DOI:
10.1039/D1CE00703C
(Paper)
CrystEngComm, 2021,
23, 5241-5248
Trends in rare earth thiophosphate syntheses: Rb3Ln(PS4)2 (Ln = La, Ce, Pr), Rb3−xNaxLn(PS4)2 (Ln = Ce, Pr; x = 0.50, 0.55), and RbEuPS4 obtained by molten flux crystal growth†
Received
27th May 2021
, Accepted 5th July 2021
First published on 5th July 2021
Abstract
Single crystals of new rubidium rare earth thiophosphates with the formulas Rb3Ln(PS4)2 (Ln = La, Ce, Pr), Rb3−xNaxLn(PS4)2 (Ln = Ce, Pr; x = 0.50, 0.55), and RbEuPS4 were crystallized out of a molten RbCl flux. The compounds Rb3Ln(PS4)2 (Ln = La, Ce, Pr) and Rb3−xNaxLn(PS4)2 (Ln = Ce, Pr; x = 0.50, 0.55) both crystallize in the monoclinic crystal system adopting the space groups P21 and P21/c, respectively, while RbEuPS4 crystallizes in the orthorhombic crystal system adopting the space group Pnma. A survey of all known rubidium rare earth thiophosphates grown using an alkali halide flux revealed trends suggesting that the Rb3Ln(PS4)2 and Rb3−xNaxLn(PS4)2 families of compounds can be actively targeted over a wide range of temperatures by employing RbCl as at least a component of the flux. Fluorescence measurements were performed on all compounds obtained, revealing resolved f–f transitions in Rb3Pr(PS4)2 and Rb2.45(2)Na0.55(2)Pr(PS4)2 and only single broad emission peaks for Rb3Ce(PS4)2 and Rb2.50(6)Na0.50(6)Ce(PS4)2, behavior characteristic for cerium(III) compounds.
Introduction
Molten flux crystal growth is a popular synthetic method that has become a pillar of modern solid state chemistry due to its continued success in the production of X-ray diffraction quality single crystals of a wide variety of structural families, including metal oxides,1–3 halides,4,5 and chalcogenides.6–11 The use of alkali halide fluxes in particular has resulted in many new single crystalline compounds, while also having the added benefit of the desired crystalline products being easily removed from the product mixture using common solvents, such as water or methanol.
Molten flux synthesis has been shown to be an effective approach for the synthesis of thiophosphate compounds and, over the past decades, has resulted in the successful crystal growth of various thiophosphate compositions incorporating many elements, including transition metals,12–14 rare earth metals,15–18 and the actinides.11,19 The thiophosphates have garnered attention in recent years for their high ionic conductivities20,21 and for their non-linear optical properties.22,23 In addition, numerous studies have focused simply on investigating the rich structural chemistry of thiophosphates, which were found to exhibit great diversity and modularity in composition and structure due to the many thiophosphate motifs that exists and that have been incorporated into new crystal structures, such as the thiopyrophosphate building block P(V)2S74−,24,25 the ortho-thiophosphate building block P(V)S43−,26–32 the hexathiometadiphosphate building block P(V)2S64−,18,33 and the hexathiohypophosphate building block P(IV)2S62−.18,33–37
Alkali halide fluxes have been demonstrated to be very effective for crystallizing new thiophosphate compounds, such as those prepared in alkali iodide melts by Klepov et al.,34 who reported on numerous rare earth containing thiophosphates, such as the two-dimensional NaLnP2S6 (Ln = La, Ce, Pr) thiohypophosphates and CsLnP2S7 (Ln = Pr, Nd, Sm, Gd, Tb, Dy, Ho, Er, Yb, Y) thiopyrophosphates,34 as well as the family of one-dimensional thiophosphates, Cs2NaLn(PS4)2 (Ln = La–Nd, Sm, Gd–Ho).38 Alkali bromide and chloride fluxes also function well for the synthesis of novel thiophosphates, such as the ALnP2S6 series37 grown out of RbBr and CsBr fluxes, and K4Nd2(PS4)2(P2S6) grown out of a KCl flux.35 Due to the relative sparseness of rubidium containing rare earth thiophosphates, by comparison with those incorporating the other alkali metals, syntheses to create the rubidium analogues of the Cs2NaLn(PS4)2 series created by Klepov et al. were carried out by Kutahyali Aslani et al., by employing the same reagents and ratios but replacing the cesium iodide used by Klepov et al. with rubidium bromide. The change in flux resulted, however, in a new family of thiophosphates with formulas Rb4Ln2(P2S6)(PS4)2 (Ln = La, Ce, Pr, Nd, Sm, Gd).17
An overarching goal of solid state chemistry is to achieve the ability to predict structures and to ultimately engineer structures with desired properties. Given the great diversity of thiophosphate structures created in different flux environments, we decided to pursue the synthesis of new thiophosphates by exploring the role of the flux on the formation of thiophosphate products in the hope to better understand the role of the flux as well as to perhaps be able to predict product formation.6 To probe the effects of the flux on the resulting product formed, experiments were carried out using the same reagents and molar ratios used by Kutahyali Aslani et al., however, this time employing a RbCl flux in place of the previously used RbBr. Herein we report on the synthesis, crystal structures, and select optical measurements of the new rubidium rare earth thiophosphates Rb3Ln(PS4)2 (Ln = La, Ce, Pr), Rb3−xNaxLn(PS4)2 (Ln = Ce, Pr; x = 0.50, 0.55), and RbEuPS4 obtained by employing the RbCl flux. In addition, we discuss our current thoughts concerning the role of the flux in the creation of rubidium rare earth thiophosphates.
Experimental
Reagents
P2S5 (99%, Sigma-Aldrich), Na2S (Alfa Aesar), N,N-dimethylformamide (DMF, Sigma-Aldrich, ACS grade), methanol (MeOH, VWR, ACS grade), and La2S3 (STREM Chemicals Inc., 99.9%) were all used as received. P2S5 and Na2S were stored and handled in a nitrogen glove bag. Ce2S3, Pr2S3, and EuS were obtained according to the procedure described in the literature.39
Synthesis
The compounds Rb3Ln(PS4)2 (Ln = La, Ce, Pr) and Rb3−xNaxLn(PS4)2 (Ln = Ce, Pr; x = 0.50, 0.55) were all obtained using the same synthetic conditions. In a nitrogen filled glove bag, powders of Ln2S3 (Ln = La, Ce, Pr) (0.265 mmol), Na2S (1.063 mmol), and P2S5 (1.062 mmol) were loaded into a fused silica tube in a 1
:
4
:
4 molar ratio along with 1 gram of RbCl flux. The fused silica tube was flame sealed under vacuum and placed in a box furnace set to ramp up to 820 °C in 1 hour where it dwelled for 20 hours and was subsequently slow cooled to 620 °C in 30 hours. The furnace was then shut off to allow the reaction mixture to cool to room temperature. The compound RbEuPS4 was synthesized using an EuS, Na2S, P2S5 molar ratio of 1
:
2
:
2 which was loaded into a fused silica tube that was evacuated, flame sealed, and placed in a box furnace set to the same temperature profile detailed above. To remove the flux and to isolate the single crystals, all products were sonicated in DMF and then subsequently sonicated in MeOH. The crystalline products were filtered and dried in air. The products are moisture sensitive and were therefore stored in a desiccator. Translucent plate crystals of the Rb3Ln(PS4)2 and Rb3−xNaxLn(PS4)2 compounds were obtained via the same product mixtures for each lanthanide sulfide reagent used. RbEuPS4 was obtained as large yellow/brown plates and was a phase pure product.
Single crystal X-ray diffraction
X-ray intensity data from small irregular crystals were collected at 301(2) K for the compounds Rb3Ln(PS4)2 (Ln = Pr, Ce), Rb2.50(6)Na0.50(6)Ce(PS4)2, and RbEuPS4, at 291(2) K for Rb3La(PS4)2, and at 100(2) K for Rb2.45(2)Na0.55(2)Pr(PS4)2 using a Bruker D8 QUEST diffractometer equipped with a PHOTON-II area detector and an Incoatec microfocus source (Mo Kα radiation, λ = 0.71073 Å). The raw area detector data frames were reduced and corrected for absorption effects using the SAINT+ and SADABS programs.40,41 Final unit cell parameters were determined by least-squares refinement of large sets of reflections taken from each data set. An initial structural model was obtained with SHELXT.42 Subsequent difference Fourier calculations and full-matrix least-squares refinement against F2 were performed with SHELXL-2018 (ref. 43) using the Olex2 interface.44 Detailed explanations on the X-ray structure determination of all compounds reported herein can be found in the Supporting Information. The crystallographic data and results of the diffraction experiments are summarized in Table 1.
Table 1 The crystallographic information for all compounds reported herein
Chemical formula |
Rb3La(PS4)2 |
Rb3Pr(PS4)2 |
Rb3Ce(PS4)2 |
Rb2.45(2)Na0.55(2)Pr(PS4)2 |
Rb2.50(6)Na0.50(6)Ce(PS4)2 |
RbEuPS4 |
Formula weight |
713.74 |
715.74 |
714.95 |
681.32 |
683.76 |
396.64 |
Crystal system |
Monoclinic |
Orthorhombic |
Space group, Z |
P21 |
P21 |
P21 |
P21/c |
P21/c |
Pnma
|
a, Å |
9.9053(9) |
9.8265(3) |
9.8512(6) |
9.0570(3) |
9.1218(4) |
17.3308(4) |
b, Å |
6.7376(6) |
6.7613(2) |
6.7530(4) |
17.3059(6) |
17.4862(8) |
6.6787(2) |
c, Å |
11.4553(10) |
11.4228(4) |
11.4321(7) |
9.4252(3) |
9.4704(4) |
6.43470(10) |
β, deg. |
90.005(3) |
90.2997(12) |
90.241(2) |
91.8396(13) |
91.7907(17) |
90 |
V, Å3 |
764.50(12) |
758.92(4) |
760.52(8) |
1476.54(8) |
1509.84(11) |
744.80(3) |
ρ
calcd, g cm−3 |
3.101 |
3.132 |
3.122 |
3.065 |
3.008 |
3.537 |
Radiation (λ, Å) |
Mo Kα (0.71073 Å) |
μ, mm−1 |
13.545 |
14.040 |
13.800 |
12.644 |
12.317 |
16.132 |
T, K |
291(2) |
301(2) |
301(2) |
100(2) |
301(2) |
301(2) |
Crystal dim., mm3 |
0.040 × 0.030 × 0.020 |
0.040 × 0.030 × 0.020 |
0.080 × 0.040 × 0.020 |
0.040 × 0.020 × 0.020 |
0.070 × 0.020 × 0.010 |
0.050 × 0.050 × 0.010 |
2θ range, deg. |
2.718–30.028 |
2.073–29.142 |
2.067–32.597 |
2.250–30.001 |
2.234–28.310 |
3.377–29.992 |
Reflections collected |
35 700 |
27 258 |
51 419 |
47 150 |
35 860 |
12 334 |
Data/parameters/restraints |
4460/132/2 |
4082/134/1 |
5434/134/1 |
4311/143/0 |
3763/143/0 |
1170/43/0 |
R
int
|
0.0387 |
0.0412 |
0.0368 |
0.0462 |
0.0720 |
0.0306 |
Goodness of fit |
1.099 |
1.181 |
1.157 |
1.093 |
1.129 |
1.171 |
R
1(I > 2σ(I)) |
0.0493 |
0.0329 |
0.0262 |
0.0297 |
0.0563 |
0.0247 |
wR2 (all data) |
0.0983 |
0.0541 |
0.0515 |
0.0503 |
0.1267 |
0.0647 |
Powder X-ray diffraction
Powder X-ray diffraction (PXRD) data for phase purity confirmation was collected using a polycrystalline sample of RbEuPS4 obtained by grinding single crystals (Fig. S1†). Data was collected on a Bruker D2 PHASER diffractometer using Cu Kα radiation over a 2θ range 10–65° with a step size of 0.02°.
Energy-dispersive spectroscopy (EDS)
EDS was performed on single crystal products using a Tescan Vega-3 SEM equipped with a Thermo EDS attachment. The SEM was operated in low-vacuum mode. Crystals were mounted on an SEM stub with carbon tape and analyzed using a 20 kV accelerating voltage and an 80 s accumulation time. SEM images of the crystals can be found in Fig. S2.† The obtained EDS spectra for each crystal can be found in Fig. S3–S8.† The results of EDS confirm the presence of elements found by single-crystal X-ray diffraction and are summarized in Table S1.†
Optical properties
Photoluminescence data were collected on HORIBA Scientific Standard Microscope Spectroscopy Systems connected with iHR320 Spectrometer and Synchrony detector operating on Labspec 6 software. Spectra were recorded from 400 to 800 nm using 375 nm laser excitation source power 0.5 mW with 10× UV objective.
Results and discussion
Synthesis
To better understand the role of the flux employed in the molten flux crystal growth of rubidium rare earth thiophosphates, the same starting materials and ratios used by Kutahyali Aslani et al. to synthesize the Rb4Ln2(P2S6)(PS4)2 series17 were employed with the only change being the use of a RbCl flux instead of the RbBr flux. This approach resulted in a new series of thiophosphates, specifically, single crystals of the rubidium rare earth thiophosphates Rb3Ln(PS4)2 (Ln = La, Ce, Pr) and Rb3−xNaxLn(PS4)2 (Ln = Ce, Pr; x = 0.50, 0.55), which were found in the same product mixture. It is important to note that the compound Rb2.61(1)Na0.39(1)La(PS4)2 was also obtained in the same product mixture as Rb3La(PS4)2, however, a similar compound has already been reported in the literature.15
As mentioned earlier, one goal of solid state chemistry is to achieve predictive abilities as it pertains to the targeted synthesis of compounds with desired properties. While this goal is far from being accomplished, it is important to document observed patterns and create conversations that may help push this goal further to completion. Our group has had significant success in the growth of alkali rare earth thiophosphates using various alkali halide fluxes and, given the large number of compositions synthesized, this now allows us to look for patterns within this data. Kutahyali Aslani et al. attempted to make the rubidium analogues of the Cs2NaLn(PS4)2 series created by Klepov et al.38 utilizing the same starting materials and molar ratios however changing the flux from the CsI used by Klepov et al. to a RbBr flux. With just the change in flux, a new series of compounds, Rb4Ln2(P2S6)(PS4)2 (Ln = La, Ce, Pr, Nd, Sm, and Gd), was obtained. This success of crystallizing brand new rubidium rare earth thiophosphates with just a change in flux motivated the work reported herein. Using the same starting materials and reagent ratios employed by Kutahyali Aslani et al., however, changing from a RbBr to a RbCl flux, the new compounds Rb3Ln(PS4)2 (Ln = La, Ce, Pr) and Rb3−xNaxLn(PS4)2 (Ln = Ce, Pr; x = 0.50, 0.55) were obtained. Table 2 lists the known rubidium rare earth thiophosphates grown using alkali halide fluxes.
Table 2 A table listing the starting materials used, reagent ratios, flux identities, and reaction temperatures for all known rubidium rare earth thiophosphates grown using alkali halide fluxes
Compound |
Starting material |
Reagent ratios |
Flux identity |
Reaction temperature |
Ref. |
Rb2.65Na0.35La(PS4)2 |
Ln2S3:Na2S:P2S5 |
1 : 2 : 3 |
RbCl–NaCl |
650 for 48 h |
15
|
Rb2.42Na0.58Nd(PS4)2 |
Ln2S3:Na2S:P2S5 |
1 : 2 : 3 |
RbCl–NaCl |
650 for 48 h |
15
|
Rb2.50(6)Na0.50(6)Ce(PS4)2 |
Ln2S3:Na2S:P2S5 |
1 : 4 : 4 |
RbCl |
820 for 20 h |
This work |
Rb2.45(2)Na0.55(2)Pr(PS4)2 |
Ln2S3:Na2S:P2S5 |
1 : 4 : 4 |
RbCl |
820 for 20 h |
This work |
Rb3Gd(PS4)2 |
Ln2S3:Na2S:P2S5 |
1 : 2 : 3 |
RbCl–NaCl |
650 for 48 h |
15
|
Rb3Ln(PS4)2 Ln = La, Ce, Pr |
Ln2S3:Na2S:P2S5 |
1 : 4 : 4 |
RbCl |
820 for 20 h |
This work |
Rb2NaNd(PS4)2 |
Ln2S3:Na2S:P2S5 |
1 : 2 : 3 |
RbI–NaI |
650 for 20 h |
15
|
Rb4Ln2(P2S6)(PS4)2 Ln = La, Ce, Pr, Nd, Sm, Gd |
Ln2S3:Na2S:P2S5 |
1 : 4 : 4 |
RbBr |
800 for 12 h |
17
|
Rb3Pr3(PS4)4 |
Pr:P:S:RbBr |
10 : 12 : 42 : 12 |
RbBr |
950 for 14 days |
16
|
Rb3Er3(PS4)4 |
Er:P:S:RbBr |
4 : 4 : 16 : 3 |
RbBr |
950 for 14 days |
16
|
RbLaP2S6 |
Ln:RbBr:S:P |
4 : 6 : 18 : 6 |
RbBr |
800 for 24 h |
37
|
The data in the table demonstrates that the use of different fluxes led to the crystallization of different thiophosphate compositions. To discuss the role of the flux requires, however, that we address two interconnected variables: the identity of the flux and the reaction temperature used. It is known that different products can crystallize at different temperatures depending on the kinetic and thermodynamic processes at play.6 The change in flux from the RbBr used by Kutahyali Aslani et al. to the RbCl used herein also necessitated a change in the reaction temperature used. It is common when using molten fluxes as crystallization media that the reaction temperature is set to 100 °C above the melting point of the flux to ensure the full liquification of the flux and maximizing the likelihood of dissolving all reagents within the flux to achieve the supersaturation necessary for crystal growth upon cooling.1 The melting point of RbBr is ∼700 °C which is the reason that Kutahyali Aslani et al. synthesized their crystals at a reaction temperature of 800 °C. The crystals reported herein, using the RbCl flux with a melting point of ∼720 °C, dictated that a reaction temperature of 820 °C be used. The 20 °C difference in reaction temperature, while small, nonetheless introduces temperature as a variable. It is likely that in the case of the Rb3Ln(PS4)2 and Rb3−xNaxLn(PS4)2 families prepared in an RbCl flux, temperature plays only a minor role in determining product formation. This is because other members of the Rb3Ln(PS4)2 and Rb3−xNaxLn(PS4)2 families, Table 2, were synthesized in a RbCl–NaCl eutectic flux (melting point: 486 °C) at a reaction temperature of 600 °C.15 The fact that Rb3Ln(PS4)2 and Rb3−xNaxLn(PS4)2 crystallizes over a wide temperature range suggests that the identity of the flux predominantly impacts the identity of the product formed. Though the use of a RbBr flux at various temperatures resulted in different products formed, it is interesting that the use of a RbCl flux resulted in the same families of compounds. The observation suggests that these families of compounds can be readily targeted using specifically the RbCl flux as at least one component of the flux. Furthermore, it suggests that it is always worthwhile to explore different alkali halide fluxes after a new composition has been found, as the switch in fluxes may easily lead to additional series of compounds.
The single crystal growth of RbEuPS4 was performed using a 1
:
2
:
2 ratio of EuS, P2S5, and Na2S in order to keep the ratio of lanthanide to phosphorus pentasulfide and sodium sulfide the same as in the other syntheses reported herein. The resulting product, which utilized 1 gram of RbCl flux, was phase pure yellow/brown plates of RbEuPS4. It is important to note that in this structure the lanthanide, europium, is in its 2+ oxidation state where all other compounds reported herein have the lanthanides in their 3+ oxidation state. This is most likely the reason that a different compound, structure, and composition, was obtained for europium, as its ability of taking on the 2+ oxidation state is unique among the rare earths described herein.
Structure description
Rb3Pr(PS4)2, and Rb3Ce(PS4)2.
Rb3Pr(PS4)2, and Rb3Ce(PS4)2 belong to the Rb3Ln(PS4)2 family of thiophosphates and crystallize in a one-dimensional structure type in the monoclinic space group P21, Fig. 1a–e. The structures consist of two unique PS43− tetrahedra and one 8-coordinate LnS8 highly distorted square antiprism. The LnS8 polyhedra corner-share with each other creating corrugated infinite chains. Within the chains, the P(1)S43− tetrahedra share two edges with adjacent LnS8 polyhedra and corner share with a third. The P(2)S43− thiophosphate tetrahedra then decorate the sides of the LnS8 polyhedra via face-sharing and point into the channels between the infinite chains. These channels are populated with ordered rubidium cations which hold the infinite chains together resulting in the full structure. In these compounds the Ln–S interatomic distances range from 2.8916(19)–3.253(16) Å, the P–S interatomic distances range from 2.0004(18)–2.089(14) Å, and the Rb–S interatomic distances range from 3.290(17)–3.9974(16) Å.
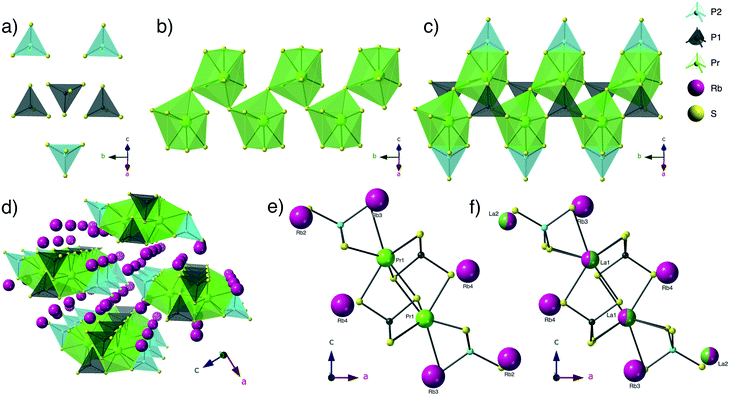 |
| Fig. 1 A schematic of the Rb3Ln(PS4)2 (Ln = La, Ce, Pr) structure showing (a) the two PS43− units, (b) the connectivity of the LnS8 polyhedra, (c) the infinite chains of LnS8 and PS43− polyhedra, and (d) the full crystal structure. Fig. 1e and f show a ball and stick representation of the infinite chains of the (e) Rb3Pr(PS4)2 and (f) Rb3La(PS4)2 structures to visualize where the lanthanum/rubidium site mixing (split pink/green spheres) occurs in Ln = La analogue. | |
Rb3La(PS4)2.
Rb3La(PS4)2 is isostructural with the other Rb3Ln(PS4)2 compounds reported herein however exhibits rubidium/lanthanum site mixing within the structure, Fig. 1f. Of the 4 unique rubidium sites, the Rb1 and Rb2 sites are mixed with lanthanum with La1/Rb1 and La2/Rb2 occupancies of 0.548(4)/0.452(4) and 0.452(4)/0.548(2), respectively, while the Rb3 and Rb4 sites are fully occupied by rubidium. Due to the approximate 50/50 rubidium and lanthanum site mixing, the resulting overall chemical formula is nonetheless the same as those of Rb3Pr(PS4)2 and Rb3Ce(PS4)2. In this compound the Ln–S interatomic distances, impacted by the site mixing, range from 2.976(8)–3.384(6) Å, the P–S interatomic distances range from 1.984(12)–2.108(11) Å, and the Rb–S interatomic distances range from 3.327(8)–3.949(8) Å.
Rb2.45(2)Na0.55(2)Pr(PS4)2 and Rb2.50(6)Na0.50(6)Ce(PS4)2.
Rb2.45(2)Na0.55(2)Pr(PS4)2 and Rb2.50(6)Na0.50(6)Ce(PS4)2 belong to the Rb3−xNaxLn(PS4)2 family of thiophosphates and crystallize in the monoclinic space group P21/c, Fig. 2. The one-dimensional crystal structure consists of one 9-coordinate LnS9 polyhedral unit and two unique PS43− tetrahedra. The LnS9 polyhedra alternate between edge- and face-sharing with one another creating infinite LnS9 chains. Between two face-sharing LnS9 polyhedra, the P(1)S43− tetrahedra face-share with one LnS9 polyhedra and edge-share with the other. Between two edge-sharing LnS9 polyhedra, the P(2)S43− tetrahedra share one edge with each LnS9 polyhedra, see Fig. 2d. The resulting [Ln(PS4)2]3− infinite chains are held together by 3 unique disordered cationic sites. Sites Rb1 (split into Rb1a and Rb1b) and Rb3 (split into Rb3a and Rb3b) contain solely rubidium cations while site Rb2 is a disordered mixed site of both rubidium and sodium cations. In these compounds, the Ln–S interatomic distances range from 2.8731(10)–3.3038(14) Å, the P–S interatomic distances range from 1.996(4)–2.073(3) Å, and the Rb–S interatomic distances, impacted by the site mixing, range from 3.0046(16)–3.958(13) Å.
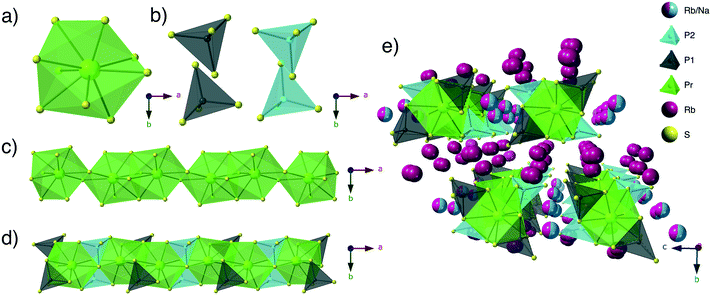 |
| Fig. 2 A schematic of the Rb3−xNaxLn(PS4)2 (Ln = Ce, Pr; x = 0.50, 0.55) structure showing (a) the LnS9 polyhedral unit, (b) the two PS43− units, (c) the connectivity of the rare earth polyhedra, (d) the [Ln(PS4)2]3− infinite chains, and (e) the full crystal structure. | |
RbEuPS4.
RbEuPS4 is the rubidium analogue of KEuPS4 (ref. 36) and crystallizes in the orthorhombic space group Pnma, Fig. 3. The crystal structure consists of one PS43− tetrahedral unit and one 8 coordinate EuS8 bicapped trigonal prism. The EuS8 polyhedra edge-share with each other creating a layer of europium polyhedra parallel to the b–c plane. The edge-sharing of the polyhedra leave trigonal prismatic holes in the layers that are filled by the thiophosphate tetrahedra. The successive layers are connected through rubidium cations which are disordered on their respective sites. In this compound the Eu–S interatomic distances range from 2.9679(16)–3.4516(4) Å, the P–S interatomic distances range from 2.021(2)–2.046(2) Å, and the Rb–S interatomic distances range from 3.327(8)–3.809(9) Å.
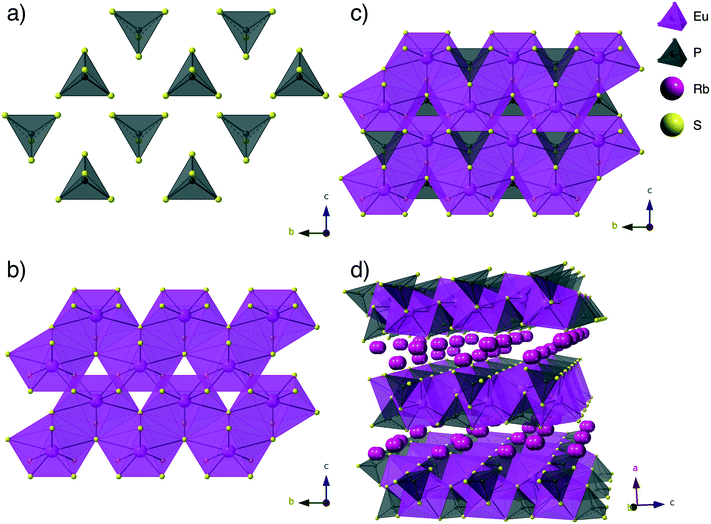 |
| Fig. 3 A schematic of the RbEuPS4 structure showing (a) the PS43− unit, (b) the connectivity of the EuS8 polyhedra, (c) the layers of EuS8 and PS43− polyhedra, and (d) the full crystal structure. | |
Optical properties
Fluorescence spectra were obtained at an excitation wavelength of 375 nm on single crystals of Rb3La(PS4)2, Rb3Pr(PS4)2, Rb3Ce(PS4)2, Rb2.45(2)Na0.55(2)Pr(PS4)2, Rb2.50(6)Na0.50(6)Ce(PS4)2 and RbEuPS4. As expected, there was no fluorescence observed for Rb3La(PS4)2, as lanthanum compounds have a 4f0 electron configuration.45 In the case of RbEuPS4, where europium is 4f7, one might expect fluorescence; however, no fluorescence was observed from the single crystals of RbEuPS4. The fluorescence spectra for Rb3Pr(PS4)2, Rb2.45(2)Na0.55(2)Pr(PS4)2, Rb3Ce(PS4)2, and Rb2.50(6)Na0.50(6)Ce(PS4)2 are shown in Fig. 4. In the Rb3Pr(PS4)2 and Rb2.45(2)Na0.55(2)Pr(PS4)2 spectra, the expected 3P0 → 3H4, 3P0 → 3H5, 1D2 → 3H4, and 3P0 → 3H6 f–f transitions were observed.46 The fluorescence spectra for the Rb3Ce(PS4)2 and Rb2.50(6)Na0.50(6)Ce(PS4)2, exhibit a single broad peak covering much of the visible range measured, a phenomenon reported for other cerium compounds in the literature.47–49
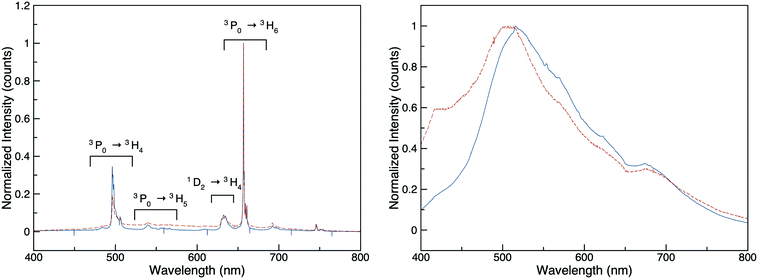 |
| Fig. 4 The emission spectrum of Rb3Pr(PS4)2 (blue solid line) and Rb2.45(2)Na0.55(2)Pr(PS4)2 (orange dashed line) on the left, and that of Rb3Ce(PS4)2 (blue solid line) and Rb2.50(6)Na0.50(6)Ce(PS4)2 (orange dashed line) on the right, taken at an excitation wavelength of 375 nm. | |
Conclusion
Single crystals of new rubidium rare earth thiophosphates with the formulas Rb3Ln(PS4)2 (Ln = La, Ce, Pr), Rb3−xNaxLn(PS4)2 (Ln = Ce, Pr; x = 0.50, 0.55), and RbEuPS4 were crystallized out of a molten RbCl flux. The role of the flux in the creation of rubidium rare earth thiophosphates was discussed and contrasted with other thiophosphate families crystallized out of similar halide fluxes. Fluorescence measurements were performed on all compounds obtained, revealing resolved f–f transitions in Rb3Pr(PS4)2 and Rb2.45(2)Na0.55(2)Pr(PS4)2 and only single broad emission peaks for Rb3Ce(PS4)2 and Rb2.50(6)Na0.50(6)Ce(PS4)2, behavior characteristic for cerium(III) compounds.
Author contributions
Breton – conceptualization, investigation, sample synthesis, structure determination, writing. Smith – structure determination, writing, reviewing. zur Loye – conceptualization, methodology, writing, reviewing.
Conflicts of interest
The authors declare no conflicts of interest.
Acknowledgements
Research supported by the US Department of Energy, Office of Basic Energy Sciences, Division of Materials Sciences and Engineering under award DE-SC0018739.
References
- D. E. Bugaris and H.-C. zur Loye, Angew. Chem., Int. Ed., 2012, 51, 3780–3811 CrossRef CAS.
- J. Boltersdorf, N. King and P. A. Maggard, CrystEngComm, 2015, 17, 2225–2241 RSC.
- C. Juillerat, V. V. Klepov, G. Morrison, K. A. Pace and H.-C. zur Loye, Dalton Trans., 2019, 48, 3162–3181 RSC.
- K. Habermehl, A.-V. Mudring and G. Meyer, Eur. J. Inorg. Chem., 2010, 26, 4075–4078 CrossRef.
- V. V. Klepov, C. Juillerat, K. Pace, G. Morrison and H.-C. zur Loye, Front. Chem., 2020, 8, 518 CrossRef CAS PubMed.
- M. G. Kanatzidis, Inorg. Chem., 2017, 56, 3158–3173 CrossRef CAS.
- Y. Wang, J. Wu, Y. Tang, X. Lu, C. Yang, M. Qin, F. Huang, X. Li and X. Zhang, ACS Appl. Mater. Interfaces, 2012, 4, 4246–4250 CrossRef CAS PubMed.
- F. Lissner, S. P. Meyer and T. Schleid, Z. Naturforsch. B, 2019, 74, 99–107 CrossRef CAS.
- I. Ijjaali, K. Mitchell and J. A. Ibers, J. Solid State Chem., 2004, 177, 760–764 CrossRef CAS.
- Y. Yang and J. A. Ibers, J. Solid State Chem., 2000, 149, 384–390 CrossRef CAS.
- L. S. Breton, V. V. Klepov and H.-C. zur Loye, J. Am. Chem. Soc., 2020, 142, 14365–14373 CrossRef CAS PubMed.
- J. Do, K. Lee and H. Yun, J. Solid State Chem., 1996, 125, 30–36 CrossRef CAS.
- J.-E. Kwak and J.-S. Yun, Bull. Korean Chem. Soc., 2008, 29, 273–275 CrossRef CAS.
- Y. Dong, S. Kim and H. Yun, Acta Crystallogr., Sect. C: Cryst. Struct. Commun., 2005, 61, i25–i26 CrossRef PubMed.
- M. Usman, M. D. Smith, V. Klepov and H.-C. zur Loye, Cryst. Growth Des., 2019, 19, 5648–5657 CrossRef CAS.
- T. Komm and T. Schleid, J. Solid State Chem., 2005, 178, 454–463 CrossRef CAS.
- C. Kutahyali Aslani, L. S. Breton, V. Klepov and H.-C. zur Loye, Dalton Trans., 2021, 50, 1683–1689 RSC.
- L. M. Schoop, R. Eger, J. Nuss, F. Pielnhofer and B. V. Lotsch, Z. Anorg. Allg. Chem., 2017, 643, 1818–1823 CrossRef CAS.
- V. V. Klepov and H.-C. zur Loye, Inorg. Chem., 2018, 57, 11175–11183 CrossRef CAS PubMed.
- M. Tachez, J.-P. Malugani, R. Mercier and G. Robert, Solid State Ionics, 1984, 14, 181–185 CrossRef CAS.
- R. Schlem, P. Till, M. Weiss, T. Krauskopf, S. P. Culver and W. G. Zeier, Chem. – Eur. J., 2019, 25, 4143–4148 CrossRef CAS PubMed.
- Z. Li, X. Jiang, M. Zhou, Y. Guo, X. Luo, Y. Wu, Z. Lin and J. Yao, Inorg. Chem., 2018, 57, 10503–10506 CrossRef CAS PubMed.
- L. Kang, M. Zhou, J. Yao, Z. Lin, Y. Wu and C. Chen, J. Am. Chem. Soc., 2015, 137, 13049–13059 CrossRef CAS.
- E.-Y. Goh, E.-J. Kim and S.-J. Kim, J. Solid State Chem., 2001, 160, 195–204 CrossRef CAS.
- R. F. Hess, P. L. Gordon, C. D. Tait, K. D. Abney and P. K. Dorhout, J. Am. Chem. Soc., 2002, 124, 1327–1333 CrossRef CAS PubMed.
- T. Komm and T. Schleid, Z. Anorg. Allg. Chem., 2006, 632, 42–48 CrossRef CAS.
- T. Komm and T. Schleid, J. Alloys Compd., 2006, 418, 106–110 CrossRef CAS.
- C. Muller, S. Jorgens and A. Mewis, Z. Anorg. Allg. Chem., 2007, 633, 1633–1638 CrossRef.
- V. Manriquez, A. Galdamez and D. Guzman-Aguila, Mater. Res. Bull., 2008, 43, 2469–2475 CrossRef CAS.
- Y. Klawitter, W. Bensch and C. Wickleder, Chem. Mater., 2006, 18, 187–197 CrossRef CAS.
- S. Milot, Y. Wu, C. Nather, W. Bensch and K. O. Klepp, Z. Anorg. Allg. Chem., 2008, 634, 1575–1580 CrossRef CAS.
- T. Komm, S. Strobel and T. Schleid, J. Alloys Compd., 2008, 451, 648–653 CrossRef CAS.
- A. Kuhn, R. Eger, J. Nuss and B. V. Lotsch, Z. Anorg. Allg. Chem., 2014, 640, 689–692 CrossRef CAS.
- V. V. Klepov, L. S. Breton, K. A. Pace, V. Kocevski, T. M. Besman and H.-C. zur Loye, Inorg. Chem., 2019, 58, 6565–6573 CrossRef CAS.
- T. Schleid, I. Hartenbach and T. Komm, Z. Anorg. Allg. Chem., 2002, 628, 7–9 CrossRef CAS.
- C. R. Evenson and P. K. Dorhout, Inorg. Chem., 2001, 40, 2884–2891 CrossRef CAS PubMed.
- L. M. Schoop, R. Eger, R. K. Kremer, A. Kuhn, J. Nuss and B. V. Lotsch, Inorg. Chem., 2017, 56, 1121–1131 CrossRef CAS PubMed.
- V. V. Klepov, M. D. Smith and H.-C. zur Loye, Inorg. Chem., 2020, 59, 1905–1916 CrossRef CAS PubMed.
- M. Ohta, S. Hirai, H. Kato, V. V. Sokolov and V. V. Bakovets, Mater. Trans., 2009, 50, 1885–1889 CrossRef CAS.
- L. Krause, R. Herbst-Irmer, G. M. Sheldrick and D. Stalke, J. Appl. Crystallogr., 2015, 48, 3–10 CrossRef CAS PubMed.
-
APEX3 Version 2019.1–0 and SAINT+ Version 8.40A, Bruker Nano, Inc., Madison, WI, United States, 2019 Search PubMed.
- G. M. Sheldrick, Acta Crystallogr., Sect. A: Found. Adv., 2015, 71, 3–8 CrossRef PubMed.
- G. M. Sheldrick, Acta Crystallogr., Sect. A: Found. Crystallogr., 2008, 64, 112–122 CrossRef CAS PubMed.
- O. V. Dolomanov, L. J. Bourhis, R. J. Gildea, J. A. K. Howard and H. Puschmann, J. Appl. Crystallogr., 2009, 42, 339–341 CrossRef CAS.
- P. S. Peijzel, J. Solid State Chem., 2005, 178, 448–453 CrossRef CAS.
- Y.-F. Lin, Y. H. Chang, Y.-S. Chang, B.-S. Tsai and Y.-C. Li, J. Electrochem. Soc., 2006, 153, G543–G547 CrossRef CAS.
- E. Banks and R. Ward, J. Electrochem. Soc., 1949, 96, 297–303 CrossRef CAS.
- P. N. Hazin, C. Lakshminarayan, L. S. Brinen, J. L. Knee, J. W. Bruno, W. E. Streib and K. Folting, Inorg. Chem., 1988, 27, 1393–1400 CrossRef CAS.
- V. V. Klepov, V. Kocevski, T. M. Besmann and H.-C. zur Loye, CrystEngComm, 2021, 23, 831–840 RSC.
Footnote |
† Electronic supplementary information (ESI) available: The ESI contains SEM images, EDS spectra and obtained elemental compositions, as well as a PXRD of the phase pure RbEuPS4 product, and detailed explanations of the X-ray structure solutions for all compounds reported herein. CCDC 2084750–2084755. For ESI and crystallographic data in CIF or other electronic format see DOI: 10.1039/d1ce00703c |
|
This journal is © The Royal Society of Chemistry 2021 |
Click here to see how this site uses Cookies. View our privacy policy here.