4] resulted in [L2H4][CoBr4]·2MeCN (6·2MeCN) as the only crystalline product. The molecular structure of the salt 6 comprises a protonated macrocycle L2H2 and the Co(II) containing anion [CoBr4]2−; see ESI (Fig. S4†) for details.
–O– bridged systems
We have also initiated a programme to investigate the coordination chemistry of the oxy-bridged macrocycles {[2-(OH)-5-(R)-C6H2-1,3-(CH)2][O(2-C6H4N)2]}2 (R = Me L3H2, tBu L4H2). Reaction of L3H2 with two equivalents of CoBr2 afforded, following work-up (acetone), the complex [(CoBr)2L3]·2C3H6O (7·2C3H6O), containing two Co(II) centres, as black crystals in ca. 40% isolated yield. Single crystals can be grown from a saturated solution of acetone at ambient temperature, and the molecular structure is shown in Fig. 5; selected bond lengths and angles are given in the caption. Both cobalt centres adopt distorted trigonal bipyramidal with a bromide at each apex (τ = 0.59 and 0.53 for Co1 and Co2, respectively).9
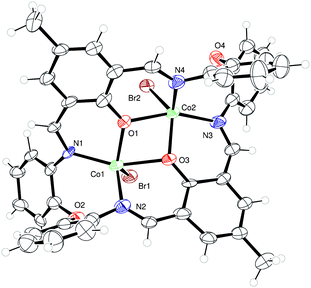 |
| Fig. 5 Molecular structure of [(CoBr)2(L3)]·2C3H6O (7·2C3H6O) with atoms drawn as 50% probability ellipsoids. Selected bond lengths (Å) and angles (°): Co(1)–O(1) 1.982(11), Co(1)–O(3) 2.216(11), Co(1)–N(1) 2.067(12), Co(1)–N(2) 2.091(13), Co(1)–Br(1) 2.421(3), Co(2)–O(1) 2.179(11), Co(2)–O(3) 1.977(11), Co(2)–N(3) 2.102(14), Co(2)–N(4) 2.079(13), Co(2)–Br(2) 2.421(3); O(1)–Co(1)–O(3) 70.0(4), N(1)–Co(1)–N(2) 112.3(5), O(1)–Co(1)–Br(1) 122.2(3), O(1)–Co(2)–N(3) 157.8(5), N(4)–Co(2)–Br(2) 111.2(4). | |
In the case of L4H2, reaction with CoBr2 afforded, following work-up (MeCN), two sets of crystals (∼90
:
10). Both sets were subjected to single crystal X-ray diffraction, and the major product, as shown in Fig. 6, was found to be the Co(II) containing salt [Co(NCMe)2(L4H2)][CoBr4]·5MeCN (8·5MeCN). In the cation, the cobalt centre is distorted octahedral, and is bound by two phenoxide oxygens and the two nitrogens N(1) and N(3) of the macrocycle plus two bound acetonitrile ligands. In the solid-state, there are C–H⋯Br interactions present. The minor product was found to be Co(II) containing salt [Co(NCMe)6][CoBr3NCMe]2·2MeCN (9·2MeCN), details of this structure can be found in the ESI (Fig. S4†).
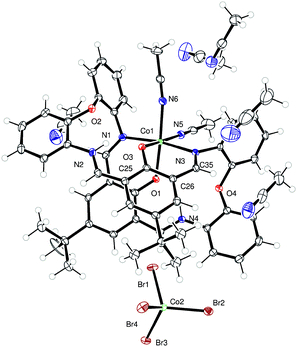 |
| Fig. 6 Molecular structure of [Co(NCMe)2(L4)][CoBr4]·5MeCN (8·5MeCN) with atoms drawn as 50% probability ellipsoids. Selected bond lengths (Å) and angles (°): Co(1)–O(1) 2.016(3), Co(1)–O(3) 2.056(3), Co(1)–N(1) 2.156(3), Co(1)–N(3) 2.156(3), Co(1)–N(5) 2.121(3), Co(1)–N(6) 2.114(3); O(1)–Co(1)–O(3) 91.98(11), N(1)–Co(1)–N(3) 169.15(12), O(1)–Co(1)–N(6) 174.71(13), O(3)–Co(1)–N(5) 174.77(13)°. | |
‘Dialdehyde’ systems
To probe the role played by the presence of the macrocycle during catalysis (see ROP section), we have also prepared cobalt complexes bearing chelate ligands derived from the 4-tert-butyl-2,6-diformylphenol 2,6-(CHO)2-4-tBu-C6H2OH (L5H). Reaction of L5H with CoBr2 in the presence of excess Et3N afforded, after work-up (MeCN), the Co(II) complex {[CoBr(NCMe)]L5}2·2MeCN (10·2MeCN) as orange/brown prisms in good yield. The molecular structure is shown in Fig. 7, with selected bond lengths and angles given in the caption. Each cobalt centre is distorted octahedral, and are bound by oxygens from two L ligands and a bromide and acetonitrile ligand. The ligand arrangement is such that one bromide and an MeCN ligand reside above the Co2O6 plane and one bromide and an MeCN ligand are below this plane.
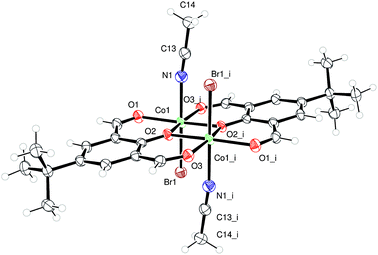 |
| Fig. 7 Molecular structure of {[CoBr(NCMe)]L5}2·2MeCN (10·2MeCN) with atoms drawn as 50% probability ellipsoids (unbound solvent not shown). Selected bond lengths (Å) and angles (°): Co(1)–O(1) 2.052(2), Co(1)–O(2) 2.079(2), Co(1)–Br(1) 2.5751(6), Co(1)–N(1) 2.163(3); O(1)–Co(1)–O(2) 87.17(8), Co(1)–O(2)–Co(1_i) 100.59(9), Br(1)–Co(1)–N(1) 169.51(8). | |
If the same reaction is conducted in the absence of Et3N, then, following work-up (MeCN), green prisms are isolated in good yield. The molecular structure (see Fig. 8 for the cation) revealed the structure of the complex to be Co(II) containing salt [Co(NCMe)2L5]2[CoBr3(NCMe)]2 (11). The dimer is centrosymmetric – the second half of the dimer is generated by the symmetry operation −x, 1 − y, 1 − z. Each cobalt centre in the cation is distorted octahedral, but unlike 10, there is no bound bromide.
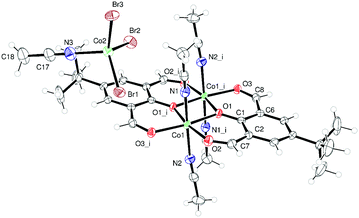 |
| Fig. 8 Molecular structure of [Co(NCMe)2L5]2[CoBr3(NCMe)]2 (11) with atoms drawn as 50% probability ellipsoids. Symmetry operation used to generate equivalent atoms: i = −x, 1 − y, 1 − z. Selected bond lengths (Å) and angles (°): Co(1)–O(1) 2.046(2), Co(1)–O(2) 2.050(2), Co(1)–N(1) 2.108(3), Co(1)–N(2) 2.143(3); O(1)–Co(1)–O(2) 88.56(9), Co(1)–O(2)–Co(1i) 99.59(9), N(1)–Co(1)–N(2) 172.07(11). | |
If a similar reaction is conducted with FeBr2 in the presence of Et3N, then, following work-up (MeCN), black prisms are isolated in good yield. The molecular structure (Fig. S5†) revealed the structure of the Fe(II) complex to be [FeBr(NCMe)L5]2·2MeCN (12·2MeCN). Each iron centre in the cation is distorted octahedral, bound by oxygens from two L ligands, a bromide and an acetonitrile ligand.
Finally, for comparative catalytic studies, the salt [Fe(NCMe)6] [FeBr3OFeBr3] (13) was prepared from L1H2 and FeBr3.
Ring opening polymerisation (ROP) of ε-CL and δ-VL
The Fe, Cu and Co complexes 1, 2, 4, 5, 9–13 have been screened for their ability to ring open polymerise ε-caprolactone (ε-CL) and δ-valerolactone (δ-VL); runs were conducted in the presence of benzyl alcohol (BnOH). For complexes 1, 2 and 4, a variety of conditions were used in the attempted ROP of ε-CL including differing ratios of [ε-CL]
:
[Cat]
:
[BnOH] and run times, and it was found that the Fe compounds were far more active than the other metal complexes screened herein. ROP systems based on Fe,8 Cu10 and Co5,11 have been reported with mixed success.
The polymerisation data for the ε-CL runs is given in Table 1. Highest conversion was achieved using 2 in run 5 using the ratio 500
:
1
:
1 at 130 °C over 24 h, albeit with less control than observed in run 6 using 250
:
1
:
1. Systems employing 1 and 4 were also less controlled, whilst the non-macrocyclic cobalt systems 9–11 proved to be inactive under the conditions employed herein. Interestingly, the non-macrocyclic iron complexes 12 and 13 proved to be poorly active with low conversions for ε-CL. However, for δ-VL, a 32% conversion (affording small molecular weight oligomers) was observed for 13, whereas 12 was inactive. The MALDI-TOF spectra can be interpreted using the formula C6H6–CH2O[O(CH2)5CO]nOH, for example see run 5 (Fig. S7, ESI†). The 1H NMR spectrum of the PCL indicated the presence of benzyloxy and hydroxyl end groups (Fig. S8, ESI†). There was evidence of significant transesterication, whilst all observed Mn values were significantly lower than the calculated values.
Table 1 Synthesis of polycaprolactone using catalysts 1, 2, 4, 9–13
Run |
Cat. |
[Monomer] : [cat] : BnOH |
T (°C) |
t/h |
Conv.a (%) |
M
n × 103 b |
M
nCalcd × 104 c |
PDId |
Conversion was confirmed by 1H NMR spectroscopy.
Determined by GPC analysis calibrated with polystyrene standards and multiplied by correction factor of 0.56.
F.W. ([M]/[BnOH])(conversion) + BnOH.
Polydispersity index (Mw/Mn) were determined by GPC.
|
1 |
1
|
500 : 1 : 1 |
130 |
24 |
63.2 |
3.70 |
3.61 |
1.95 |
2 |
1
|
250 : 1 : 1 |
130 |
24 |
88.7 |
2.95 |
2.54 |
3.28 |
3 |
1
|
500 : 1 : 1 |
130 |
12 |
79.4 |
0.97 |
4.53 |
1.11 |
4 |
1
|
500 : 1 : 1 |
130 |
12 |
66.3 |
0.70 |
3.79 |
1.17 |
5 |
2
|
500 : 1 : 1 |
130 |
24 |
99.3 |
5.92 |
5.66 |
1.56 |
6 |
2
|
250 : 1 : 1 |
130 |
24 |
55.0 |
2.60 |
1.58 |
1.06 |
7 |
4
|
500 : 1 : 1 |
130 |
24 |
68.9 |
8.67 |
3.94 |
1.75 |
8 |
4
|
250 : 1 : 1 |
130 |
24 |
87.6 |
7.52 |
2.50 |
1.58 |
9 |
9
|
500 : 1 : 1 |
130 |
24 |
— |
— |
— |
— |
10 |
10
|
500 : 1 : 1 |
130 |
24 |
— |
— |
— |
— |
11 |
11
|
500 : 1 : 1 |
130 |
24 |
— |
— |
— |
— |
12 |
12
|
500 : 1 : 1 |
130 |
24 |
8.4 |
— |
0.45 |
— |
13 |
13
|
500 : 1 : 1 |
130 |
24 |
15.3 |
— |
0.87 |
— |
In the case of δ-VL (Table 2), complexes 1 and 2 exhibited better conversions versus4 and 12, whilst 5, 9, 10 and 11 proved to be inactive. In the MALDI-TOF mass spectra of the PVL, a number of families of peaks were observed separated by 100 mass units (Fig. S22, ESI†) can be assigned by the formula C6H6–CH2O[O(CH2)4CO]nOH; the highest were typically about 6000 (run 4, Fig. S21†). The 1H NMR spectra of the PVL (e.g. Fig. S20†) revealed the presence of benzyloxy and OH end groups. The observed molecular weights were lower than the calculated values, suggesting the presence of a chain transfer agent (H2O or BnOH).
Table 2 ROP of δ-VL using complexes 1, 2, 4, 5, 9–13
Run |
Cat. |
[VL] : [Cat] : BnOH |
T (°C) |
t/h |
Conv.a (%) |
M
n × 103 b |
M
nCalcd × 104 c |
PDId |
Conversion was confirmed by 1H NMR spectroscopy.
Determined by GPC analysis calibrated with polystyrene standards and multiplied by correction factor of 0.58.
F.W. ([M]/[BnOH])(conversion) + BnOH.
Polydispersity index (Mw/Mn) were determined by GPC.
|
1 |
1
|
500 : 1 : 1 |
130 |
24 |
98.6 |
2.52 |
4.94 |
1.14 |
2 |
1
|
250 : 1 : 1 |
130 |
24 |
94.3 |
3.10 |
2.36 |
1.28 |
3 |
1
|
500 : 1 : 1 |
130 |
12 |
39.7 |
— |
2.00 |
— |
4 |
2
|
500 : 1 : 1 |
130 |
24 |
96.1 |
6.00 |
4.92 |
1.38 |
5 |
2
|
250 : 1 : 1 |
130 |
24 |
93.2 |
4.53 |
2.41 |
1.23 |
6 |
2
|
500 : 1 : 1 |
130 |
12 |
83.8 |
— |
4.20 |
— |
7 |
4
|
500 : 1 : 1 |
130 |
24 |
34.5 |
2.59 |
1.84 |
1.25 |
8 |
4
|
250 : 1 : 1 |
130 |
24 |
23.5 |
2.51 |
0.60 |
1.27 |
9 |
5
|
500 : 1 : 1 |
130 |
24 |
— |
— |
— |
— |
10 |
9
|
500 : 1 : 1 |
130 |
24 |
— |
— |
— |
— |
11 |
10
|
500 : 1 : 1 |
130 |
24 |
— |
— |
— |
— |
12 |
11
|
500 : 1 : 1 |
130 |
24 |
— |
— |
— |
— |
13 |
12
|
500 : 1 : 1 |
130 |
24 |
— |
— |
— |
— |
14 |
13
|
500 : 1 : 1 |
130 |
24 |
32.0 |
0.76 |
2.18 |
1.26 |
From a kinetic study (Fig. S11 and S18†), it was observed that the polymerisation rate exhibited near first order dependence on the ε-CL or δ-VL concentration at 130 °C, and complex 2 displayed the best rate in both δ-VL [Kobs = 7.29 × 10−3 h−1] and ε-CL polymerisations [Kobs = 4.70 × 10−3 h−1].
Synthesis of block co-polymers
The co-polymerisation of ε-CL with r-LA and of δ-VL with r-LA was also examined (Table 3). Noteworthy, the co-polymerisation of ε-CL with r-LA is much easier than δ-VL with r-LA when using the Fe complexes. In the presence of complex 2, the Mn of the block co-poly(ε-CL + r-LA) reached around 12
000. Complexes 1 and 4 were less active in these co-polymerisations. As observed by 1H NMR spectroscopy (Fig. S14 and S24†), the co-polymers were also capped by benzyloxy and hydroxyl end groups. The composition of the copolymer was further illustrated by 13C NMR spectroscopy (Fig. S17 and S25†). From the MALDI-ToF mass spectrum (positive mode) of poly(ε-CL + r-LA) a gap of 114 corresponding to the molecular weight of ε-CL was evident, whilst running the spectra in negative method revealed a gap of 144 corresponding to the molecular weight of r-LA (Fig. S12 and 13†). The MALDI-ToF mass spectrum for co-poly (δ-VL + r-LA) was also recorded, see Fig. S26.† The 2D J-resolved 1H NMR spectrum for the copolymer was recorded and the peaks were assigned to the corresponding tetrads (see Fig. S17 and S22, ESI†) according to literature reports,12 which revealed an atactic LA–LA–LA chain.
Table 3 Synthesis of block copolymers from cyclic ester monomers using the Fe catalysts 1, 2 and 4
Run |
Composition |
Catalyst |
t (h) |
Incorporated amounta |
M
n × 103 b |
PDI |
Conversion was confirmed by 1H NMR spectroscopy.
Determined by GPC analysis calibrated with polystyrene standards and multiplied by correction factor of 0.56.
|
1 |
Poly (ε-CL + r-LA) |
1
|
24 + 24 :![[thin space (1/6-em)]](https://www.rsc.org/images/entities/char_2009.gif) |
ε-CL : r-LA = 47 : 53 |
3.75 |
2.22 |
[ε-CL] : [r-LA] : [cat] : [BnOH] = 500 : 500 : 1 : 1 |
2 |
Poly (δ-VL + r-LA) |
1
|
24 + 24 |
δ-VL : r-LA = 14 : 86 |
5.51 |
1.73 |
[δ-VL] : [r-LA] : [cat] : [BnOH] = 500 : 500 : 1 : 1 |
3 |
Poly (r-LA + ε-CL) |
2
|
24 + 24 |
r-LA : ε-CL = 49 : 51 |
10.55 |
1.51 |
[r-LA] : [ε-CL] : [cat] : [BnOH] = 500 : 500 : 1 : 1 |
4 |
Poly (r-LA + ε-CL) |
2
|
24 + 24 |
r-LA : ε-CL = 93 : 7 |
6.20 |
1.82 |
[ε-CL] : [r-LA] : [cat] : [BnOH] = 250 : 250 : 1 : 1 |
5 |
Poly (ε-CL + r-LA) |
2
|
24 + 24 |
ε-CL : r-LA = 60 : 40 |
9.31 |
1.64 |
[ε-CL] : [r-LA] : [cat] : [BnOH] = 500 : 500 : 1 : 1 |
6 |
Poly (ε-CL + r-LA) |
2
|
24 + 24 |
ε-CL : r-LA = 63 : 37 |
12.63 |
3.02 |
[ε-CL] : [r-LA] : [cat] : [BnOH] = 250 : 250 : 1 : 1 |
7 |
Poly (δ-VL + r-LA) |
2
|
24 + 24 |
δ-VL : r-LA = 55 : 45 |
3.40 |
1.26 |
[δ-VL] : [r-LA] : [cat] : [BnOH] = 500 : 500 : 1 : 1 |
8 |
Poly (δ-VL + r-LA) |
2
|
24 + 24 |
δ-VL : r-LA = 88 : 12 |
2.57 |
2.56 |
[δ-VL] : [r-LA] : [cat] : [BnOH] = 250 : 250 : 1 : 1 |
9 |
Poly (ε-CL + r-LA) |
4
|
24 + 24 |
ε-CL : r-LA = 100 : 0 |
4.61 |
1.23 |
[ε-CL] : [r-LA] : [cat] : [BnOH] = 500 : 500 : 1 : 1 |
10 |
Poly (ε-CL + r-LA) |
4
|
24 + 24 |
ε-CL : r-LA = 90 : 10 |
3.61 |
1.18 |
[ε-CL] : [r-LA] : [cat] : [BnOH] = 250 : 250 : 1 : 1 |
Conclusion
In conclusion, we have successfully synthesised iron, cobalt and copper complexes bearing [2 + 2] Schiff-base macrocycles derived from dianilines containing CH2CH2 bridges. Cobalt complexes were prepared from a related dianiline containing an oxy bridge. A number on non-macrocyclic Fe and Co complexes were also prepared for comparative catalytic studies. The iron complexes outperformed the other metal systems herein for the ring opening polymerisation of both ε-caprolactone and δ-valerolactone. Best results were obtained using the iron salt 2, however the non-macrocyclic system 12 also performed well. Complex 2 was also capable of the copolymerisation of ε-CL (or δ-VL) with rac-lactide, affording copolymers appreciable amounts of each monomer incorporated.
Experimental
General
All manipulations were carried out under an atmosphere of nitrogen using standard Schlenk and cannula techniques or in a conventional nitrogen-filled glove-box. Toluene was refluxed over sodium, whilst acetonitrile was refluxed over calcium hydride. IR spectra (nujol mulls, KBr windows) were recorded on a Nicolet Avatar 360 FT-IR spectrometer; 1H and 13C NMR spectra were recorded at room temperature on a Varian VXR 400 S spectrometer at 400 MHz or a Gemini 300 NMR spectrometer or a Bruker Advance DPX-300 spectrometer. The 1H NMR spectra were calibrated against the residual protio impurity of the deuterated solvent. Elemental analyses were performed by the elemental analysis service at the London Metropolitan University, the Chemistry Department at the University of Hull or Nanjing University. The precursors 2,6-(CHO)2-4-R-C6H2OH (R = Me, tBu) and 2,2′-ethylenedianiline (or 2,2′-oxydianiline) and the Schiff-base pro-ligands were prepared by the literature.3a,c,13–15 For the iron and cobalt complexes, all manipulations were carried out under an atmosphere of dry nitrogen using conventional Schlenk and cannula techniques or in a conventional nitrogen-filled glove box. All solvents were distilled and degassed prior to use (Table 2).
Synthesis of [FeBr(L1H2)]2[(FeBr3)2O]·2(MeCN) (1·2MeCN)
L1H2 (0.52 g, 0.77 mmol) and FeBr2 (0.34 g, 1.58 mmol) were combined in a Schlenk and toluene (20 mL) was added. After refluxing for 12 h, the volatiles were removed in-vacuo, and the residue was extracted into MeCN (20 mL). Prolonged standing at room temperature afforded orange/brown prisms. Yield: 0.72 g, 78%. Elemental analysis calculated for C96H82Br8Fe4N10O5: required: C 49.32% H 3.60% N 5.00% Found: 49.88% H 3.58% N 5.09%. IR (KBr, cm−1): 3378 (s), 2923 (s), 2726 (w), 2671 (w), 2360 (w), 1633 (m), 1585 (m), 1538 (m), 1462 (s), 1377 (s), 1300 (w), 1280 (w), 1238 (m), 1102 (m), 977 (w), 873 (w), 801 (m), 754 (m), 722 (m), 688 (w), 622 (w), 574 (w), 534 (w), 502 (m), 486 (w), 455 (w). M.S. (MALDI-ToF): 817 (M+). Magnetic moment: 6.35 B.M.16
Synthesis of [FeBr(L2H2)]2[(FeBr3)2O]·0.5MeCN (2·0.5MeCN)
To the pro-ligand L2H2 (0.50 g, 0.65 mmol) in toluene was added 2.1 equivalents of FeBr2 (0.30 g, 1.39 mmol), and the system was refluxed for 12 h. On cooling, the volatiles were removed under vacuum, and the residue was extracted into acetonitrile (30 ml) to afford 2·MeCN as red needles (0.48 g, 56%). Elemental analysis calculated for C106H107Br8Fe4N9O5: C 51.97, H 4.40, N 5.15%; found: C 51.21, H 4.43, N 5.55%. IR (KBr, cm−1): 3168 (m), 2925 (s), 2854 (s), 2726 (w), 1704 (m), 1620 (m), 1587 (m), 1542 (s), 1462 (s), 1377 (s), 1317 (w), 1260 (w), 1233 (m), 1214 (m), 1180 (m), 1130 (w), 933 (w), 890 (w), 839 (w), 799 (m), 744 (m), 722 (m), 526 (m), 449 (m). M.S. (MALDI-ToF): 901 (M − anion). Magnetic moment: 5.86 B.M.16
Synthesis of [FeBr(L2H2)]Br·3MeCN (5.5MeCN)
As for 2, but using L2H2 (0.52 g, 0.68 mmol) and FeBr2 (0.16 g, 0.74 mmol) affording 3·5.5MeCN as brown prisms. Yield: 0.52 g, 65%. IR (KBr, cm−1): 3168 (m), 2925 (s), 2854 (s), 2726 (w), 1636 (s), 1619 (s), 1599 (m), 1587 (m), 1537 (s), 1463 (s), 1377 (s), 1333 (m), 1283 (w), 1241 (m), 1183 (m), 1101 (m), 1063 (s), 1007 (m), 976 (m), 880 (s), 794 (m), 753 (s), 722 (m), 689 (m), 622 (w), 595 (w), 575 (w), 535 (w), 513 (m), 494 (m), 476 (w). M.S. (MALDI-ToF): 901 (M − 5.5MeCN − anion).
Synthesis of [Fe(L2)(L2H)]·3MeCN (4·3MeCN)
To FeBr2 (1.00 g, 4.64 mmol) was added KOtBu (1.04 g, 9.27 mmol) in THF (30 ml) at 0 °C and the system was stirred for 5 h. Following removal of the volatiles, L2H2 (3.57 g, 4.64 mmol) and toluene (20 ml) was added and the system was refluxed for 12 h. On cooling, the volatiles were removed and the residue was extracted into cold acetonitrile (30 ml). 4·3MeCN formed. Yield 1.62 g, 41%. Elemental analysis calculated for C104H101FeN8O4 (sample dried in-vacuo for 2 h): C 78.92, H 6.43, N 7.08%. Found C 79.09, H, 6.49, N On prolonged standing at ambient dark brown block. Yield: 1.84 g, 46.6%. IR (KBr, cm−1): 3377 (w), 2958 (s), 2924 (s), 2854 (s), 2726 (w), 1630 (m), 1587 (m), 1461 (s), 1415 (w), 1377 (s), 1260 (s), 1202 (w), 1093 (s), 1019 (s), 863 (m), 800 (s), 755 (w), 740 (w), 723 (m), 705 (w), 662 (w), 566 (w), 530 (w), 504 (w), 465 (w). MS (Maldi): 1584 (M + H)+. Magnetic moment: 5.07 B.M.16
Synthesis of [CuBr(L2H2)][CuBr2]·2MeCN (5·2MeCN)
As for 1, but using L2H2 (0.52 g, 0.68 mmol) and CuBr2 (0.30 g, 1.34 mmol), affording 5 as brown prisms. Yield 0.69 g, 84%. Elemental analysis calculated for C52H52Br3Cu2N4O2: C 55.18, H 4.63, N 4.95%. Found C 54.59, H, 4.48, N 4.93%. IR (KBr, cm−1): 3171 (m), 2922 (s), 2853 (s), 2727 (s), 2671 (w), 2350 (w), 1633 (s), 1618 (s), 1594 (s), 1538 (s), 1463 (s), 1377 (s), 1336 (w), 1285 (m), 1260 (w), 1249 (m), 1239 (w), 1183 (m), 1156 (w), 1103 (m), 1062 (m), 1022 (w), 977 (w), 950 (w), 938 (w), 876 (s), 846 (m), 830 (m), 794 (m), 754 (s), 738 (w), 722 (s), 688 (m), 623 (m), 594 (w), 575 (m), 558 (m), 550 (m). M.S. (ESI): 908 (M − anion), 827 (M − anion − Br). Magnetic moment: 1.19 B.M.17
Synthesis of [L2H4][CoBr4]·2MeCN (6·2MeCN)
To [CoBrL2][CoBr3(NCMe)] (1.00 g, 0.80 mmol) in toluene (30 mL) was added [TiCl4] (0.80 mL, 1.0 M, 0.80 mmol) and the system was refluxed for 12 h. On cooling, the volatiles were removed and the residue was extracted into acetonitrile (30 ml). On prolonged standing at 0 °C small red blocks of 6·2MeCN formed. Yield: 0.38 g, 83%. Elemental analysis calculated for C52H54Br4CoN4O2: C 54.52, H 4.75, N 4.89%. Found C 53.34, H, 5.19, N 4.95%. IR (KBr, cm−1): 3381 (m), 2925 (s), 2924 (s), 2854 (s), 2726 (w), 2359 (w), 1636 (w), 1618 (s), 1594 (m), 1574 (w), 1538 (s), 1462 (s), 1377 (s), 1333 (s), 1285 (m), 1260 (m), 1183 (s), 1102 (m), 1063 (m), 1021 (m), 873 (m), 799 (s), 756 (m), 722 (m), 688 (w), 668 (w), 624 (w), 576 (w), 558 (w), 522 (w). M.S. (ESI): 847 (M − CoBr3). 829 (M − Br4). 766.97 (M − CoBr4). Magnetic moment: 5.05 B.M.18
Synthesis of [(CoBr)2(L3)]·2C3H6O (7·2C3H6O)
To the pro-ligand L3H2 (1.00 g, 1.52 mmol) in toluene was added 2.1 equivalents of CoBr2 (0.70 g, 3.20 mmol), and the system was refluxed for 12 h. On cooling, the volatile were removed under vacuum, and the residue was extracted into acetone (30 ml) to afford 7·2C3H6O as black crystals (0.98 g, 62%). Single crystals can also be grown from a saturated acetone solution of 7. Elemental analysis calculated for C42H30Br2Co2N4O4: C 54.10, H 3.24, N 6.01%. Found C 53.88, H 3.19, N 6.25%. IR (KBr, cm−1): 2924 (s), 2853 (s), 2727 (w), 1746 (w), 1693 (w), 1620 (m), 1587 (m), 1537 (m), 1463 (s), 1377 (s), 1261 (m), 1235 (m), 1215 (m), 1151 (w), 1106 (w), 1072 (m), 1030 (w), 891 (w), 867 (w), 842 (w), 800 (m), 757 (w), 722 (m), 534 (w), 478 (w), 449 (w). M.S. (ESI): 851 (M − 2C3H6O − Br), 714 (M − 2C3H6O − CoBr). Magnetic moment: 6.10 B.M.18
Synthesis of [Co(NCMe)2(L4H2)]·[CoBr4] 5MeCN (8·5MeCN)
To the pro-ligand L4H2 (1.00 g, 1.36 mmol) in toluene was added 2.1 equivalents of CoBr2 (0.62 g, 2.83 mmol), and the system was refluxed for 12 h. On cooling, the volatile were removed under vacuum, and the residue was extracted into acetonitrile (30 ml) to afford 8·3MeCN as red crystals, yield 0.86 g, 44%, and a smaller amount of green crystals of 9, yield ca. 5%. Elemental analysis calculated for C62H65Br4Co2N11O4: C 49.55, H 4.00, N 6.67% Found for 8 C, 49.35, H 3.96, N 6.61%. IR (KBr, cm−1): 3172 (m), 2956 (s), 2923 (s), 2853 (s), 2726 (w), 1619 (m), 1595 (m), 1536 (m), 1461 (s), 1377 (s), 1260 (s), 1155 (w), 1096 (s), 1060 (s), 1022 (s), 892 (w), 874 (w), 800 (s), 722 (s). M.S. (ESI): 933 (M − 7MeCN − 3Br), 817 (M − 7MeCN − 3Br − 2Co). Magnetic moment: 6.94 B.M.18
IR (KBr, cm−1) for Complex 9: 2922 (s), 2854 (s), 2727 (w), 2308 (m), 2282 (m), 2247 (m), 1658 (s), 1635 (s), 1580 (w), 1531 (s), 1464 (s), 1416 (s), 1377 (s), 1367 (s), 1354 (s), 1327 (w), 1287 (m), 1255 (m), 1244 (w), 1222 (m), 1149 (w), 1130 (w), 1098 (w), 1040 (m), 1014 (s), 930 (w), 917 (w), 846 (s), 801 (m), 772 (m), 757 (m), 727 (s), 627 (w), 550 (s), 531 (m), 430 (m).
Synthesis of {CoBr(NCMe)L5}2·2MeCN (10·2MeCN)
To L5H (1.00 g, 4.85 mmol) and [CoBr2] (1.05 g, 4.85 mmol) was added toluene (20 mL) and Et3N (0.3 ml, 0.22 mmol) and the system was refluxed for 12 h. Following removal of volatiles in-vacuo, the residue was extracted into MeCN (20 mL), and on standing at ambient temperature large orange/brown prisms of 10·3MeCN formed. Isolated yield: 1.41 g, 68%. Elemental analysis calculated for C32H38Br2Co2N4O6 (sample dried in-vacuo for 2 h): C 43.66, H 4.19, N 3.64. Found C 43.81, H 4.23, N 3.67%. IR (KBr, cm−1): 2957 (s), 2924 (s), 2360 (s), 2342 (m), 2312 (m), 2284 (s), 1696 (m), 1641 (s), 1566 (m), 1535 (s), 1464 (s), 1402 (w), 1377 (s), 1367 (s), 1352 (w), 1259 (s), 1225 (m), 1093 (s), 1038 (s), 939 (m), 911 (m), 867 (w), 845 (m), 800 (s), 768 (s), 729 (s), 668 (m), 620 (m), 556 (s), 532 (m), 435 (m). M.S. (ESI): 733 (M − MeCN), 585 (M − 3MeCN − Co), 573 (M − MeCN − 2Br). Magnetic moment: 6.35 B.M.18
Synthesis of [Co(NCMe)2L5]2[CoBr3(NCMe)]2 (11)
To L5H (1.00 g, 4.85 mmol) and [CoBr2] (1.05 g, 4.85 mmol) was added toluene (20 mL) and the system was refluxed for 12 h. Following removal of volatiles in-vacuo, the residue was extracted into MeCN (20 mL), and on standing at ambient temperature large green prisms of 11 formed. Isolated yield: 1.53 g, 46%. Elemental analysis calculated for C36H44Co4N6O6 (sample dried in-vacuo for 2 h): C 47.43, H 4.73, N 6.91%. Found C 47.82, H 4.79, N 6.98%. IR (KBr, cm−1): 2926 (s), 2854 (s), 2360 (m), 2342 (w), 2312 (s), 2284 (m), 1641 (s), 1621 (s), 1566 (m), 1535 (s), 1464 (s), 1402 (w), 1377 (s), 1367 (s), 1352 (w), 1259 (s), 1225 (m), 1093 (s), 1038 (s), 1019 (s), 845 (s), 800 (s), 768 (m), 758 (m), 729 (s), 668 (m), 620 (m), 556 (s), 532 (s), 435 (s). M.S. (ESI): 412 (M − 4MeCN − 2Co). Magnetic moment: 6.69 B.M.18
Synthesis of [FeBr(NCMe)L5]2·2MeCN (12·2MeCN)
To L5H (1.00 g, 4.85 mmol) and [FeBr2] (1.06 g, 4.85 mmol) was added toluene (20 mL) and Et3N (0.7 ml, 0.485 mmol) and the system was refluxed for 12 h. Following removal of volatiles in-vacuo, the residue was extracted into MeCN (20 mL), and on standing at ambient temperature large orange/brown prisms of 10·3MeCN formed. Isolated yield: 0.837 g, 40%. Elemental analysis calculated for C28H32Br2Fe2N2O6 (sample dried in-vacuo for 2 h): C 44.02, H 4.22, N 3.67. Found C 44.61, H 4.52, N 3.72%. IR (KBr, cm−1): 3352 (w), 2954 (s), 2923 (s), 2854 (s), 2727 (w), 2360 (w), 2341 (w), 1643 (m), 1620 (m), 1528 (m), 1462 (s), 1377 (s), 1259 (m), 1160 (m), 1037 (s), 1016 (m), 842 (w), 800 (s), 769 (w), 755 (w), 722 (m), 668 (w), 618 (m), 539 (m). M.S. (ESI): 519 (M − 4MeCN − 2Br). Magnetic moment: 6.70 B.M.18
Synthesis of [Fe(NCMe)6][FeOBr3]2 (13)
L1H2 (0.52 g, 0.77 mmol) and [FeBr3] (0.47 g, 1.58 mmol) were combined in a Schlenk and toluene (20 mL) was added. After refluxing for 12 h, the volatiles were removed in-vacuo, and the residue was extracted into MeCN (20 mL). Prolonged standing at room temperature afforded brown prisms; isolated yield 0.39 g 81%. Elemental analysis calculated for C12H18Br6Fe3N6O (sample dried in-vacuo for 2 h): C 15.85, H 2.00, N 9.24%. Found C, 15.61 H, 2.12 N 9.31%. IR (KBr, cm−1): 2925 (s), 2854 (s), 2361 (s), 2339 (s), 1868 (s), 1844 (s), 1830 (m), 1792 (m), 1772 (w), 1750 (m), 1734 (m), 1717 (m), 1700 (m), 1684 (m), 1669 (m), 1653 (m), 1646 (m), 1635 (m), 1616 (s), 1576 (s), 1559 (s), 1540 (s), 1521 (s), 1506 (s), 1497 (m), 1489 (m), 1457 (s), 1419 (m), 1377 (s), 1260 (s), 1089 (s), 800 (s), 668 (s).
ROP procedure
ε-Caprolactone and δ-valerolactone.
Typical polymerisation procedure in the presence of one equivalent of benzyl alcohol (Table 1, run 1) is as follows. A toluene solution of 1 (0.010 mmol, in 1.0 mL toluene) and BnOH (0.010 mmol) were added into a Schlenk tube in the glove-box at room temperature. The solution was stirred for 2 min, and then ε-caprolactone (2.5 mmol) or δ-valerolactone along with 1.5 mL toluene was added to the solution. The reaction mixture was then placed into an oil bath pre-heated to the required temperature, and the solution was stirred for the prescribed time. The polymerisation mixture was then quenched by addition of an excess of glacial acetic acid (0.2 mL) into the solution, and the resultant solution was then poured into cold methanol (200 mL). The resultant polymer was then collected on filter paper and was dried in vacuo.
Co-polymerisations
A toluene solution of catalyst (0.010 mmol, in 1.0 mL toluene) was added into a Schlenk tube in the glove-box at room temperature. The solution was stirred for 2 min, and then 1 equivalent of BnOH (from 1 mmol BnOH in 100 ml toluene) and monomer were added, after 24 h the other monomer was added to the solution. The reaction mixture was then placed into an oil bath pre-heated to the 130 °C, and the solution was stirred for another 24 h. The polymerisation mixture was then quenched by addition of an excess of glacial acetic acid (0.2 mL) into the solution, and the resultant solution was then poured into cold methanol (200 mL). The resultant polymer was then collected on filter paper and was dried in vacuo.
Kinetic studies
The polymerisations were carried out at 130 °C in toluene (1 mL) using 0.010 mmol of complex. The molar ratio of monomer to initiator was fixed at 500
:
1, and at appropriate time intervals, 0.5 μL aliquots were removed (under N2) and were quenched with wet CDCl3. The percent conversion of monomer to polymer was determined by 1H NMR spectroscopy.
Mass spectrometry
Polymer samples were run at the University of Hull using MALDI-TOF MS analysis. Samples were dissolved in THF, and the matrix, 2-(4-hydroxyphenylazo) benzoic acid (HPABA) with added NaOAc was employed, which was dissolved in THF to give a saturated solution. 50 μL of the sample solution was then mixed with 50 μL matrix solution, and 1 μL of the mixed solution applied to the sample target. The sample was allowed to dry in air before analysis. The co-poly (δ-VL + r-LA) (run 7) sample was analysed by MALDI in positive-linear and reflectron modes, with DCTB matrix and NaOAc additive. The sample was fully soluble in THF at 10 mg mL−1.
X-ray crystallography
Single-crystal X-ray diffraction data for the structures (except for 4 and 11) were collected at the UK National Crystallography service on a range of Rigaku Oxford Diffraction ultra-high intensity instruments employing modern areas detectors. Samples were held at 100 K for data collection. For 4, data were from a Rigaku Oxford Diffraction Xcalibur-3 CCD diffractometer at 140 K. For 11, data were from a Stoe IPSD2 image plate diffractometer utilising monochromated Mo radiation (λ = 0.71073 Å). In all cases standard procedures were employed for integration and processing of data. Crystallographic data for all samples are collated in Table 4.
Table 4 Crystallographic data
Compound |
1·2MeCN |
2·0.5MeCN |
3·5.5MeCN |
4·3MeCN |
Formula |
C96H86Br8Fe4N10O5 |
C52H52BrFeN4O2, C2H3N, 0.5(Br6Fe2O) |
C52H52BrFeN4O2, Br, 5.5(C2H3N) |
C104H101FeN8O4, (3MeCN) |
Formula weight |
2322.42 |
1224.841185.89 |
1185.89 |
1705.9 |
Crystal system |
Monoclinic |
Triclinic |
Monoclinic |
Monoclinic |
Space group |
P21/n |
P![[1 with combining macron]](https://www.rsc.org/images/entities/char_0031_0304.gif) |
P21/n |
P21/c |
Unit cell dimensions |
a (Å) |
15.6606(4) |
13.6454(5) |
14.2077(6) |
12.6268(5) |
b (Å) |
16.0326(4) |
13.9070(7) |
27.6241(9) |
30.3249(10) |
c (Å) |
37.4062(11) |
15.6432(8) |
15.1579(8) |
24.6577(10) |
α (°) |
90 |
67.263(5) |
90 |
90 |
β (°) |
99.896(3) |
88.642(4) |
112.484(6) |
100.817(3) |
γ (°) |
90 |
81.638(4) |
90 |
90 |
V (Å3) |
9252.2(4) |
2707.0(2) |
5496.9(5) |
9273.8(6) |
Z
|
4 |
1 |
4 |
4 |
Temperature (K) |
100(2) |
100(2) |
100(2) |
140(1) |
Wavelength (Å) |
1.54184 |
0.71075 |
0.71073 |
0.71073 |
Calculated density (g cm−3) |
1.667 |
1.503 |
1.185 |
1.222 |
Absorption coefficient (mm−1) |
9.439 |
3.531 |
1.766 |
0.223 |
T
min, Tmax |
0.755, 1.000 |
0.592, 1.000 |
0.555, 1.000 |
0.749, 1.185 |
Crystal size (mm3) |
0.08 × 0.05 × 0.02 |
0.30 × 0.015 × 0.01 |
0.26 × 0.045 × 0.01 |
0.74 × 0.18 × 0.16 |
θ(max) (°) |
68.2 |
27.5 |
27.5 |
20.0 |
Reflections measured |
68 070 |
12 337 |
47 911 |
51 064 |
Unique reflections |
16 805 |
12 337 |
12 466 |
8583 |
R
int
|
0.107 |
0.067 |
0.056 |
0.143 |
Number of parameters |
1127 |
604 |
556 |
1129 |
R
1 [F2 > 2σ(F2)] |
0.070 |
0.061 |
0.088 |
0.057 |
wR2 (all data) |
0.178 |
0.164 |
0.168 |
0.123 |
GOOF, S |
1.011 |
1.049 |
1.050 |
0.913 |
Largest difference peak and hole (e Å−3) |
1.42 and −0.86 |
1.53 and −0.80 |
0.90 and −1.61 |
0.30 and −0.25 |
Compound |
5·2MeCN |
6·2MeCN |
7·2C3H6O |
8·3MeCN |
Formula |
C56H58Br3Cu2N6O2 |
C52H54Br4CoN4O2·2(C2H3N) |
C42H30Br2Co2N4O4·2(C3H6O) |
C62H65Br4Co2N11O4 |
Formula weight |
1213.89 |
1145.56 |
1048.56 |
1465.8 |
Crystal system |
Triclinic |
Triclinic |
Monoclinic |
Monoclinic |
Space group |
P![[1 with combining macron]](https://www.rsc.org/images/entities/char_0031_0304.gif) |
P![[1 with combining macron]](https://www.rsc.org/images/entities/char_0031_0304.gif) |
P21/c |
Ia
|
Unit cell dimensions |
a (Å) |
10.0808(2) |
12.2629(5) |
11.4774(8) |
15.77810(10) |
b (Å) |
15.0015(4) |
13.2489(6) |
14.5787(10) |
25.0018(2) |
c (Å) |
18.6604(5) |
17.0384(7) |
26.021(3) |
16.28380(10) |
α (°) |
71.442(2) |
96.850(3) |
90 |
90 |
β (°) |
82.738(2) |
91.115(3) |
100.235(8) |
91.911(10) |
γ (°) |
76.600(2) |
99.672(4) |
90 |
90 |
V (Å3) |
2598.2(1) |
2707.2(2) |
4284.6(6) |
6420.08(8) |
Z
|
2 |
2 |
4 |
4 |
Temperature (K) |
100(2) |
100(2) |
100(2) |
100(2) |
Wavelength (Å) |
1.54184 |
0.71075 |
1.54178 |
0.71073 |
Calculated density (g cm−3) |
1.552 |
1.405 |
1.535 |
1.516 |
Absorption coefficient (mm−1) |
4.572 |
3.309 |
8.652 |
3.060 |
T
min, Tmax |
0.149, 1.000 |
0.652, 1.000 |
0.404, 1.000 |
0.698, 1.000 |
Crystal size (mm3) |
0.70 × 0.60 × 0.15 |
0.10 × 0.04 × 0.02 |
0.14 × 0.03 × 0.01 |
0.25 × 0.18 × 0.05 |
θ(max) (°) |
68.3 |
21.7 |
68.2 |
28.7 |
Reflections measured |
44 092 |
10 619 |
6241 |
147 825 |
Unique reflections |
9337 |
10 619 |
3471 |
16 480 |
R
int
|
0.083 |
0.179 |
0.054 |
0.047 |
Number of parameters |
622 |
564 |
526 |
762 |
R
1 [F2 > 2σ(F2)] |
0.066 |
0.114 |
0.128 |
0.032 |
wR2 (all data) |
0.184 |
0.297 |
0.371 |
0.064 |
GOOF, S |
1.062 |
1.098 |
1.226 |
1.023 |
Largest difference peak and hole (e Å−3) |
3.45 and −1.24 |
1.02 and −1.54 |
2.52 and −2.02 |
0.52 and −0.46 |
Compound |
9
|
10·2MeCN |
11·2MeCN |
12·2MeCN |
13
|
Formula |
C20H30Br6Co3N10 |
C28H32Br2Co2N2O6·2(C2H3N) |
C36H44Br6Co4N6O6 |
C32H38Br2Fe2N4O6 |
C12H18Br6Fe3N6O |
Formula weight |
1066.79 |
852.34 |
1371.95 |
846.18 |
909.33 |
Crystal system |
Monoclinic |
Triclinic |
Triclinic |
Triclinic |
Trigonal |
Space group |
P21/n |
P![[1 with combining macron]](https://www.rsc.org/images/entities/char_0031_0304.gif) |
P![[1 with combining macron]](https://www.rsc.org/images/entities/char_0031_0304.gif) |
P![[1 with combining macron]](https://www.rsc.org/images/entities/char_0031_0304.gif) |
R :H |
Unit cell dimensions |
a (Å) |
8.34720(10) |
8.4718(5) |
10.416(2) |
8.51990(10) |
12.39520(10) |
b (Å) |
18.8457(3) |
9.2109(3) |
11.156(4) |
9.20980(10) |
12.39520(10) |
c (Å) |
12.5811(2) |
12.2495(5) |
11.738(4) |
12.1881(2) |
30.5277(3) |
α (°) |
90 |
101.186(3) |
78.04(3) |
101.2110(10) |
90 |
β (°) |
108.941(2) |
102.715(4) |
70.59(2) |
102.4660(10) |
90 |
γ (°) |
90 |
97.208(4) |
87.78(2) |
97.4090(10) |
120 |
V (Å3) |
1871.95(5) |
900.47(7) |
1257.8(7) |
901.29(2) |
4061.92(8) |
Z
|
2 |
1 |
1 |
1 |
6 |
Temperature (K) |
100(2) |
100(2) |
150(2) |
100(2) |
100(2) |
Wavelength (Å) |
0.71075 |
1.54184 |
0.71073 |
1.54184 |
1.54184 |
Calculated density (g cm−3) |
1.893 |
1.572 |
1.811 |
1.559 |
2.230 |
Absorption coefficient (mm−1) |
7.743 |
10.197 |
6.105 |
9.433 |
23.225 |
T
min, Tmax |
0.154, 0.733 |
0.423, 1.000 |
0.725, 0.820 |
0.646, 1.000 |
0.154, 0.733 |
Crystal size (mm3) |
0.216 × 0.125 × 0.10 |
0.32 × 0.16 × 0.10 |
0.31 × 0.13 × 0.09 |
0.30 × 0.20 × 0.10 |
0.16 × 0.14 × 0.03 |
θ(max) (°) |
27.49 |
68.2 |
29.2 |
70.3 |
67.8 |
Reflections measured |
48 302 |
15 481 |
11 602 |
32 046 |
24 700 |
Unique reflections |
4301 |
3240 |
6632 |
3387 |
1644 |
R
int
|
0.0235 |
0.066 |
0.044 |
0.0342 |
0.0363 |
Number of parameters |
183 |
217 |
268 |
217 |
88 |
R
1 [F2 > 2σ(F2)] |
0.017 |
0.042 |
0.035 |
0.0299 |
0.0188 |
wR2 (all data) |
0.0037 |
0.119 |
0.072 |
0.0822 |
0.0474 |
GOOF, S |
1.170 |
1.072 |
0.823 |
1.091 |
1.111 |
Largest difference peak and hole (e Å−3) |
0.79 and −0.35 |
0.85 and −0.83 |
0.84 and −0.63 |
0.413 and −0.69 |
0.38 and −0.51 |
Crystal structures were solved using direct methods (in SHELXS19a) or dual space methods implemented within SHELXT.19b Completion of structures was achieved by performing least squares refinement against all unique F2 values using SHELXL-2018.20 All non-H atoms were refined with anisotropic displacement parameters. Hydrogen atoms were placed using a riding model. Where the location of hydrogen atoms was obvious from difference Fourier maps, C–H and O–H bond lengths were refined subject to chemically sensible restraints. Minor disorder was treated using standard methods.
The crystal of 5 examined was the best of those available. The crystal was split and intensity data from the major component were used for refinement. The final refinement has good quality of fit (R1 = 6.66%) but it is not perfect because of the imperfect nature of the crystals available.
Conflicts of interest
There are no conflicts of interest to declare.
Acknowledgements
We thank the Chinese Scholarship Council for a PhD studentship (to KW). CR thanks the EPSRC (grant EP/S025537/1) for financial support. We thank the EPSRC National Crystallographic Service at Southampton for data collection and the Physical Sciences Data Service Centre for access to the CCDC. We also thank the National Mass Spectrometry Facility (NMSF), Swansea for data.
References
- L. M. Heidbreder, I. Bablok, S. Drews and C. Menzel, Sci. Total Environ., 2019, 668, 1077–1093 CrossRef CAS PubMed.
-
(a) C. Redshaw, Catalysts, 2017, 7, 165 CrossRef;
(b) O. Santoro, X. Zhang and C. Redshaw, Catalysts, 2020, 10, 800 CrossRef CAS.
-
(a) A. Arbaoui, C. Redshaw and D. L. Hughes, Chem. Commun., 2008, 4717–4719 RSC;
(b) A. Arbaoui, C. Redshaw and D. L. Hughes, Supramol. Chem., 2009, 21, 35–43 CrossRef CAS;
(c) W. Yang, K.-Q. Zhao, T. J. Prior, D. L. Hughes, A. Arbaoui, T. Bian, M. R. J. Elsegood and C. Redshaw, Dalton Trans., 2016, 45, 11990–12005 RSC.
- W. Yang, K.-Q. Zhao, B.-Q. Wang, C. Redshaw, M. R. J. Elsegood, J.-L. Zhao and T. Yamato, Dalton Trans., 2016, 45, 226–236 RSC.
- K. Wang, T. J. Prior and C. Redshaw, Chem. Commun., 2019, 55, 11279–11282 RSC.
- F. H. Allen, Acta Crystallogr., Sect. B: Struct. Sci., 2002, 58, 380–388 CrossRef.
-
(a) S. Brooker, Coord. Chem. Rev., 2001, 222, 33–56 CrossRef CAS;
(b) W. Radecka-Paryzek, V. Patroniak and J. Lisowski, Coord. Chem. Rev., 2005, 249, 2156–2175 CrossRef CAS.
- For examples of iron-based ROP systems see:
(a) B. J. O'Keefe, S. M. Monnier, M. A. Hillmyer and W. B. Tolman, J. Am. Chem. Soc., 2001, 123, 339–340 CrossRef;
(b) B. J. O'Keefe, L. E. Breyfogle, M. A. Hillmyer and W. B. Tolman, J. Am. Chem. Soc., 2002, 124, 4384–4393 CrossRef;
(c) K. R. Delle Chiaie, A. B. Biernesser, M. A. Ortuño, B. Dereli, D. A. Iovan, M. J. T. Wilding, B. Li, C. J. Cramer and J. A. Byers, Dalton Trans., 2017, 46, 12971–12980 RSC;
(d) S.-L. Lee, F.-L. Hu, X.-J. Shang, Y.-X. Shi, A. L. Tan, J. Mizera, J. K. Clegg, W.-H. Zhang, D. J. Young and J.-P. Lang, New J. Chem., 2017, 41, 14457–14465 RSC;
(e) E. Fazekas, G. S. Nichol, J. A. Garden and M. P. Shaver, ACS Omega, 2018, 3, 16945–16953 CrossRef CAS;
(f) M. Cozzolino, V. Leo, C. Tedesco, M. Mazzeo and M. Lamberti, Dalton Trans., 2018, 47, 13229–13238 RSC;
(g) M. A. Ortuño, B. Dereli, K. R. D. Chiaie, A. B. Biernesser, M. Qi, J. A. Byers and C. J. Cramer, Inorg. Chem., 2018, 57, 2064–2071 CrossRef.
- A. W. Addison, T. N. Rao, J. Reedijk, G. C. van Rijn and J. Verschoor, J. Chem. Soc., Dalton Trans., 1984, 7, 1349–1356 RSC.
- For examples of copper-based ROP systems see:
(a) A. Routaray, N. Nath, T. Maharana and A. K. Sutar, J. Macromol. Sci., Part A: Pure Appl. Chem., 2015, 52, 444–453 CrossRef CAS;
(b) M. Mandal, K. Oppelt, M. List, I. Teasdale, D. Chakraborty and U. Monkowius, Chem. Mon., 2016, 147, 1883–1892 CrossRef CAS;
(c) M. Zikode, S. O. Ojwach and M. P. Akerman, Appl. Organomet. Chem., 2017, 31, e3556 CrossRef;
(d) J. Rueda-Espinosa, J. F. Torres, C. V. Gauthier, L. Wojtas, G. Verma, M. A. Macias and J. Hurtado, ChemistrySelect, 2017, 2, 9815–9821 CrossRef CAS.
- For examples of cobalt-based ROP systems see:
(a) H. J. Jeon, Y. C. You and J. H. Youk, J. Polym. Sci., Part A: Polym. Chem., 2009, 47(12), 3078–3085 CrossRef CAS;
(b) J. Zhang, B. Wang, L. Wang, J. Sun, Y. Zhang, Z. Cao and Z. Wu, Appl. Organomet. Chem., 2018, 32, e4077 Search PubMed;
(c) P. Marin, M. J.-L. Tschan, P. Haquette, T. Roisnel, I. del Rosal, L. Maron and C. M. Thomas, Eur. Polym. J., 2019, 120, 109208 CrossRef CAS;
(d) H. C. Pradhan, S. Mantri, A. Routaray, T. Maharaba and A. K. Sutar, J. Chem. Sci., 2020, 132, 25–31 CrossRef CAS;
(e) N. Shen, F. Tian, J. Chang, K. L. Huang, Z. H. Zhang, X. Feng, J. Gu, S. C. Chen, M. Y. He and Q. Chen, CrystEngComm, 2020, 22, 3656–3663 RSC.
-
(a) Z. Zhong, P. J. Dijkstra and J. Feijen, J. Am. Chem. Soc., 2003, 125, 11291–11298 CrossRef CAS;
(b) R. M. Slattery, A. E. Stahl, K. R. Brereton, A. L. Rheingold, D. B. Green and J. M. Fritsch, J. Polym. Sci., Part A: Polym. Chem., 2019, 57, 48–59 CrossRef CAS.
- R. S. Drago, M. J. Desmond, B. B. Corden and K. A. Miller, J. Am. Chem. Soc., 1983, 105, 2287–2296 CrossRef CAS.
- J. J. Randell, C. E. Lewis and P. M. Slagan, J. Org. Chem., 1962, 27, 4098–4101 CrossRef.
- V. C. Gibson, C. Redshaw, W. Clegg, M. R. J. Elsegood, U. Siemeling and T. Türk, Polyhedron, 2004, 23, 189–194 CrossRef CAS.
- B. Weber and E. Kaps, Heteroat. Chem., 2005, 16, 391–397 CrossRef CAS.
- M. Kato, H. B. Jonassen and J. C. Fanning, Chem. Rev., 1964, 64, 99–128 CrossRef CAS.
-
(a) R. W. Handel, H. Willms, G. B. Jameson, K. J. Berry, B. Moubaraki, K. S. Murray and S. Brooker, Eur. J. Inorg. Chem., 2010, 2010(21), 3317–3327 CrossRef;
(b) A. A. Abou-Hussein and W. Linert, Spectrochim. Acta, Part A, 2015, 141, 223–232 CrossRef CAS.
-
(a) G. M. Sheldrick, Acta Crystallogr., Sect. A: Found. Adv., 2008, 64, 112–122 CrossRef CAS;
(b) G. M. Sheldrick, Acta Crystallogr., Sect. A: Found. Adv., 2015, 71, 3–8 CrossRef.
- G. M. Sheldrick, Acta Crystallogr., Sect. C: Struct. Chem., 2015, 71, 3–8 Search PubMed.
|
This journal is © The Royal Society of Chemistry 2021 |