DOI:
10.1039/D0BM01838D
(Review Article)
Biomater. Sci., 2021,
9, 3185-3208
(r)HDL in theranostics: how do we apply HDL's biology for precision medicine in atherosclerosis management?†
Received
28th October 2020
, Accepted 3rd February 2021
First published on 19th March 2021
Abstract
High-density lipoproteins (HDL) are key players in cholesterol metabolism homeostasis since they are responsible for transporting excess cholesterol from peripheral tissues to the liver. Imbalance in this process, due to either excessive accumulation or impaired clearance, results in net cholesterol accumulation and increases the risk of cardiovascular disease (CVD). Therefore, significant effort has been focused on the development of therapeutic tools capable of either directly or indirectly enhancing HDL-guided reverse cholesterol transport (RCT). More recently, in light of the emergence of precision nanomedicine, there has been renewed research interest in attempting to take advantage of the development of advanced recombinant HDL (rHDL) for both therapeutic and diagnostic purposes. In this review, we provide an update on the different approaches that have been developed using rHDL, focusing on the rHDL production methodology and rHDL applications in theranostics. We also compile a series of examples highlighting potential future perspectives in the field.
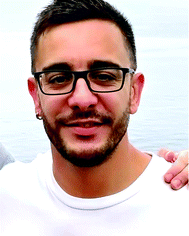 Kepa B. Uribe | Kepa B. Uribe completed his PhD in 2013. His first attempts on research were focused on the study of bacterial protein toxins and their effects on target cells. As a postdoctoral researcher, his studies were centered on different aspects of cholesterol metabolism and, particularly, in the design/development of multifunctional nanoparticles with applications in biomedicine. Nowadays he is working in the design of new hybrid protein-based tools for application in vivo imaging and therapeutics. |
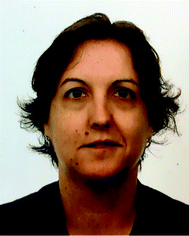 Noemi Rotllan | Noemi Rotllan earned her Ph.D. degree in 2003 from Barcelona University. She did postdoctoral trainee at New York University and Yale University, where she was an Associate Research Scientist. In 2019, she got the prestigious Ramon y Cajal fellowship and she moved back to Barcelona in order to start her own laboratory. Her research interests are focused mainly on the role of new microRNAs in hiperhomocysteinemia related atherosclerosis and to use an innovative therapeutic approach for targeting miRNAs in vivo using rHDL. |
1. Introduction
Cardiovascular disease (CVD) is the leading cause of mortality worldwide, resulting in an estimated global mortality of 17.9 million people each year1 and remains a leading cause despite pharmacological interventions for numerous risk factors.2 The main risk factors for CVD are smoking, age, sex, diabetes, obesity, hypertension and dyslipidaemias.3,4 In the group of dyslipidaemias, dysregulation of cholesterol homeostasis is one of the primary risk factors associated with the development of atherosclerosis, the underlying cause of most CVD. The main particles responsible for cholesterol transport throughout the body are lipoproteins, macromolecular complexes primarily composed of lipids and apolipoproteins. These lipoproteins are classified by density, ranging from very low density lipoproteins (VLDL) to high density lipoproteins (HDL).5 Interestingly, of the different types of lipoproteins capable of cholesterol transport, only HDLs are able to remove excessive cholesterol from peripheral tissues to the liver, a process known as reverse cholesterol transport (RCT).6 Moreover, HDLs also have additional beneficial characteristics that made these lipoproteins attractive candidates for managing or preventing atherosclerosis, such as their small size and ability to interact with other receptors, their ability to encapsulate hydrophobic/hydrophilic molecules, and other antiatherogenic functions. However, the use of natural HDLs has some limitations and in order to overcome them there has been significant research focused on the development of reassembled HDL (rHDL), a field that has grown due to the rise of precision nanomedicine. The potential advantages of these rHDL are wide-ranging, including potential use as natural drug-delivery carriers or contrast agents for medical imaging. This review aims to overview a detailed protocol of the different purification, manipulation and modification methods for developing (r)HDLs. In addition, this review also describes the application of (r)HDL in therapeutics and has compiled the most relevant pre-clinical studies and clinical trials that are using (r)HDLs.
2. HDL in health and disease
2.1 Natural HDL composition and metabolism
In 1960, HDL was first described after human plasma isolation by ultracentrifugation. HDLs are the densest (1.063 < d < 1.21 g mL−1) and smallest (5 nm to 17 nm) plasma lipoproteins. HDL is a protein-rich particle with a hydrophobic core, consisting of cholesteryl esters, triglycerides and unesterified cholesterol, surrounded by a monolayer of phospholipids, unesterified cholesterol and apolipoproteins. HDLs are heterogeneous in shape, size, density, composition and surface charge and can be classified by density (ultracentrifugation), size (gradient gel electrophoresis), surface charge (agarose gel electrophoresis) or apolipoprotein composition (immunoaffinity chromatography) (see Table 1).
Table 1 Classification of HDL subpopulations using different methodologies
UC, ultracentrifugation; GGE, gradient gel electrophoresis; AGE, agarose gel electrophoresis; IAC, immunoaffinity chromatography; LpA-I/A-II, lipoprotein A-I/A-II. |
UC |
HDL2 (1.063 < density < 1.125 g ml−1) |
HDL3 (1.126 < density < 1.210 g ml−1) |
GGE |
HDL2b (mean diameter, 10.6 nm) |
HDL2a (mean diameter, 9.2 nm) |
HDL3a (mean diameter, 8.4 nm) |
HDL3b (mean diameter, 8 nm) |
HDL3c (mean diameter, 7.6 nm) |
AGE |
α-HDL (lipid-containing spherical particles) |
preβ-HDL (lipid-free or lipid-poor ApoA-I) |
IAC |
LpA-I (with ApoA-I but without ApoA-II) |
LpA-I/A-II (with both ApoA-I and ApoA-II) |
HDL particles contain various apolipoproteins, of which ApoA-I and ApoA-II are quantitatively the most abundant.7 In addition to different apolipoproteins, a variety of enzymes and lipid transport proteins are associated with HDL, composing the protein component of this lipoprotein. These proteins, such as lecithin
:
cholesterol acyltransferase (LCAT), cholesteryl ester transfer protein (CETP) and phospholipid transfer protein (PLTP), are crucial for HDL metabolism.
The use of proteomic and lipidomic technologies have enabled the identification of over 85 proteins and more than 200 additional lipids which may reside within HDL particles, as well as genetic material in the form of microRNAs that are associated with HDL.8–10
Briefly, biogenesis of HDL occurs in the liver and intestine, which are able to synthesize and secrete ApoA-I. The first step in the formation of HDL is the interaction of a lipid poor ApoA-I with the ATP binding cassette A1 transporter (ABCA1). ABCA1 is a cholesterol-phospholipid transporter expressed by enterocytes, hepatocytes and other cell types such as macrophages, that allows the nascent HDL to acquire lipids and form preβ-HDL particles. ATP binding cassette G1 transporter (ABCG1) is another protein that plays an important role in regulating cholesterol efflux, although its mechanism of action is still controversial. Next, HDL acquires additional lipids and apolipoproteins derived from the hydrolysis of triglyceride-rich lipoproteins. LCAT acts on cholesterol in nascent HDL particles to generate cholesterol esters, which form the core of the mature HDL particle.
Considering HDL catabolism, there are three main metabolic pathways of cholesterol ester clearance in HDL: (1) through direct uptake by the liver or steroidogenic tissues via the HDL scavenger receptor BI (SR-BI); (2) through specific and non-specific transfer of unesterified cholesterol to LDL;11 or (3) through transfer to apoB-containing lipoproteins by CETP. CETP activity increases the triglyceride component of HDL, which is susceptible to lipolysis by hepatic lipase (HL) and endothelial lipase (EL). Upon modification by these two enzymes, a smaller HDL particle is formed, which is susceptible to faster catabolism. Consequently, HDL consist of a group of particles with marked structural, compositional, physiochemical and functional heterogeneity and with significant differences in their biological activities.
2.2 The role of HDL in CVD protection/development
The main function of HDL in lipid metabolism is to allow RCT, which consists of removing excess cholesterol from peripheral tissues and delivering it to the liver, where cholesterol is redistributed to other tissues or removed from the body in the stool. In addition to this beneficial function, HDL has other anti-atherosclerotic effects such as anti-inflammatory, anti-oxidative, and anti-apoptotic functions and also improves endothelial function.6 Historically, epidemiological studies have shown an inverse association between serum HDL cholesterol (HDL-C) and the risk of cardiovascular events, supporting the theory that HDL is “the good cholesterol”.12,13 Because of this, increasing plasma levels of HDL-C has long been considered a promising complementary therapy to the classical statin-based LDL-C lowering strategy. However, studies conducted in the past decade trying to prove a causal relationship between high HDL-C values and lower CVD risk did not obtain the expected results. On one hand, it has been shown that there is no correlation between epidemiological and genetic studies. There are several monogenetic studies conducted in human individuals carrying genetic variants that naturally condition them to low or high HDL-C levels that have unexpectedly demonstrated that high HDL-C levels are associated with increased risk of coronary heart disease (CAD) and, by contrast, no harmful effects were observed when the concentrations were lower.14,15 In addition, a mendelian randomization study found that although carriers of a loss-of-function variant in the endothelial lipase gene presented with higher HDL-C levels, with similar levels of other lipids and non-lipid risk factors compared with non-carriers, they were not more protected to myocardial infarction than would be expected from observational epidemiology.16 Moreover, a GWAS-type approach that reached hundreds of thousands of people and studied 157 genetic loci associated with lipids levels could not find any association between HDL-C and CAD risk when focused on loci uniquely related to HDL-C levels.17 On the other hand, studies that focused on evaluating the suitability of the chemical intervention to raise HDL-C with therapeutic purposes found this strategy not beneficial. Neither pharmacological CETP inhibition18–20,21 nor niacin administration22,23 resulted in meaningful therapeutic efficacy in the most recent clinical trials.
In part, this set of unfavourable results could be explained by the results of a recent study that has shown the existence of a U-shaped association between HDL cholesterol concentration and all-cause mortality.24 Together, the HDL-C levels hypothesis has evolved to the HDL function hypothesis, arising from evidence that suggests HDL “quality” may be more clinically relevant than “quantity”.25 Thus, the specific composition of HDL particles is crucial to the anti-atherogenic functions mentioned before. As an example, high levels of HDL-C in which HDL is dysfunctional may not only have no clinical benefits but may even increase the risk of CVD. That is why the focus in therapeutics has shifted to increasing the anti-atherogenic function of HDL instead of simply increasing HDL-C levels.26 Recently, it has been considered that a key measure of HDL functionality is HDL cholesterol efflux capacity (CEC) from macrophages. Specifically, a cross-sectional study found that the CEC from macrophages is inversely associated with both carotid intima-media thickness and the likelihood of angiographic CAD, independent of HDL-C levels.27 Some studies have found that increased efflux is associated with reduced risk for CVD,28–30 while other studies did not find this association.31 The discrepancies in these studies may be due to the use of different techniques to quantify CEC.
3. (r)HDL methodology: purification or preparation, manipulation and modification
Since the first papers describing alpha-lipoproteins purification were published in early 1950s using Cohn's method32 and subsequent attempts to their characterization,33 much progress has been made in (r)HDL related laboratory techniques and methodologies. Now, there are multiple well-established protocols for plasma HDL purification, plasma or recombinant ApoA-I purification and rHDL preparation. Similarly, a set of biophysical approaches have been defined as “standards” in order to make rHDL preparations. In the next few sections, some schemes have been included to graphically summarize the different purification/reconstitution methods. In addition, we encourage the reader to look at the “3-step (r)HDL purification/methods” that were included in the ESI† of this article, where these methods have been explained in more detail.
3.1. HDL and ApoA-I purification
Plasma HDL purification.
Plasma is a blood biofluid component primarily consisting of water along with proteins, lipids and other metabolites. It is the main means of lipoproteins transport in their route throughout the body, and therefore represents the major source of HDL and other lipoproteins in purification. Lipoproteins can be obtained by sequential density ultracentrifugation (SD-UC) (Fig. 1) or fast protein liquid chromatography (FPLC). Although the latter separation method more accurately separates different lipoproteins specimens, is not suitable for purification of large amounts of HDL.34,35
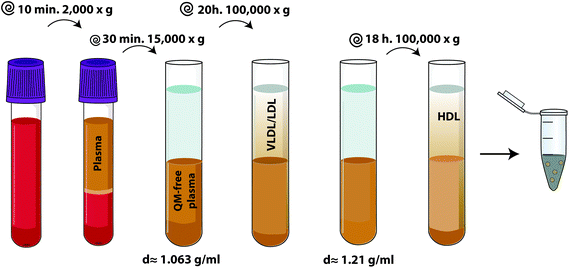 |
| Fig. 1 Plasma HDL purification by SD-UC. A more detailed explanation can be found in the ESI section.† QM, chylomicron; d, density. Purification protocol adapted from ref. 34. | |
ApoA-I purification from plasma.
Some authors have used human-derived ApoA-I directly purified from plasma for their rHDL preparations. This is the case of CSL Behring laboratories, which developed HDL-mimetic nanoparticles (SRC-rHDL/CSL-111 and CSL-112).36,37 This procedure is based on the different properties of stability at varying pH, ethanol concentration, ionic strength, protein concentration and temperature of plasma components (Fig. 2).
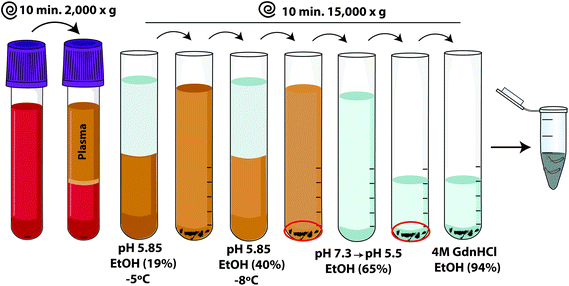 |
| Fig. 2 ApoA-I purification from plasma. A more detailed explanation can be found in the ESI section.† EtOH, ethanol; GdnHCL, guanidinium chloride. Purification protocol adapted from ref. 36 and references included in it. | |
r-ApoA-I purification.
The second primary ApoA-I source is the production and purification of a human recombinant version from bacterial, yeast, mammalian or cell-free optimized systems.38–41 Although concerns could arise from the use of bacterial origin products and their potential immunoreactivity, their use has been proven to be safe. Indeed, companies such as The Medicines Company (MDCO-216)42 and Cerenis Therapeutics (CER-001)43 have chosen this solution to prepare their rHDL formulations. An excellent solution to obtain enough concentration of a good quality human recombinant ApoA-I and in a quick fashion was developed in ref. 44. They engineered a human wild type, full-length and mature form of ApoA-I linked to a N-terminal histidine tag to allow nearly one-step protein purification from E. coli cultures (Fig. 3). Furthermore, the incorporation of cysteine substitutions (i.e. L240C) keeps the functionality of the protein intact and allows its labelling or modification.
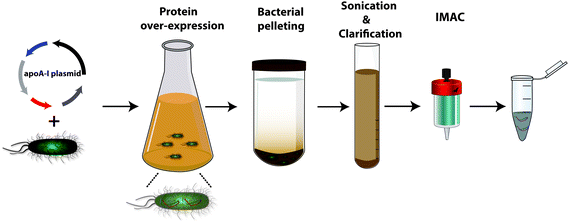 |
| Fig. 3 r-ApoA-I purification from bacterial production system. A more detailed explanation can be found in the ESI section.† IMAC, immobilized metal affinity chromatography. Purification protocol adapted from ref. 44. | |
3.2. rHDL preparation and biophysical characterization
Recombinant HDL nanoparticles are prepared using many different procedures. As lipoprotein-mimetic nanostructures, they consist of two main elements: proteins (or peptides) and lipids. Studies on rHDL formation thermodynamics demonstrated that although rHDL formation is favoured by an enthalpic component (mainly by α-helices folding after protein-lipid complexation), rHDL cannot be formed spontaneously since the activation barrier is markedly high.45 Various strategies to overcome this energetic barrier have led to the development of different methodologies for rHDL synthesis.
Sonication: Sonication was the first documented method used for rHDL formation,46 but current use of this methodology has made some adaptations to the original protocol.47 The rationale of this method is based on the application of ultrasound radiation to a lipid dispersion, which promotes the formation of small unilamellar vesicles (SUV), reducing the activation energy and facilitating ApoA-I incorporation and nanoparticle production (Fig. 4).
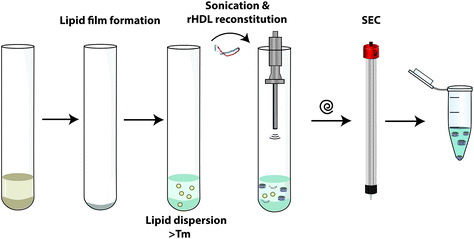 |
| Fig. 4 rHDL preparation/reconstitution by the sonication method. A more detailed explanation can be found in the ESI section.†Tm, lipids phase transition temperature; SEC, size-exclusion chromatography. Reconstitution protocol partially adapted from ref. 47. | |
Sodium cholate dialysis: This method was developed by Matz and Jonas in the 1980s and has been one of the more commonly used methods.48 Conceptually, this technique seeks to generate membrane disruptions by adding a non-denaturing detergent (sodium cholate) to facilitate ApoA-I folding and its insertion into the lipid bilayer, where is leads to generation of rHDL particles (Fig. 5). It is a very effective and versatile methodology for rHDL synthesis since the modification of the initial lipid composition (usually phospholipids, sterols and their derivatives) and/or the ApoA-I
:
lipid mole ratio enables the formation of nanoparticles with different sizes and shapes. This method has been chosen for SRC-rHDL/CSL-111 and CSL112 preparations.36,37
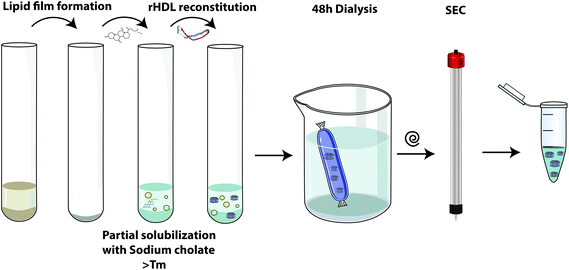 |
| Fig. 5 rHDL preparation/reconstitution by the sodium cholate dialysis method. A more detailed explanation can be found in the ESI section.†Tm, lipid phase transition temperature; SEC, size-exclusion chromatography. Reconstitution protocol adapted from ref. 48. | |
Thermal cycling: Recently, a new methodology has been described for rHDL formation using only ApoA-I peptides and lipids as initial components.49 However, this methodology could also potentially be applied using the whole ApoA-I (Fig. 6). This method reduces the activation barrier by cycling the temperature between lipid phase transition temperature (Tm) and room temperature, facilitating the appearance of discontinuities in the membrane where ApoA-I can be incorporated. This technique generates a uniform nanodisc population and is the most efficient method in terms of low waste generation.
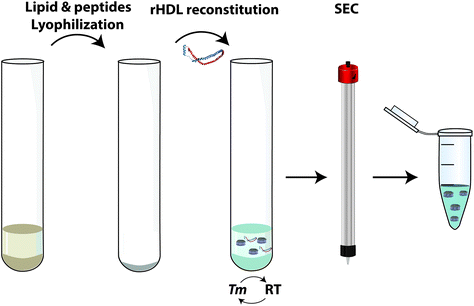 |
| Fig. 6 rHDL preparation/reconstitution by the thermal cycling method. A more detailed explanation can be found in the ESI section.†Tm, lipid phase transition temperature; RT, room temperature; SEC, size-exclusion chromatography. Reconstitution protocol adapted from ref. 49. | |
Microfluidics: In 2013, microfluidics was introduced to the rHDL field in order to produce nanoparticles (denominated as μHDL) in a more controlled fashion with less heterogeneity (Fig. 7).50 This approach starts by directly mixing the lipids, dissolved in an organic solution, with ApoA-I in buffer in a very controlled condition provided by the architecture of a microfluidic device. Once both fluids are injected into the microfluidic device, the transition of lipids from organic to aqueous solution leads to the formation of lipid aggregates in a microvortice that facilitates the spontaneous incorporation of ApoA-I into nanoparticles. Microfluidics has also been applied with ApoA-I mimetic peptides in a recently developed microfluidic platform51 and to the study of membrane proteins incorporated into nanodiscs.52
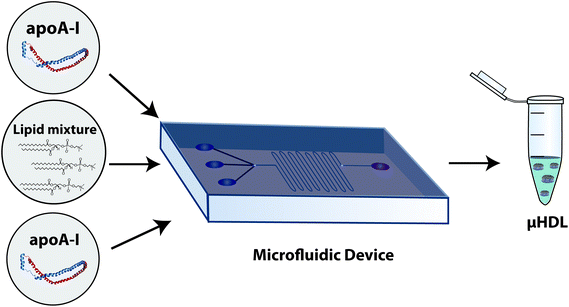 |
| Fig. 7 rHDL preparation/reconstitution by microfluidics. A more detailed explanation can be found in the ESI section.† μHDL, recombinant HDL made by microfluidic devices. Reconstitution protocol adapted from ref. 50. | |
High-pressure homogenization (HPH): Another technique that has been used for rHDL preparations is the HPH procedure (Fig. 8). This is the case of the MDCO-216 formulation.53 This type of methodology is commonly used in industry to prepare nanosuspensions, as it is easily scalable for commercial purposes. HPH is a high-energy consuming procedure where the final components of the preparation are broken down to nanoscale particle size by the application of cavitation, shear and collision forces.54 No specific information is available in the literature regarding how MDCO-216 is produced by HPH. Recently, more research laboratories have started adapting their production methods to the HPH methodology in order to scale up production.55
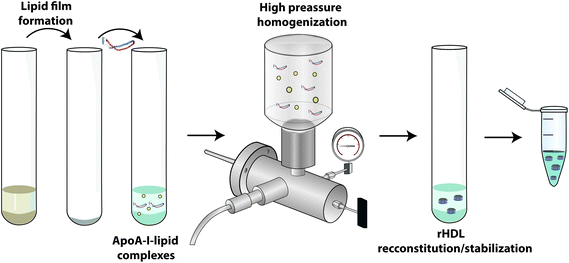 |
| Fig. 8 rHDL preparation/reconstitution by HPH method. A more detailed explanation can be found in the ESI section.† Reconstitution protocol partially adapted from ref. 55. | |
The biophysical characterization of rHDLs has to be designed to analyse predominantly (1) the size (small, medium, large) and morphology (disc vs. sphere) of the particles, (2) the protein/lipid final composition (protein
:
lipid final mole or weight ratio; final lipid composition and proportions; number of ApoA-I molecules per particle), (3) the scaffold protein structure (% alpha-helix), (4) the heterogeneity of the sample (polydispersity index) and (5) the stability of the formulation (aggregation index). Although not needed in routine work, the characterization of rHDL requires a combination of different biophysical techniques in order to have a complete and exhaustive vision of rHDLs. In the case of modified or multifunctional nanoparticles, complementary functional analyses must be undertaken. Even though an in-depth comparative analysis of the different biophysical characterization techniques is not the subject of this review, we will provide some illustrative bibliography of each technique that may serve the reader (see Table 2).
Table 2 Biophysical characterization of (r)HDL
Characteristic to study |
Technique |
Ref. |
AUC, analytical ultracentrifugation; SEC, size-exclusion chromatography; PAGE, polyacrylamide gel electrophoresis; AGE, agarose gel electrophoresis; DLS, dynamic light scattering; NTA, nanoparticle tracking analysis; AFM, atomic force microscopy; TEM, transmission electron microscopy; SAXS, small angle X-ray scattering; SANS, small angle neutron scattering; NMR, nuclear magnetic resonance; HPLC, high-performance liquid chromatography; MS, mass spectroscopy; CD, circular dichroism; ATR-FTIR, attenuated total reflection Fourier transformed infrared spectroscopy. |
Size |
• AUC |
56 and 57 |
• SEC |
58 and 59 |
• Native-PAGE/2D-PAGE |
60 and 61 |
• AGE |
62
|
• DLS |
63
|
• NTA |
64
|
• AFM |
52, 65 and 66 |
• TEM/cryo-TEM |
67 and 68 |
• SAXS/SANS |
69 and 70 |
• NMR |
71 and 72 |
Morphology |
• TEM/cryo-TEM |
67 and 68 |
• AUC |
57
|
• AFM |
52, 65 and 66 |
• NMR |
71 and 72 |
• SAXS/SANS |
69 and 70 |
Protein : lipid composition |
• Biochemical determinations (colorimetric assays) |
|
• Crosslinking/SDS-PAGE |
60 and 73 |
• AUC |
57
|
• SEC |
74
|
• MS |
9
|
Protein structure |
• CD |
75
|
• SANS |
70
|
• NMR |
71 and 72 |
• ATR-FTIR |
59 and 76 |
Polydispersity |
• DLS |
57
|
• AUC |
57
|
Stability |
• ζ-Potential |
77
|
• SEC |
58 and 59 |
• DLS |
57
|
3.3. Tailoring of (r)HDL
The major determinants of the rHDL physicochemical characteristics and hence rHDL functionality are their constituents. In this sense, many different rHDL preparations have been described in which the composition of ApoA-I/peptides, lipids and other modifications or cargo molecules are unique in their configuration, yielding a final particular (r)HDL preparation with its own unique composition and characteristics.
Proteins.
ApoA-I is the main apolipoprotein used as a membrane scaffold protein for rHDL preparations. However, other apolipoproteins such as ApoA-II, ApoA-IV, ApoE, ApoJ, serum amyloid A (SAA) or synuclein variants have been described as potential scaffolds for nanoparticle synthesis.78–83 Wild-type apolipoprotein A-I (UniProtKB #P02647) is a 243 amino acid long and 28 kDa weight amphipathic polypeptide. It is naturally synthesized as pre-pro-ApoA-I, and after being intracellularly processed, adopts the pro-form (pro-ApoA-I), which is secreted for further processing in order to acquire its mature form (ApoA-I). ApoA-I is secreted as lipid-free, or poor-lipidated apolipoprotein, and is further lipidated, generating nascent discoidal HDLs.84,85 A consensus ApoA-I monomeric and lipid free model has been recently described. In this model, ApoA-I is presented as a helical bundle composed by six helix (126 α-helical residues, 52%) organized around the N-ter and two random coil regions, the largest located in the C-ter, that are further folded after being lipidated.86 rHDL are generated using different types of ApoA-I but the predominant types are the human plasma-derived or recombinant mature ApoA-I,37,43 the recombinant pro-ApoA-I form (Pro-ApoA-I-liposome preparation from UCB SA laboratories),87,88 and natural ApoA-I variations such as ApoA-I Milano.42 It has also been synthetized in a trimeric mature ApoA-I form.89
ApoA-I mimetic peptides.
Alternatively, and in order to reduce production costs, the amphipathic alpha-helices of ApoA-I have been used as template to design a set of mimetic peptides with similar properties to those of the whole apolipoprotein, but also with unique features. These peptides do not have sequence homology with ApoA-I but all resemble class A amphipathic helices and have lipid binding capacities similar to ApoA-I. They are able to stimulate ABCA1-mediated cholesterol efflux and have anti-inflammatory and antioxidant properties. To present, there are at least six families of mimetic peptides: the 18A peptide and its derivatives (2F, 4F, 6F, etc.);90,91 the bihelical peptide 37pA and its derivative 5A;92,93 the ETC-642 preparation, composed of the 22A peptide ESP-242118 plus lipids;94 the A10 peptide and its derivatives (S1A10 and S2A10);95 the Fukuoka University ApoA-I Mimetic Peptide (FAMP) family of peptides;96 and mono- or bi-helical ELK peptides.97
Lipids.
Lipidomic studies have shown that the most abundant lipids in HDL, measured as mol% of total, in healthy normolipidemic patients are:9 phospholipids (phosphatidylcholine 32–35%; lysophosphatidylcholine 1.4–8.1%), sphingolipids (sphingomyelin 5.6–6.6%) and neutral lipids (cholesteryl esters 35–37%; free cholesterol 8.7–13.5%; triacylglycerides 2.8–3.2%). Since one of the main goals in rHDL generation has been getting nanoparticles that resemble natural HDL, the commonly used lipid compositions are phosphatidylcholine lipid extracts [Pro-ApoA-I-liposomes: pro-ApoA-I + soybean phosphatidylcholine (SPC); CSL-111/CSL-112: ApoA-I + SPC], pure phospholipids or sphingolipids of different acyl chain length and saturation (MDCO-216: ApoA-I Milano + 16:0/18:2 phosphatidylcholine), or combinations of lipids (CER-001: ApoA-I + sphingomyelin + 16:0 phosphatidylglycerol; ETC-642: ESP24218 peptide + 16:0 phosphatidylcholine + sphingomyelin).36,37,43,48,49,59,88,94,98–100 For diagnostic purposes, researchers have introduced some modified lipids in their rHDL preparations. This is the case in the use of paramagnetic lipids for magnetic resonance imaging (MRI) or phospholipid chelators for positron emission tomography/computed tomography (PET/CT).55,101 Finally, the lipid composition and the protein-to-lipid ratio will influence, among others, size, shape and surface charge which can in turn influence rHDL biological functions.102,103
rHDL modifications.
Due to current increasing interest in HDL-mimetic nanoparticles, the development of new preparations with enhanced functionalities has led to the expansion of rHDL modifications. These modifications have been adopted principally to tune HDL physicochemical properties by (1) enhancing nanoparticle stability in biological fluids (via rHDL PEGylation or lipid cross-linking),104,105 (2) improving HDL targeting (dextran sulphate or hyaluronan modified rHDL) and/or controlling the release of cargo [poly(lactic-co-glycolic acid) modified rHDL],103,106–109 and (3) allowing HDL tracking or its use in medical imaging applications (fluorescently labelled ApoA-I, lipids or hyaluronan; contrast agents for MRI, PET or CT).44,55,100,103,107,108
Cargo molecules.
As mentioned above, rHDL are nanosized particles (8–20 nm) with high tolerability in humans (up to 6.8 g ApoA-I per infusion), long half-life in blood (>24 h) and intrinsic targeting properties.37,74,103 All these advantages have led to rHDLs being considered as excellent nanocarriers for a wide range of cargo molecules. rHDLs can accommodate both hydrophilic compounds adsorbed or attached to the outer shell or hydrophobic compounds entrapped in the core. rHDL cargo can be designed to fulfil diagnostic or therapeutic functions and has been used in studies in multiple fields, ranging from cancer to neurodegenerative research.110–112 Within the CVD field, there are many cargo molecules which have been studied with therapeutic and diagnostic purposes. Among the molecules with therapeutic properties are statins,113,114 LXR agonists,103,115 bioactive lipids,116 immunomodulators,117 siRNA and miRNA molecules,118–120 as well as preparations where more than one active component is combined.121,122 For imaging applications, rHDL has been loaded with fluorescent dyes or quantum dots for optical imaging,44,50,55,103,108,123,124 contrast agents as iron oxide or gadolinium for MRI,50,113,123,125 radionuclides as zirconium-89 (89Zr) for PET,55,103,117 or gold-based rHDL for CT,50,123,126 among others.
4. (r)HDL in theranostics
Nanomedicine has emerged as a powerful tool that promoted a qualitative leap in biomedicine, allowing us to see, understand and modify with a precision level never seen before. It allows us to focus on the nanoscale, but also allows us to respond to daily challenges as well as to those that will come from more comprehensive approaches, in which diagnosis and treatment can be carried out in integrated steps. In this way, theranostics is a term that embraces innovative solutions attempting to combine agents that support “visualizing” and diagnosing the problem together with the appropriate therapeutic in a single compound, preparation or device. One of the prominent tools to study new theranostic approaches are rHDLs, mainly in the field of CVDs, but also in other fields. HDLs are biocompatible lipid–protein complexes that represent a promising scaffold for numerous nanobiotechnological applications and have the potential to become a better drug-delivery platform than liposomes, which are currently the most widely used nanocarriers.127 As mentioned in prior sections, rHDLs are tailored and fully biocompatible nano-platforms prepared in the laboratory that, together with their intrinsic anti-atherosclerotic properties, are ideal candidates for theranostic tool designing due to improved targeted delivery of both therapeutic compounds and/or imaging agents such as contrasts.
Many laboratories have attempted to adapt their rHDL-related translational research towards a more global theranostic approach, although to date only a few attempts have reached human clinical trials. We have recapitulated in the main text some examples of the application of rHDLs as theranostic tool/drug-delivery nanocarrier, but we designed the ESI Table 2† with the intention of giving readers a great number of sample cases where rHDL have been used as nanocarriers on therapeutics. Thus, we encourage readers to visit the additional information arranged in the ESI section.†
4.1. (r)HDL application in therapeutics
The idea of using lipoproteins for therapeutics was first proposed in 1982.128 They have many characteristics that make them promising candidates for therapeutics, such as their small size, ability to interact with different receptors, selective targeting capability, ability to encapsulate hydrophobic molecules, hydrophilic exterior surface, lack of immunogenicity, and extended residence time in circulation.
ApoA-I/HDL infusion.
The first pre-clinical attempts to study the therapeutic roles of (r)HDL in the field of CVD were carried out in the late 1980s studying the effect of homologous HDL infusions. Using adult New Zealand rabbits fed high-cholesterol diet as in vivo atherosclerosis model, the 50 mg per week intravenous (IV) administration of purified HDL isolated from normal rabbits led to a 60% reduction in the development of atherosclerotic-like lipid-rich lesions and significantly decreased lipid depositions in the artery wall compared to control rabbits.129 Moreover, short-term (30 days) treatments of 50 mg per week infusions were able not only to reduce atherosclerosis progression but also to induce atherosclerotic plaque regression by nearly 50%.130 Later in the mid-1990s, an analogous approximation was carried out to test the anti-atherogenic effect of non-lipidated ApoA-I infusions. In a similar atherosclerotic model, the effect of rabbit purified ApoA-I infusions was assessed using measurements of the progression and regression of atheroma lesions. This study found that although 4 × 40 mg per week purified ApoA-I IV administration pattern reduced progression of these lesions by 48%, no regression was observed.131 In the same decade, the natural variant ApoA-I Milano was also tested in vivo. It was known that families carrying this mutation had very low levels of HDL, due to an accelerated catabolism of the apolipoprotein, and a drastically reduced CVD risk. Studies conducted in New Zealand White rabbits on high-cholesterol diet and balloon injury demonstrated that 10 day treatment, in a regiment consisting of a 40 mg per dose every other day, reduced the intimal thickness of injured arteries by 57–71% and reduced the area of the intima covered by macrophages by more than 50%.132
It was not until the 2000s that studies like this were extended to primates and humans. In a first approach, the effect of human purified lipid-free ApoA-I IV bolus injection or continuous infusion was studied in healthy patients with low plasma HDL levels. This study tried to assess the effects, in terms of safety and fate, of ApoA-I after a single-dose (a single injection or a continuous infusion over 5 h of 25 mg kg−1 of ApoA-I) or a multi-dose treatment (6.25–50 mg kg−1 of ApoA-I at different infusion rates over 5 h). The results showed that the procedures were well tolerated with no clinical, biochemical or haematological changes and without evident immune response. ApoA-I concentration in plasma increased in a dose-dependent manner, with a mean half-life of >30 hours, and its presence in plasma was concentrated in HDLs, particularly in pre-β HDLs. Both attempts, ApoA-I bolus injection and continuous infusion, led to significant increases in phospholipid levels in HDL and phospholipid and triglycerides levels in non-HDL fractions but did not alter cholesterol levels in HDL.133 Years later, a second round of trials was performed to test the efficacy of selectively delipidated HDLs (HDL-sdl) to promote reverse cholesterol transport and to promote atherosclerosis regression. By a process in which collected plasma were treated with a series of organic solvents, researchers managed to reduce HDL cholesterol in plasma by 77%, which in turn resulted in enrichment of the pre-β1 HDL subspecies. When primates were infused intravenously with a unique dose of 22–23 mg kg−1 HDL-sdl plasma, these delipidated preβ-1 HDLs were rapidly converted into larger α-HDL with kinetic behaviour depicting the RCT process. Subsequently, when the same HDL-sdl dosage was infused weekly for a period of 12 weeks into monkeys that had developed atherosclerosis, treatment was associated with a significant reduction of 6.9% in the total atheroma volume analysed by IVUS, although the change in the percentage of atheroma volume (PAV) did not reach statistical significance.134 Finally, 28 patients with acute coronary syndrome (ACS) and ≥1 non-obstructive native coronary artery atheroma were enrolled in the LS-001 (Lipid Sciences Selective Delipidation trial) study and treated for 7 weeks with HDL-sdl plasma obtained from each patient plasma, collected by apheresis and reinfused after delipidation. The results showed that administration of the treatment was safe and well-tolerated and, although not reaching statistical significance, the change in mean atheroma volume represented a trend towards reduction which was not observed in the control group.135 In 2018, an innovative solution for treatment administration consisting of oral delivery of “APO milk” was presented. In this study, researchers successfully genetically modified rice plants to express full-length ApoA-I Milano in rice seeds and, after processing, obtained a protein extract containing the therapeutic asset. When tested in an atherosclerosis rodent model as a 15-day treatment consisting of 5 days per week by oral gavage of 0.83 mg kg−1 day−1 dose, there was a significant reduction in atheroma plaque size of 35–50% as well as favourable plaque remodeling.136
Lastly, an interesting pre-clinical study published also in 2018 shed some light into why pre-clinical studies on HDL-raising approaches had not shown the benefits that are presupposed when they were transferred to humans. In this work, there was a focus on the timing of when the ApoA-I/HDL is administered and the effects of ApoA-I infusions in early- vs. late-stage atherosclerotic lesions. ApoE−/− mice on a high-fat diet for 8 weeks (early-stage) or 34 weeks (late-stage) were treated with 40 mg kg−1 lipid-free ApoA-I infusions 2-to-3 times per week. The main result of the study was that while ApoA-I induced plaque size reduction by 30% and improved plaque stability as well HDL functionality in the early-stage model, none of these beneficial effects were observed in the late-stage model. Thus, the atheroprotective effects of ApoA-I/HDL infusions likely depend, at least in part, on the plaque development stage and/or the age of the subject of study.137
ApoA-I mimetic peptides.
Another set of pre-clinical studies has focused on the potential beneficial effects of ApoA-I short mimetic peptide treatment. Due to the large number of papers published with pre-clinical data, those most relevant to atherosclerosis are summarized in the ESI Table 1.† In addition to short peptides, a longer peptide of 54 amino acids derived from the C-terminal region of ApoA-I (peptide RG54) has been shown to be beneficial for glucose control and for inhibiting atherosclerosis.138 Moreover, some of the short mimetic peptides have been tested in humans. The first candidate on this bench-to-bedside journey was ETC-642 in 2002. Two different single-dose studies in atherosclerotic patients and stable cardiovascular patients showed that a single infusion of ETC-642 was safe and well tolerated in a 0.1–20 mg kg−1 dosage. Then, a multiple-dose study was planned at 10–20 mg kg−1 dosages in patients with stable cardiovascular disease, but to date, there is no publicly available data on the results.139 In 2008, the 4F peptide was assessed in humans for the first time. In a phase I trial, patients with stable coronary heart disease (CHD) or equivalent risk were treated with a single oral dose of 30–500 mg of an unformulated D-4F peptide preparation (also known as APP-018; Bruin Pharma Inc.). The study found that although bioavailability was low, the single dose was safe, well tolerated at all concentrations, and could improve the HDL anti-inflammatory index.140 In a subsequent study, the effect of an L-4F peptide preparation (also known as APL180; Novartis Pharmaceuticals) was assayed in patients with stable CHD or equivalent risk in a regimen of 7 days IV infusion or 28 days subcutaneous (SQ) injection. Although the multi-dose IV or SQ administration was well-tolerated at all doses, it was not effective in improving biomarkers of HDL anti-inflammatory function and a trend toward an increase in inflammatory markers (hs-CRP and IL-6) was noted (Clinicaltrials.gov # NCT00568594 and NCT00907998).141 The last study conducted to date was in 2017 and in this occasion, the benefits of treatment with D-4F as an oral multi-dose prescription were assessed. The study subjects were high-risk CHD patients and were treated for 13 days with daily 100–500 mg dose. The D-4F oral treatment was safe and well tolerated and resulted in reducing the HDL inflammatory index by half. Interestingly, the peptide oral dose was highly predictive of HDL inflammatory index improvement while plasma D-4F level was unrelated, leading to the conclusion that peptide penetration in the plasma may be unnecessary.142
rHDL infusions.
The study of rHDLs in a therapeutic context has a long history, and a large number of both pre-clinical studies in diverse disease models and clinical trials in human populations of various conditions have been conducted. Some of the most relevant studies are summarized in the ESI Tables 2 (pre-clinical trials) and 3 (clinical trials).† Although rHDLs have received high commercial interest, only a few compositions have been tested in clinical trials. We describe below the formulations that have been tested in humans.
Pro-ApoA-I liposomes (UCB).
This formulation consisting of human pro-ApoA-I and SPC (1
:
45 mol ratio) demonstrated that a single infusion of 40–50 mg kg−1 in patients with Heterozygous Familial Hypercholesterolemia (HeFH) induced body cholesterol mobilization and excretion with no safety issues.88 Although promising, no other studies with this formulation were found in the literature.
SRC-rHDL (ZLB Central Lab)/CSL-111 (CSL Limited)/CSL112 (CSL Behring).
These are the only formulations prepared with ApoA-I extracted from human plasma and the difference between them lies in the ApoA-I
:
lipid mol ratio. All the formulations contain ApoA-I and parenteral SPC grade; SRC-rHDL and CSL-111 were prepared using 1
:
150 mol ratio while CSL112 was prepared at 1
:
55 mol ratio. Both SRC-rHDL and CSL-111 were the first to be tested and shown that a single dose of 25–80 mg kg−1 promoted body cholesterol mobilization and improved anti-inflammatory markers and atheroma plaque.143,144 In 2007, the phase 2 ERASE trial was conducted with 183 patients with ACS to test the effect of four weekly 40 or 80 mg kg−1 CSL-111 treatment on plaque burden. CSL-111 treatment failed at PAV reduction on IVUS, showing no significant differences compared to placebo. Moreover, the study arm receiving 80 mg kg−1 dose had to be discontinued early due to safety concerns.145 Therefore, the formulation was modified in order to improve its efficacy and safety profile. The new formulation, CSL112, was satisfactorily tested in phase I/2a trials with a favorable efficacy profile and no safety concerns in both single and multiple dosages,37,146–148 but again failed in efficacy when studied in a larger trial. AEGIS-1 was a phase 2b trial completed in 2016 in which four weekly doses of 2 or 6 g ApoA-I per dose were infused in 1258 patients with a recent history of type I myocardial infarction. The results showed that the coprimary safety endpoints, hepatotoxicity rate and renal toxicity, did not show differences when compared to placebo. Although the study was not primarily designed to analyze the efficacy of CSL112, the time to first occurrence of a major adverse cardiovascular event (MACE) was registered during a 12-month follow up in the intent-to-treat group, showing that the risk of MACE was similar to placebo, indicating absence of therapeutic effect.149 Currently, the AEGIS-II study (NCT03473223) is being carried out to analyze the effect of CSL112 in a large population of more than 17
000 people. This study will evaluate the safety and the efficacy of CSL112 in subjects with ACS diagnosis and myocardial infarction and is estimated to be completed by the first half of 2022. Hopefully, the rationale used in the design of the study will enable testing the CVD benefits of an rHDL preparation with improved CEC capacity.150
ETC-216 (Esperion Therapeutics-Pfizer)/MDCO-216 (The Medicines Company-Novartis).
First ETC-216 and later MDCO-216, both were formulated using ApoA-I Milano and 1-palmitoyl-2-oleoyl-glycero-3-phosphocholine (POPC) at a 1
:
40 mol ratio. After an extensive pre-clinical validation, ETC-216 was shown to be able to mobilize body cholesterol in healthy volunteers and was well tolerated up to doses of 50–75 mg kg−1.151 In 2003, the results of the phase II “The ApoA-I Milano Trial” study showed that ETC-216 promoted a mean 2.7% coronary atherosclerosis regression in ACS patients treated with 15 mg kg−1 or 45 mg kg−1 IV infusions.152 Despite these promising results, clinical development of ETC-216 was discontinued due to serious adverse effects in some patients. After significant improvements in the manufacturing process, the new formulation MDCO-216 was tested and proven to be safe in terms of immunostimulatory effects.153 Therefore, the phase I/II MILANO-PILOT study was designed to assess the efficacy of 5 weekly 20 mg kg−1 MDCO-216 IV infusions in statin-treated patients with ACS. The analysis of the results showed that although MDCO-216 tended to reduce the PAV, the reduction was not significantly different from placebo.154 Hence, it became clear that MDCO-216 treatment was not effective in patients with established and intensive ACS treatment as it was not able to reduce the residual risk in these patients. A second phase II trial, MILANO-DRIVE, was designed to be conducted in subjects with CAD and recent ACS, but it was discontinued in 2016 due to lack of efficacy. After that, The Medicine Company stopped investigating MDCO-216 in 2016.
CER-001 (Cerenis Therapeutics).
CER-001 represents the most complex formulation. The scaffold protein is a recombinant version of human ApoA-I produced in eukaryotic cultures and the lipid mixture is composed of sphingomyelin and 1,2-dipalmitoyl-sn-glycero-3-phospho-(1′-rac-glycerol) (DPPG). The final mol ratio of ApoA-I
:
SM
:
DPPG is of 1
:
103
:
3. In 2013 the phase II CHI SQUARE trial was completed in ACS patients to test the efficacy of the treatment on total atheroma volume reduction by the end of the study compared to baseline. CER-001 was not effective and there were some safety issues related to the treatment in some study subjects.43 Nevertheless, CER-001 was further tested in a new phase II MODE trial in patients with phenotypic characteristics of homozygous familial hypercholesterolemia (HoFH). Unlike what was observed in the CHI-SQUARE trial, the results of the MODE trial were favorable and found that 12 biweekly treatments at 8 mg kg−1 dose resulted in a significant carotid mean vessel wall area reduction.155 A post-hoc analysis of data extracted from the CHI-SQUARE trial showed that the subjects with the most extensive plaque-burden (baseline plaque atheroma volume ≥30%) receiving 3 mg kg−1 had the greatest benefit since the treatment with CER-001 was statistically effective compared to placebo in these conditions.156 Hence, a phase II CARAT trial was attempted in ACS patients with these characteristics. In this trial, patients on statin-therapy were administered 10 weekly doses of 3 mg kg−1 CER-001 by IV infusion and the efficacy on reduction of PAV from baseline was analyzed after 78 days. Once again, the results were not favorable as CER-001 was unable to promote atherosclerosis regression.157 The last of the assays with CER-001 on humans carried out in the phase II SAMBA trial was focused on determining CER-001 effects on RCT and the arterial vessel wall on patients with Familial Hypoalphalipoproteinemia (FHA). The study concluded that a 28 infusion treatment mobilized body lipids, with a positive trend toward increased fecal sterol excretion, but more importantly, it was effective in thinning the carotid artery vessel wall and reducing arterial wall inflammation.158 In 2018, a phase III TANGO trial was designed to be carried out in a larger population of FHA patients but, due to lack of efficacy, it was terminated prematurely.
Other preparations.
Many other innovative formulations developed in recent years have only been tested in vitro and in animal models, with some of them showing high potential for further evaluation in clinical trials. Most of the new rHDL preparations sought to exploit their characteristics as specific and targetable nanocarriers to “load” them with therapeutically active compounds (e.g. statins, immunomodulatory drugs or siRNA/miRNA molecules) and enhance their intrinsic efficacy, reducing in turn the side effects of the payloads.113,117,122 On the other hand, other preparations sought to increase the residence time of rHDL-cargo in the system in order to increase the vector's therapeutic effect (e.g. rHDL PEGylation, LCAT inhibitors or cargo liberation controlling polymers) or improve/adapt its targeting capacity (e.g. hyaluronic acid or anionic lipids), thus limiting cargo release in unwanted cells/tissues.104,121,159,160
4.2. (r)HDL application in diagnostics
Similar to the applications of rHDLs in therapeutics, several works have shown an increasing role of rHDL in diagnostics too. Although the focus of this review is the application of rHDL in atherosclerotic cardiovascular disease, increasing evidence suggests that rHDLs could be successfully used as diagnostic tools in additional fields such as oncology. This feature is due to their nanoscale size, their receptor-guided specificity and their capacity to be loaded with contrast agents or tracers of diverse natures. In the case of atherosclerosis, these advantages enable specifically targeting the tracer to the atheroma plaque, penetrating into it and marking the regions where lesions are in an active state. At the pre-clinical level, rHDLs have been tested for use with MRI, CT, PET and Near-infrared (NIR) imaging for diagnostic applications.
The development of the rHDLs was initially dominated by their potential role in therapeutics, which is why it was not until the 21st century that rHDLs were evaluated in diagnostic in vivo studies. Preliminary attempts studied the biodistribution of 125I-HDLs in animal models and their binding to regions of the aorta especially affected by lipid depositions by autoradiography.161 Since 2004, however, rHDLs have begun to be systematically used as in vivo imaging tools. That year and for the first time, the combination of a gadolinium chelating modified phospholipid (Gd3+-DTPA-DMPE) with rHDLs was shown to be a useful contrast agent for MRI-based atheroma plaque detection, specifically in atherosclerosis model animals.162 Similar positive results were obtained when Gd3+-DTPA-DMPE was reconstituted in disk-shaped instead of spherical rHDLs or when full ApoA-I was substituted by the 18A or 37pA mimetic peptides.163–165 Subsequent studies showed a significant improvement in MRI signal when substituting the ApoA-I for its oxidized form, apparently due to an increased uptake by plaque macrophages.166,167 Furthermore, it is feasible to track the signal of ApoA-I or any of its mimetic peptides in vivo by covalently binding Gd3+ to ApoA-I by selective cysteine substitution, zirconium-89 to lysine residues via deferoxamine B conjugation (89Zr-AI-HDL) or gallium-68 radiolabeling of FAMP peptides (68Ga-DOTA-FAMP).168–170 The synthesis of a phospholipid coordinated to 68Ga for its use with rHDL (68Ga-PCTA-DSPE-HDL) has also been reported.171 In 2009, a new rHDL nanoplatform concept was designed for imaging purposes by combining Au, FeO or quantum dots (QD) nanocrystal core with a phospholipid corona bearing fluorescent or paramagnetic lipids. When tested, it was shown that this multimodal contrast agent was useful for in vivo T1- and T2-weighted MRI, CT and fluorescence imaging.123 The in vivo application of Au-rHDL was further validated in a multicolor CT study in combination with other contrast agents.172 The last step carried out in imaging mediated pre-clinical diagnostics is the multimodal approach. Thus, in a study published in 2019, the authors showed how combining 89Zr-rHDL and other functional radiotracers with PET, CT, MRI and NIRF imaging allows studying the pharmacokinetics, biodistribution, and the anti-atherosclerotic effects of an rHDL-based nanoimmunotherapy.55
To date, only a single human imaging study related to atherosclerosis has been conducted, the Netherlands Trial Registry – NTR5178, with the purpose of studying in vivo the delivery of 89Zr-labelled CER-001 to atheroma plaques of patients with CAD. After a single infusion of 3 mg kg−1 of CER-001 together with 89Zr-CER-001 (10 mg–18 MBq), patients were monitored by serial PET/CT imaging and contrast-enhanced MRI for 48 hours. The study concluded that CER-001 targets the atheroma plaque and that is actively uptaken by cells, and can correlate with plaque contrast enhancement.173
5. Concluding remarks
CVD management continues to be one of the leading biomedical challenges of recent times, in part due to the rise of ageing populations, as well as the growing trend of CVD-promoting lifestyle factors. The pharmacologic objectives that have been pursued for atherosclerosis prevention and regression include reduction of the underlying systemic inflammation and correction of the dysregulation generated by increases in atherogenic lipid fractions. Cholesterol is one of the central lipid molecules whose dysregulation is associated with CVD development. Guided by knowledge acquired about cholesterol metabolism, two basic strategies have been implemented: reducing pro-atherogenic particles rich in cholesterol (“bad cholesterol”) or increasing those particles whose purpose is to remove excess cholesterol (“good cholesterol”). HDLs are included in this second group and for that reason have attracted the attention of many investigators as potential therapeutic targets. HDLs are a dynamic and heterogenous group of lipoproteins whose main function is to redistribute excess cholesterol from peripheral tissues to the liver to be ultimately excreted as stool, a process known as reverse cholesterol transport.
Although the epidemiological studies identified a positive correlation between increased HDL-C levels and a reduction in CVD risk, subsequent genetic studies and large clinical trials with HDL-C promoting drugs did not obtain positive expected results that demonstrated a clear causal relationship. Furthermore, it has been described that certain HDL populations could have pro-atherogenic properties. For these reasons, the exogenous administration of purified HDLs or reconstituted (r)HDLs has been proposed as an alternative therapeutic solution that could accommodate current “quality” parameters by designing or modifying rHDL on demand. As has come up throughout this review, this is a key feature rHDLs offer to researchers: the capability to improve on the quality of HDLs through the enhancement of current anti-atherosclerotic capabilities or by acquiring new properties through incorporation of specific compounds. Inspired by this, we may name such optimized rHDL particles as tailored or “smart” rHDLs.
Of all the proposed rHDLs studied in vitro, only four rHDL formulations (Pro-ApoA-I liposomes; SRC rHDL/CSL-111/CSL112; ETC-216/MDCO-216; CER-001) have been studied in humans. After thorough validation in pre-clinical studies, they have been predominantly tested in patient cohorts with CVD as a single or multi-weekly IV dose regimen. Unfortunately, results have not been satisfactory for most of these candidates. In fact, only one Phase 3 study (AEGIS-II) sponsored by CSL Behring in a cohort of 17
400 patients with ACS is currently taking place. The lack of statistically significant outcomes in large clinical trials to date has led to doubts about the clinical application of this therapeutic strategy. It is noteworthy however that two of the successful earlier-stage studies were focused on familial hypercholesterolemia, a disease in which we know there is an impairment of reverse cholesterol transport.11 Additionally, in animal models, HDL has shown to be more protective in initial that advanced lesions. Given the current knowledge that modified HDL can be converted into pro-inflammatory particles with impaired reverse cholesterol transport capacities, this could at least partially explain the limited advances towards clinical application so far. In contrast, there is accumulating evidence that is positioning the tailored manufactured, new generation of rHDLs as suitable biocompatible nanocarriers for the development of future theranostic approaches (Fig. 9 and ESI Tables 1 and 2†). Although none of these advanced formulations have been tested on humans yet, accumulating evidence from both in vitro and in pre-clinical studies has demonstrated promise. For atherosclerosis management, the designed tailored rHDL undergo modifications/adaptations that enhance their atheroprotective function. In pre-clinical studies, newly assayed ApoA-I variations (e.g. V156K variant or trimeric ApoA-I) or an improved generation of mimetic peptides (e.g. the trimeric A-10 or i-FAMP-D1 peptides) have better functional profiles than their predecessors. Also, it has been recently demonstrated in vitro that different lipid compositions of rHDL can modulate cholesterol efflux activity stimulation,174 thus demonstrating the importance of optimal lipid compositions of rHDL in maximizing cholesterol efflux and achieving therapeutic objectives. In addition to optimizations of rHDL components, rHDL has also been improved through chemical modifications or addition of active compounds that enhance rHDL's biodistribution and bioavailability (e.g. rHDL PEGylation or addition of gangliosides, hyaluronic acid or LCAT activity modulators). On the other hand, what is more interesting is the incorporation of active compounds into rHDLs with therapeutic purposes, since rHDLs gather drug-delivery nanocarrier properties. The combination of rHDL with statins, immunomodulatory drugs or siRNA/miRNA molecules has a profound synergistic effect, as it improves the efficacy parameters compared to treatment with bare rHDL. Specifically, when a bioactive compound is given in conjunction with rHDLs, the effects of the carried compound occurs more locally, where it is needed, and unwanted side effects are minimized. A clear example of this is noted when compared the efficacy of the treatments of the ETC-642 formulation with the LXR agonist T1317 inside or administered in parallel, as it is published very recently.175 In both cases the concomitant administration of rHDL + T1317 shows a positive synergistic effect, although it is clearly superior in terms of atheroma burden reduction the treatment of the loaded nanocarrier.
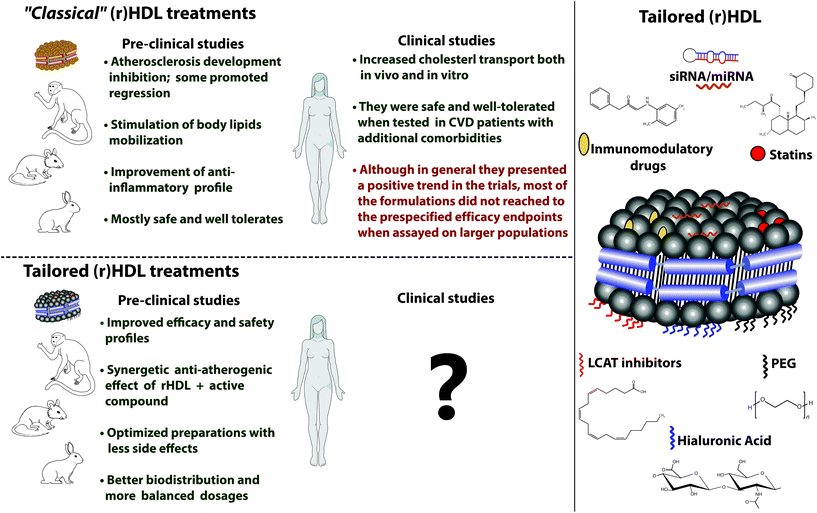 |
| Fig. 9 Scheme/summary of the main results obtained in the different pre-clinical and clinical studies and some of the innovative modifications applied to rHDLs. To have a broader view of the studies carried out as well as the particular formulations that have been studied, we encourage the reader to see the different tables in the ESI section.† | |
Complementing the advances in the therapeutic plane and giving it the dimension of a theranostic tool, tailored rHDL have also been developed to fulfil needs in imaging-based studies. In this sense, rHDL have been specifically labelled for PET, MRI, CT or NIRF imaging, leading to demonstration of rHDL's usefulness not only for diagnosis but also as a tracer for functional studies. A great example of the potency of tailored rHDL as a theranostic tool is the very recently published work carried out by Binderup and colleagues55 and which will be called to serve as an example for the next developments.
For future, the development of a new generation of tailored rHDL with improved characteristics and added functionalities that, in general, constitute better “quality” HDL should serve to attract the attention of researchers and investors and relaunch interest in the development of new clinical trials in humans to assess the capabilities of optimized rHDL as a theranostic tool.
Abbreviations
ACS | Acute coronary syndrome |
Apo | Apolipoprotein |
ABCA1/G1 | ATP-binding cassette A1 or G1 transporter |
CAD | Coronary artery disease |
CEC | Cholesterol efflux capacity |
CHD | Coronary heart disease |
CVD | Cardiovascular disease |
CETP | Cholesteryl ester transfer protein |
CT | Computed tomography |
DOTA | 1,4,7,10-Tetraazacyclododecane-1,4,7,10-tetraacetic acid |
DMPE | 1,2-Dimyristoyl-sn-glycero-3-phosphoethanol-amine |
DPPG | 1,2-Dipalmitoyl-sn-glycero-3-phospho-(1′-rac-glycerol) |
DSPE | 1,2-Distearoyl-sn-glycero-3-phosphoethanolamine |
DTPA | Diethylenetriamine penta acetic acid |
EL | Endothelial lipase |
FAMP | Fukuoka apolipoprotein A-I mimetic peptide |
FH | Familial hypercholesterolemia |
FHA | Familial hypoalphalipoproteinemia |
FPLC | Fast performance liquid chromatography |
HDL/HDL-C | High-density lipoprotein/HDL-associated cholesterol |
HeFH/HoFH | Heterozygous/homozygous familial hypercholesterolemia |
HL | Hepatic lipase |
HPH | High-pressure homogenization |
IV | Intravenous |
IVUS | Intravascular ultrasound |
LCAT | Lecithin : cholesterol acyltransferase |
LDL/LDL-C | Low-density lipoprotein/LDL-associated cholesterol |
LXR | Liver X receptor |
MACE | Major adverse cardiovascular event |
μHDL | rHDL produced with microfluidics techniques |
miRNA | micro-RNA |
MRI | Magnetic resonance imaging |
NIR | Near-infrared |
PAV | Percentage of atheroma volume |
PCTA | Polycyclohexylenedimethylene terephthalate acid |
PEG | Polyethylene glycol |
PET | Positron emission tomography |
PLTP | Phospholipid transfer protein |
POPC | 1-Palmitoyl-2-oleoyl-glycero-3-phosphocholine |
pro-ApoA-I | Pro-form of apolipoprotein A-I |
QD | Quantum dot |
r-ApoA-I | Recombinant version of apolipoprotein A-I |
RCT | Reverse cholesterol transport |
(r)HDL | Combination of recombinant and natural HDL |
SAA | Serum amyloid A |
SD-UC | Sequential density ultracentrifugation |
SEC | Size-exclusion chromatography |
siRNA | Small interfering RNA |
SM | Sphingomyelin |
SPC | Soybean L-α-phosphatidylcholine |
SQ | Subcutaneous |
SR-BI | Scavenger receptor class B type I |
SUV | Small-unilamellar vesicle |
T
m
| Phase transition temperature |
VLDL | Very low-density lipoprotein |
Author contributions
K. B. U., A. B-V. and N. R. drafted the manuscript, which was critically reviewed by C. M. and F. B-V. All authors have read and agreed to the published version of the manuscript.
Funding
This review was funded by Ministerio de Ciencia, Innovación y Universidades (PID2019-104367RB-100), as well as the Subprograma Ramón y Cajal (RYC-201722879) to N.R. and PI18/0164 to F.B.-V., FEDER “Una manera de hacer Europa”. CIBER de Diabetes y Enfermedades Metabólicas Asociadas (CIBERDEM) is a project of Instituto de Salud Carlos III. Institut de Recerca de l'Hospital de la Santa Creu i Sant Pau is accredited by the Generalitat de Catalunya as Centre de Recerca de Catalunya (CERCA). This work was also supported by the Basque Government (Grupos Consolidados IT-1264-19) to C.M. and A.B.-V. was supported by Programa de especialización de Personal Investigador Doctor en la UPV/EHU (2019) 2019–2020.
Conflicts of interest
The authors declare no conflicts of interest.
Acknowledgements
The authors would like to thank Haziq Siddiqi for manuscript English editing.
References
-
World Health Organization, Cardiovascular diseases (CVDs), 2020 Search PubMed.
- R. L. Sacco, G. A. Roth, K. S. Reddy, D. K. Arnett, R. Bonita, T. A. Gaziano, P. A. Heidenreich, M. D. Huffman, B. M. Mayosi, S. Mendis, C. J. Murray, P. Perel, D. J. Pineiro, S. C. Smith Jr., K. A. Taubert, D. A. Wood, D. Zhao and W. A. Zoghbi, The Heart of 25 by 25: Achieving the Goal of Reducing Global and Regional Premature Deaths From Cardiovascular Diseases and Stroke: A Modeling Study From the American Heart Association and World Heart Federation, Circulation, 2016, 133, e674–e690 CrossRef PubMed.
- M. F. Piepoli, A. W. Hoes, S. Agewall, C. Albus, C. Brotons, A. L. Catapano, M. T. Cooney, U. Corra, B. Cosyns, C. Deaton, I. Graham, M. S. Hall, F. D. R. Hobbs, M. L. Lochen, H. Lollgen, P. Marques-Vidal, J. Perk, E. Prescott, J. Redon, D. J. Richter, N. Sattar, Y. Smulders, M. Tiberi, H. B. van der Worp, I. van Dis, W. M. M. Verschuren, S. Binno and E.S.C.S.D. Group, 2016 European Guidelines on cardiovascular disease prevention in clinical practice: The Sixth Joint Task Force of the European Society of Cardiology and Other Societies on Cardiovascular Disease Prevention in Clinical Practice (constituted by representatives of 10 societies and by invited experts)Developed with the special contribution of the European Association for Cardiovascular Prevention & Rehabilitation (EACPR), Eur. Heart J., 2016, 37, 2315–2381 CrossRef PubMed.
- F. Mach, C. Baigent, A. L. Catapano, K. C. Koskinas, M. Casula, L. Badimon, M. J. Chapman, G. G. De Backer, V. Delgado, B. A. Ference, I. M. Graham, A. Halliday, U. Landmesser, B. Mihaylova, T. R. Pedersen, G. Riccardi, D. J. Richter, M. S. Sabatine, M. R. Taskinen, L. Tokgozoglu, O. Wiklund and E.S.C.S.D. Group, 2019 ESC/EAS Guidelines for the management of dyslipidaemias: lipid modification to reduce cardiovascular risk, Eur. Heart J., 2020, 41, 111–188 CrossRef PubMed.
- P. Alaupovic, Significance of apolipoproteins for structure, function, and classification of plasma lipoproteins, Methods Enzymol., 1996, 263, 32–60 CAS.
- A. von Eckardstein, J. R. Nofer and G. Assmann, High density lipoproteins and arteriosclerosis. Role of cholesterol efflux and reverse cholesterol transport, Arterioscler., Thromb., Vasc. Biol., 2001, 21, 13–27 CrossRef CAS PubMed.
- P. T. Duong, H. L. Collins, M. Nickel, S. Lund-Katz, G. H. Rothblat and M. C. Phillips, Characterization of nascent HDL particles and microparticles formed by ABCA1-mediated efflux of cellular lipids to apoA-I, J. Lipid Res., 2006, 47, 832–843 CrossRef CAS PubMed.
- A. S. Shah, L. Tan, J. L. Long and W. S. Davidson, Proteomic diversity of high density lipoproteins: our emerging understanding of its importance in lipid transport and beyond, J. Lipid Res., 2013, 54, 2575–2585 CrossRef CAS PubMed.
- A. Kontush, M. Lindahl, M. Lhomme, L. Calabresi, M. J. Chapman and W. S. Davidson, Structure of HDL: particle subclasses and molecular components, Handb. Exp. Pharmacol., 2015, 224, 3–51 CAS.
- K. C. Vickers, B. T. Palmisano, B. M. Shoucri, R. D. Shamburek and A. T. Remaley, MicroRNAs are transported in plasma and delivered to recipient cells by high-density lipoproteins, Nat. Cell Biol., 2011, 13, 423–433 CrossRef CAS PubMed.
- L. Cedo, J. Metso, D. Santos, A. Garcia-Leon, N. Plana, S. Sabate-Soler, N. Rotllan, A. Rivas-Urbina, K. A. Mendez-Lara, M. Tondo, J. Girona, J. Julve, V. Pallares, A. Benitez-Amado, V. Llorente-Cortes, A. Perez, D. Gomez-Coronado, A. K. Ruotsalainen, A. L. Levonen, J. L. Sanchez-Quesada, L. Masana, P. T. Kovanen, M. S. Jauhiainen, M. Lee-Rueckert, F. Blanco-Vaca and J. C. Escola-Gil, LDL Receptor Regulates the Reverse Transport of Macrophage-Derived Unesterified Cholesterol via Concerted Action of the HDL-LDL Axis: Insight from Mouse Models, Circ. Res., 2020, 127, 778–792 CrossRef CAS PubMed.
- T. Gordon, W. P. Castelli, M. C. Hjortland, W. B. Kannel and T. R. Dawber, High density lipoprotein as a protective factor against coronary heart disease. The Framingham Study, Am. J. Med., 1977, 62, 707–714 CrossRef CAS PubMed.
- W. P. Castelli, R. J. Garrison, P. W. Wilson, R. D. Abbott, S. Kalousdian and W. B. Kannel, Incidence of coronary heart disease and lipoprotein cholesterol levels. The Framingham Study, JAMA, J. Am. Med. Assoc., 1986, 256, 2835–2838 CrossRef CAS.
- R. Frikke-Schmidt, B. G. Nordestgaard, M. C. Stene, A. A. Sethi, A. T. Remaley, P. Schnohr, P. Grande and A. Tybjaerg-Hansen, Association of loss-of-function mutations in the ABCA1 gene with high-density lipoprotein cholesterol levels and risk of ischemic heart disease, JAMA, J. Am. Med. Assoc., 2008, 299, 2524–2532 CrossRef CAS PubMed.
- P. Zanoni, S. A. Khetarpal, D. B. Larach, W. F. Hancock-Cerutti, J. S. Millar, M. Cuchel, S. DerOhannessian, A. Kontush, P. Surendran, D. Saleheen, S. Trompet, J. W. Jukema, A. De Craen, P. Deloukas, N. Sattar, I. Ford, C. Packard, A. Majumder, D. S. Alam, E. Di Angelantonio, G. Abecasis, R. Chowdhury, J. Erdmann, B. G. Nordestgaard, S. F. Nielsen, A. Tybjaerg-Hansen, R. F. Schmidt, K. Kuulasmaa, D. J. Liu, M. Perola, S. Blankenberg, V. Salomaa, S. Mannisto, P. Amouyel, D. Arveiler, J. Ferrieres, M. Muller-Nurasyid, M. Ferrario, F. Kee, C. J. Willer, N. Samani, H. Schunkert, A. S. Butterworth, J. M. Howson, G. M. Peloso, N. O. Stitziel, J. Danesh, S. Kathiresan, D. J. Rader, C. H. D. E. Consortium, C. A. E. Consortium and C. Global Lipids Genetics, Rare variant in scavenger receptor BI raises HDL cholesterol and increases risk of coronary heart disease, Science, 2016, 351, 1166–1171 CrossRef CAS PubMed.
- B. F. Voight, G. M. Peloso, M. Orho-Melander, R. Frikke-Schmidt, M. Barbalic, M. K. Jensen, G. Hindy, H. Holm, E. L. Ding, T. Johnson, H. Schunkert, N. J. Samani, R. Clarke, J. C. Hopewell, J. F. Thompson, M. Li, G. Thorleifsson, C. Newton-Cheh, K. Musunuru, J. P. Pirruccello, D. Saleheen, L. Chen, A. Stewart, A. Schillert, U. Thorsteinsdottir, G. Thorgeirsson, S. Anand, J. C. Engert, T. Morgan, J. Spertus, M. Stoll, K. Berger, N. Martinelli, D. Girelli, P. P. McKeown, C. C. Patterson, S. E. Epstein, J. Devaney, M. S. Burnett, V. Mooser, S. Ripatti, I. Surakka, M. S. Nieminen, J. Sinisalo, M. L. Lokki, M. Perola, A. Havulinna, U. de Faire, B. Gigante, E. Ingelsson, T. Zeller, P. Wild, P. I. de Bakker, O. H. Klungel, A. H. Maitland-van der Zee, B. J. Peters, A. de Boer, D. E. Grobbee, P. W. Kamphuisen, V. H. Deneer, C. C. Elbers, N. C. Onland-Moret, M. H. Hofker, C. Wijmenga, W. M. Verschuren, J. M. Boer, Y. T. van der Schouw, A. Rasheed, P. Frossard, S. Demissie, C. Willer, R. Do, J. M. Ordovas, G. R. Abecasis, M. Boehnke, K. L. Mohlke, M. J. Daly, C. Guiducci, N. P. Burtt, A. Surti, E. Gonzalez, S. Purcell, S. Gabriel, J. Marrugat, J. Peden, J. Erdmann, P. Diemert, C. Willenborg, I. R. Konig, M. Fischer, C. Hengstenberg, A. Ziegler, I. Buysschaert, D. Lambrechts, F. Van de Werf, K. A. Fox, N. E. El Mokhtari, D. Rubin, J. Schrezenmeir, S. Schreiber, A. Schafer, J. Danesh, S. Blankenberg, R. Roberts, R. McPherson, H. Watkins, A. S. Hall, K. Overvad, E. Rimm, E. Boerwinkle, A. Tybjaerg-Hansen, L. A. Cupples, M. P. Reilly, O. Melander, P. M. Mannucci, D. Ardissino, D. Siscovick, R. Elosua, K. Stefansson, C. J. O'Donnell, V. Salomaa, D. J. Rader, L. Peltonen, S. M. Schwartz, D. Altshuler and S. Kathiresan, Plasma HDL cholesterol and risk of myocardial infarction: a mendelian randomisation study, Lancet, 2012, 380, 572–580 CrossRef CAS.
- C. J. Willer, E. M. Schmidt, S. Sengupta, G. M. Peloso, S. Gustafsson, S. Kanoni, A. Ganna, J. Chen, M. L. Buchkovich, S. Mora, J. S. Beckmann, J. L. Bragg-Gresham, H. Y. Chang, A. Demirkan, H. M. Den Hertog, R. Do, L. A. Donnelly, G. B. Ehret, T. Esko, M. F. Feitosa, T. Ferreira, K. Fischer, P. Fontanillas, R. M. Fraser, D. F. Freitag, D. Gurdasani, K. Heikkila, E. Hypponen, A. Isaacs, A. U. Jackson, A. Johansson, T. Johnson, M. Kaakinen, J. Kettunen, M. E. Kleber, X. Li, J. Luan, L. P. Lyytikainen, P. K. E. Magnusson, M. Mangino, E. Mihailov, M. E. Montasser, M. Muller-Nurasyid, I. M. Nolte, J. R. O'Connell, C. D. Palmer, M. Perola, A. K. Petersen, S. Sanna, R. Saxena, S. K. Service, S. Shah, D. Shungin, C. Sidore, C. Song, R. J. Strawbridge, I. Surakka, T. Tanaka, T. M. Teslovich, G. Thorleifsson, E. G. Van den Herik, B. F. Voight, K. A. Volcik, L. L. Waite, A. Wong, Y. Wu, W. Zhang, D. Absher, G. Asiki, I. Barroso, L. F. Been, J. L. Bolton, L. L. Bonnycastle, P. Brambilla, M. S. Burnett, G. Cesana, M. Dimitriou, A. S. F. Doney, A. Doring, P. Elliott, S. E. Epstein, G. Ingi Eyjolfsson, B. Gigante, M. O. Goodarzi, H. Grallert, M. L. Gravito, C. J. Groves, G. Hallmans, A. L. Hartikainen, C. Hayward, D. Hernandez, A. A. Hicks, H. Holm, Y. J. Hung, T. Illig, M. R. Jones, P. Kaleebu, J. J. P. Kastelein, K. T. Khaw, E. Kim, N. Klopp, P. Komulainen, M. Kumari, C. Langenberg, T. Lehtimaki, S. Y. Lin, J. Lindstrom, R. J. F. Loos, F. Mach, W. L. McArdle, C. Meisinger, B. D. Mitchell, G. Muller, R. Nagaraja, N. Narisu, T. V. M. Nieminen, R. N. Nsubuga, I. Olafsson, K. K. Ong, A. Palotie, T. Papamarkou, C. Pomilla, A. Pouta, D. J. Rader, M. P. Reilly, P. M. Ridker, F. Rivadeneira, I. Rudan, A. Ruokonen, N. Samani, H. Scharnagl, J. Seeley, K. Silander, A. Stancakova, K. Stirrups, A. J. Swift, L. Tiret, A. G. Uitterlinden, L. J. van Pelt, S. Vedantam, N. Wainwright, C. Wijmenga, S. H. Wild, G. Willemsen, T. Wilsgaard, J. F. Wilson, E. H. Young, J. H. Zhao, L. S. Adair, D. Arveiler, T. L. Assimes, S. Bandinelli, F. Bennett, M. Bochud, B. O. Boehm, D. I. Boomsma, I. B. Borecki, S. R. Bornstein, P. Bovet, M. Burnier, H. Campbell, A. Chakravarti, J. C. Chambers, Y. I. Chen, F. S. Collins, R. S. Cooper, J. Danesh, G. Dedoussis, U. de Faire, A. B. Feranil, J. Ferrieres, L. Ferrucci, N. B. Freimer, C. Gieger, L. C. Groop, V. Gudnason, U. Gyllensten, A. Hamsten, T. B. Harris, A. Hingorani, J. N. Hirschhorn, A. Hofman, G. K. Hovingh, C. A. Hsiung, S. E. Humphries, S. C. Hunt, K. Hveem, C. Iribarren, M. R. Jarvelin, A. Jula, M. Kahonen, J. Kaprio, A. Kesaniemi, M. Kivimaki, J. S. Kooner, P. J. Koudstaal, R. M. Krauss, D. Kuh, J. Kuusisto, K. O. Kyvik, M. Laakso, T. A. Lakka, L. Lind, C. M. Lindgren, N. G. Martin, W. Marz, M. I. McCarthy, C. A. McKenzie, P. Meneton, A. Metspalu, L. Moilanen, A. D. Morris, P. B. Munroe, I. Njolstad, N. L. Pedersen, C. Power, P. P. Pramstaller, J. F. Price, B. M. Psaty, T. Quertermous, R. Rauramaa, D. Saleheen, V. Salomaa, D. K. Sanghera, J. Saramies, P. E. H. Schwarz, W. H. Sheu, A. R. Shuldiner, A. Siegbahn, T. D. Spector, K. Stefansson, D. P. Strachan, B. O. Tayo, E. Tremoli, J. Tuomilehto, M. Uusitupa, C. M. van Duijn, P. Vollenweider, L. Wallentin, N. J. Wareham, J. B. Whitfield, B. H. R. Wolffenbuttel, J. M. Ordovas, E. Boerwinkle, C. N. A. Palmer, U. Thorsteinsdottir, D. I. Chasman, J. I. Rotter, P. W. Franks, S. Ripatti, L. A. Cupples, M. S. Sandhu, S. S. Rich, M. Boehnke, P. Deloukas, S. Kathiresan, K. L. Mohlke, E. Ingelsson, G. R. Abecasis and C. Global Lipids Genetics, Discovery and refinement of loci associated with lipid levels, Nat. Genet., 2013, 45, 1274–1283 CrossRef CAS PubMed.
- P. J. Barter, M. Caulfield, M. Eriksson, S. M. Grundy, J. J. Kastelein, M. Komajda, J. Lopez-Sendon, L. Mosca, J. C. Tardif, D. D. Waters, C. L. Shear, J. H. Revkin, K. A. Buhr, M. R. Fisher, A. R. Tall, B. Brewer and I. Investigators, Effects of torcetrapib in patients at high risk for coronary events, N. Engl. J. Med., 2007, 357, 2109–2122 CrossRef CAS PubMed.
- G. G. Schwartz, A. G. Olsson, M. Abt, C. M. Ballantyne, P. J. Barter, J. Brumm, B. R. Chaitman, I. M. Holme, D. Kallend, L. A. Leiter, E. Leitersdorf, J. J. McMurray, H. Mundl, S. J. Nicholls, P. K. Shah, J. C. Tardif, R. S. Wright and O. I. Dal, Effects of dalcetrapib in patients with a recent acute coronary syndrome, N. Engl. J. Med., 2012, 367, 2089–2099 CrossRef CAS PubMed.
- A. M. Lincoff, S. J. Nicholls, J. S. Riesmeyer, P. J. Barter, H. B. Brewer, K. A. A. Fox, C. M. Gibson, C. Granger, V. Menon, G. Montalescot, D. Rader, A. R. Tall, E. McErlean, K. Wolski, G. Ruotolo, B. Vangerow, G. Weerakkody, S. G. Goodman, D. Conde, D. K. McGuire, J. C. Nicolau, J. L. Leiva-Pons, Y. Pesant, W. Li, D. Kandath, S. Kouz, N. Tahirkheli, D. Mason, S. E. Nissen and A. Investigators, Evacetrapib and Cardiovascular Outcomes in High-Risk Vascular Disease, N. Engl. J. Med., 2017, 376, 1933–1942 CrossRef PubMed.
- L. Bowman, J. C. Hopewell, F. Chen, K. Wallendszus, W. Stevens, R. Collins, S. D. Wiviott, C. P. Cannon, E. Braunwald, E. Sammons, M. J. Landray and HPS3/TIMI55–REVEAL Collaborative Group, Effects of Anacetrapib in Patients with Atherosclerotic Vascular Disease., N. Engl. J. Med., 2017, 377, 1217–1227 CrossRef PubMed.
- W. E. Boden, J. L. Probstfield, T. Anderson, B. R. Chaitman, P. Desvignes-Nickens, K. Koprowicz, R. McBride, K. Teo, W. Weintraub and AIM-HIGH Investigators, Niacin in patients with low HDL cholesterol levels receiving intensive statin therapy., N. Engl. J. Med., 2011, 365, 2255–2267 CrossRef PubMed.
- H. T. C. Group, M. J. Landray, R. Haynes, J. C. Hopewell, S. Parish, T. Aung, J. Tomson, K. Wallendszus, M. Craig, L. Jiang, R. Collins and J. Armitage, Effects of extended-release niacin with laropiprant
in high-risk patients, N. Engl. J. Med., 2014, 371, 203–212 CrossRef PubMed.
- C. M. Madsen, A. Varbo and B. G. Nordestgaard, Extreme high high-density lipoprotein cholesterol is paradoxically associated with high mortality in men and women: two prospective cohort studies, Eur. Heart J., 2017, 38, 2478–2486 CrossRef CAS PubMed.
- S. Kajani, S. Curley and F. C. McGillicuddy, Unravelling HDL-Looking beyond the Cholesterol Surface to the Quality Within, Int. J. Mol. Sci., 2018, 19, 1971 CrossRef.
- D. J. Rader and G. K. Hovingh, HDL and cardiovascular disease, Lancet, 2014, 384, 618–625 CrossRef CAS.
- A. V. Khera, M. Cuchel, M. de la Llera-Moya, A. Rodrigues, M. F. Burke, K. Jafri, B. C. French, J. A. Phillips, M. L. Mucksavage, R. L. Wilensky, E. R. Mohler, G. H. Rothblat and D. J. Rader, Cholesterol efflux capacity, high-density lipoprotein function, and atherosclerosis, N. Engl. J. Med., 2011, 364, 127–135 CrossRef CAS PubMed.
- A. Rohatgi, A. Khera, J. D. Berry, E. G. Givens, C. R. Ayers, K. E. Wedin, I. J. Neeland, I. S. Yuhanna, D. R. Rader, J. A. de Lemos and P. W. Shaul, HDL cholesterol efflux capacity and incident cardiovascular events, N. Engl. J. Med., 2014, 371, 2383–2393 CrossRef CAS PubMed.
- D. Saleheen, R. Scott, S. Javad, W. Zhao, A. Rodrigues, A. Picataggi, D. Lukmanova, M. L. Mucksavage, R. Luben, J. Billheimer, J. J. Kastelein, S. M. Boekholdt, K. T. Khaw, N. Wareham and D. J. Rader, Association of HDL cholesterol efflux capacity with incident coronary heart disease events: a prospective case-control study, Lancet Diabetes Endocrinol., 2015, 3, 507–513 CrossRef CAS PubMed.
- A. Ritsch, H. Scharnagl and W. Marz, HDL cholesterol efflux capacity and cardiovascular events, N. Engl. J. Med., 2015, 372, 1870–1871 Search PubMed.
- X. M. Li, W. H. Tang, M. K. Mosior, Y. Huang, Y. Wu, W. Matter, V. Gao, D. Schmitt, J. A. Didonato, E. A. Fisher, J. D. Smith and S. L. Hazen, Paradoxical association of enhanced cholesterol efflux with increased incident cardiovascular risks, Arterioscler., Thromb., Vasc. Biol., 2013, 33, 1696–1705 CrossRef CAS.
- E. J. Cohn, F. R. N. Gurd, D. M. Surgenor, B. A. Barnes, R. K. Brown, G. Derouaux, J. M. Gillespie, F. W. Kahnt, W. F. Lever, C. H. Liu, D. Mittelman, R. F. Mouton, K. Schmid and E. Uroma, A System for the Separation of the Components of Human Blood: Quantitative Procedures for the Separation of the Protein Components of Human Plasma, J. Am. Chem. Soc., 1950, 72(1)), 465–474, DOI:10.1021/ja01157a122.
- D. P. Barr, E. M. Russ and H. A. Eder, Protein-lipid relationships in human plasma. II. In atherosclerosis and related conditions, Am. J. Med., 1951, 11, 480–493 CrossRef CAS.
- K. Li, D. K. Wong, F. S. Luk, R. Y. Kim and R. L. Raffai, Isolation of Plasma Lipoproteins as a Source of Extracellular RNA, Methods Mol. Biol., 2018, 1740, 139–153 CrossRef CAS.
- N. L. Price, N. Rotllan, X. Zhang, A. Canfran-Duque, T. Nottoli, Y. Suarez and C. Fernandez-Hernando, Specific Disruption of Abca1 Targeting Largely Mimics the Effects of miR-33 Knockout on Macrophage Cholesterol Efflux and Atherosclerotic Plaque Development, Circ. Res., 2019, 124, 874–880 CrossRef CAS.
- P. G. Lerch, V. Fortsch, G. Hodler and R. Bolli, Production and characterization of a reconstituted high density lipoprotein for therapeutic applications, Vox Sang., 1996, 71, 155–164 CrossRef CAS.
- R. Easton, A. Gille, D. D'Andrea, R. Davis, S. D. Wright and C. Shear, A multiple ascending dose study of CSL112, an infused formulation of ApoA-I, J. Clin. Pharmacol., 2014, 54, 301–310 CrossRef CAS PubMed.
- M. H. Caparon, K. J. Rust, A. K. Hunter, J. K. McLaughlin, K. E. Thomas, J. T. Herberg, R. E. Shell, P. B. Lanter, B. F. Bishop, R. L. Dufield, X. Wang and S. V. Ho, Integrated solution to purification challenges in the manufacture of a soluble recombinant protein in E. coli, Biotechnol. Bioeng., 2010, 105, 239–249 CrossRef CAS PubMed.
- V. Narasimhan Janakiraman, A. Noubhani, K. Venkataraman, M. Vijayalakshmi and X. Santarelli, High yield of recombinant human Apolipoprotein A-I expressed in Pichia pastoris by using mixed-mode chromatography, Biotechnol. J., 2016, 11, 117–126 CrossRef CAS PubMed.
- C. Tardy, M. Goffinet, N. Boubekeur, G. Cholez, R. Ackermann, G. Sy, C. Keyserling, N. Lalwani, J. F. Paolini, J. L. Dasseux, R. Barbaras and R. Baron, HDL and CER-001 Inverse-Dose Dependent Inhibition of Atherosclerotic Plaque Formation in apoE-/- Mice: Evidence of ABCA1 Down-Regulation, PLoS One, 2015, 10, e0137584 CrossRef.
- J. A. Cappuccio, A. K. Hinz, E. A. Kuhn, J. E. Fletcher, E. S. Arroyo, P. T. Henderson, C. D. Blanchette, V. L. Walsworth, M. H. Corzett, R. J. Law, J. B. Pesavento, B. W. Segelke, T. A. Sulchek, B. A. Chromy, F. Katzen, T. Peterson, G. Bench, W. Kudlicki, P. D. Hoeprich Jr. and M. A. Coleman, Cell-free expression for nanolipoprotein particles: building a high-throughput membrane protein solubility platform, Methods Mol. Biol., 2009, 498, 273–296 CrossRef CAS PubMed.
- D. G. Kallend, J. A. Reijers, S. E. Bellibas, A. Bobillier, H. Kempen, J. Burggraaf, M. Moerland and P. L. Wijngaard, A single infusion of MDCO-216 (ApoA-1 Milano/POPC) increases ABCA1-mediated cholesterol efflux and pre-beta 1 HDL in healthy volunteers and patients with stable coronary artery disease, Eur. Heart J.: Cardiovasc. Pharmacother., 2016, 2, 23–29 CAS.
- J. C. Tardif, C. M. Ballantyne, P. Barter, J. L. Dasseux, Z. A. Fayad, M. C. Guertin, J. J. Kastelein, C. Keyserling, H. Klepp, W. Koenig, P. L. L’Allier, J. Lesperance, T. F. Luscher, J. F. Paolini, A. Tawakol, D. D. Waters and CHI-SQUARE Investigators, Effects of the high-density lipoprotein mimetic agent CER-001 on coronary atherosclerosis in patients with acute coronary syndromes: a randomized trial, Eur. Heart J., 2014, 35, 3277–3286 CrossRef CAS PubMed.
- S. Stukas, J. Robert, M. Lee, I. Kulic, M. Carr, K. Tourigny, J. Fan, D. Namjoshi, K. Lemke, N. DeValle, J. Chan, T. Wilson, A. Wilkinson, R. Chapanian, J. N. Kizhakkedathu, J. R. Cirrito, M. N. Oda and C. L. Wellington, Intravenously injected human apolipoprotein A-I rapidly enters the central nervous system via the choroid plexus, J. Am. Heart Assoc., 2014, 3, e001156 Search PubMed.
- M. Fukuda, M. Nakano, M. Miyazaki and T. Handa, Thermodynamic and kinetic stability of discoidal high-density lipoprotein formation from phosphatidylcholine/apolipoprotein A-I mixture, J. Phys. Chem. B, 2010, 114, 8228–8234 CrossRef CAS.
- A. Scanu, Binding of human serum high density lipoprotein apoprotein with aqueous dispersions of phospholipids, J. Biol. Chem., 1967, 242, 711–719 CrossRef CAS.
- R. C. Pittman, C. K. Glass, D. Atkinson and D. M. Small, Synthetic high density lipoprotein particles. Application to studies of the apoprotein specificity for selective uptake of cholesterol esters, J. Biol. Chem., 1987, 262, 2435–2442 CrossRef CAS.
- C. E. Matz and A. Jonas, Micellar complexes of human apolipoprotein A-I with phosphatidylcholines and cholesterol prepared from cholate-lipid dispersions, J. Biol. Chem., 1982, 257, 4535–4540 CrossRef CAS.
- A. Schwendeman, D. O. Sviridov, W. Yuan, Y. Guo, E. E. Morin, Y. Yuan, J. Stonik, L. Freeman, A. Ossoli, S. Thacker, S. Killion, M. Pryor, Y. E. Chen, S. Turner and A. T. Remaley, The effect of phospholipid composition of reconstituted HDL on its cholesterol efflux and anti-inflammatory properties, J. Lipid Res., 2015, 56, 1727–1737 CrossRef CAS PubMed.
- Y. Kim, F. Fay, D. P. Cormode, B. L. Sanchez-Gaytan, J. Tang, E. J. Hennessy, M. Ma, K. Moore, O. C. Farokhzad, E. A. Fisher, W. J. Mulder, R. Langer and Z. A. Fayad, Single step reconstitution of multifunctional high-density lipoprotein-derived nanomaterials using microfluidics, ACS Nano, 2013, 7, 9975–9983 CrossRef CAS PubMed.
- Y. J. Sei, J. Ahn, T. Kim, E. Shin, A. J. Santiago-Lopez, S. S. Jang, N. L. Jeon, Y. C. Jang and Y. Kim, Detecting the functional complexities between high-density lipoprotein mimetics, Biomaterials, 2018, 170, 58–69 CrossRef CAS PubMed.
- J. H. Wade, J. D. Jones, I. L. Lenov, C. M. Riordan, S. G. Sligar and R. C. Bailey, Microfluidic platform for efficient Nanodisc assembly, membrane protein incorporation, and purification, Lab Chip, 2017, 17, 2951–2959 RSC.
- H. J. Kempen, D. B. Schranz, B. F. Asztalos, J. Otvos, E. Jeyarajah, D. Drazul-Schrader, H. L. Collins, S. J. Adelman and P. L. Wijngaard, Incubation of MDCO-216 (ApoA-IMilano/POPC) with Human Serum Potentiates ABCA1-Mediated Cholesterol Efflux Capacity, Generates New Prebeta-1 HDL, and Causes an Increase in HDL Size, J. Lipids, 2014, 2014, 923903 Search PubMed.
- R. Al-Kassas, M. Bansal and J. Shaw, Nanosizing techniques for improving bioavailability of drugs, J. Controlled Release, 2017, 260, 202–212 CrossRef CAS PubMed.
- T. Binderup, R. Duivenvoorden, F. Fay, M. M. T. van Leent, J. Malkus, S. Baxter, S. Ishino, Y. Zhao, B. Sanchez-Gaytan, A. J. P. Teunissen, Y. C. A. Frederico, J. Tang, G. Carlucci, S. Lyashchenko, C. Calcagno, N. Karakatsanis, G. Soultanidis, M. L. Senders, P. M. Robson, V. Mani, S. Ramachandran, M. E. Lobatto, B. A. Hutten, J. F. Granada, T. Reiner, F. K. Swirski, M. Nahrendorf, A. Kjaer, E. A. Fisher, Z. A. Fayad, C. Perez-Medina and W. J. M. Mulder, Imaging-assisted nanoimmunotherapy for atherosclerosis in multiple species, Sci. Transl. Med., 2019, 11, eaaw7736 CrossRef PubMed.
- S. Inagaki and R. Ghirlando, Nanodisc characterization by analytical ultracentrifugation, Nanotechnol. Rev., 2017, 6(1), 3–14, DOI:10.1515/ntrev-2016-0082.
- S. Inagaki, R. Ghirlando and R. Grisshammer, Biophysical characterization of membrane proteins in nanodiscs, Methods, 2013, 59, 287–300 CrossRef CAS PubMed.
- L. A. Fuentes, W. H. J. Beck, M. Tsujita and P. M. M. Weers, Charged Residues in the C-Terminal Domain of Apolipoprotein A-I Modulate Oligomerization, Biochemistry, 2018, 57, 2200–2210 CrossRef CAS PubMed.
- H. Patel, B. Ding, K. Ernst, L. Shen, W. Yuan, J. Tang, L. R. Drake, J. Kang, Y. Li, Z. Chen and A. Schwendeman, Characterization of apolipoprotein A-I peptide phospholipid interaction and its effect on HDL nanodisc assembly, Int. J. Nanomed., 2019, 14, 3069–3086 CrossRef CAS PubMed.
- X. M. Du, M. J. Kim, L. Hou, W. Le Goff, M. J. Chapman, M. Van Eck, L. K. Curtiss, J. R. Burnett, S. P. Cartland, C. M. Quinn, M. Kockx, A. Kontush, K. A. Rye, L. Kritharides and W. Jessup, HDL particle size is a critical determinant of ABCA1-mediated macrophage cellular cholesterol export, Circ. Res., 2015, 116, 1133–1142 CrossRef CAS PubMed.
- L. A. Freeman, Native-native 2D gel electrophoresis for HDL subpopulation analysis, Methods Mol. Biol., 2013, 1027, 353–367 CrossRef CAS PubMed.
- D. L. Sparks and M. C. Phillips, Quantitative measurement of lipoprotein surface charge by agarose gel electrophoresis, J. Lipid Res., 1992, 33, 123–130 CrossRef CAS.
- S. Inagaki and R. Ghirlando, Nanodisc characterization by analytical ultracentrifugation, Nanotechnol. Rev., 2017, 6(1), 3–14, DOI:10.1515/ntrev-2016-0082.
- M. de Messieres, A. Ng, C. J. Duarte, A. T. Remaley and J. C. Lee, Single-Particle Tracking of Human Lipoproteins, Anal. Chem., 2016, 88, 596–599 CrossRef CAS.
- C. Gan, M. Ao, Z. Liu and Y. Chen, Imaging and force measurement of LDL and HDL by AFM in air and liquid, FEBS Open Bio, 2015, 5, 276–282 CrossRef CAS PubMed.
- C. Gan, Z. Wang and Y. Chen, In situ AFM imaging of apolipoprotein A-I directly derived from plasma HDL, Atherosclerosis, 2017, 259, 5–11 CrossRef CAS PubMed.
- L. Zhang, J. Song, G. Cavigiolio, B. Y. Ishida, S. Zhang, J. P. Kane, K. H. Weisgraber, M. N. Oda, K. A. Rye, H. J. Pownall and G. Ren, Morphology and structure of lipoproteins revealed by an optimized negative-staining protocol of electron microscopy, J. Lipid Res., 2011, 52, 175–184 CrossRef CAS PubMed.
- S. C. Murray, B. K. Gillard, S. J. Ludtke and H. J. Pownall, Direct Measurement of the Structure of Reconstituted High-Density Lipoproteins by Cryo-EM, Biophys. J., 2016, 110, 810–816 CrossRef CAS PubMed.
- I. G. Denisov, Y. V. Grinkova, A. A. Lazarides and S. G. Sligar, Directed self-assembly of monodisperse phospholipid bilayer Nanodiscs with controlled size, J. Am. Chem. Soc., 2004, 126, 3477–3487 CrossRef CAS PubMed.
- V. Gogonea, G. S. Gerstenecker, Z. Wu, X. Lee, C. Topbas, M. A. Wagner, T. C. Tallant, J. D. Smith, P. Callow, V. Pipich, H. Malet, G. Schoehn, J. A. DiDonato and S. L. Hazen, The low-resolution structure of nHDL reconstituted with DMPC with and without cholesterol reveals a mechanism for particle expansion, J. Lipid Res., 2013, 54, 966–983 CrossRef CAS PubMed.
- F. Hagn, M. Etzkorn, T. Raschle and G. Wagner, Optimized phospholipid bilayer nanodiscs facilitate high-resolution structure determination of membrane proteins, J. Am. Chem. Soc., 2013, 135, 1919–1925 CrossRef CAS PubMed.
- S. Bibow, Y. Polyhach, C. Eichmann, C. N. Chi, J. Kowal, S. Albiez, R. A. McLeod, H. Stahlberg, G. Jeschke, P. Guntert and R. Riek, Solution structure of discoidal high-density lipoprotein particles with a shortened apolipoprotein A-I, Nat. Struct. Mol. Biol., 2017, 24, 187–193 CrossRef CAS PubMed.
- G. Cavigiolio, B. Shao, E. G. Geier, G. Ren, J. W. Heinecke and M. N. Oda, The interplay between size, morphology, stability, and functionality of high-density lipoprotein subclasses, Biochemistry, 2008, 47, 4770–4779 CrossRef CAS PubMed.
- N. N. Lyssenko, M. Nickel, C. Tang and M. C. Phillips, Factors controlling nascent high-density lipoprotein particle heterogeneity: ATP-binding cassette transporter A1 activity and cell lipid and apolipoprotein AI availability, FASEB J., 2013, 27, 2880–2892 CrossRef CAS PubMed.
- R. D. Giudice, O. Nilsson, J. Domingo-Espin and J. O. Lagerstedt, Synchrotron radiation circular dichroism spectroscopy reveals structural divergences in HDL-bound apoA-I variants, Sci. Rep., 2017, 7, 13540 CrossRef PubMed.
- J. H. Wald, E. Coormaghtigh, J. De Meutter, J. M. Ruysschaert and A. Jonas, Investigation of the lipid domains and apolipoprotein orientation in reconstituted high density lipoproteins by fluorescence and IR methods, J. Biol. Chem., 1990, 265, 20044–20050 CrossRef CAS.
- J. Jiang, G. Oberdörster and P. Biswas, Characterization of size, surface charge, and agglomeration state of nanoparticle dispersions for toxicological studies, J. Nanopart. Res., 2009, 11, 77, DOI:10.1007/s11051-008-9446-4.
- G. R. Bassett, B. K. Gillard and H. J. Pownall, Cholesterol determines and limits rHDL formation from human plasma apolipoprotein A-II and phospholipid membranes, Biochemistry, 2012, 51, 8627–8635 CrossRef CAS PubMed.
- X. Deng, J. Morris, J. Dressmen, M. R. Tubb, P. Tso, W. G. Jerome, W. S. Davidson and T. B. Thompson, The structure of dimeric apolipoprotein A-IV and its mechanism of self-association, Structure, 2012, 20, 767–779 CrossRef CAS PubMed.
- M. Huang, M. Hu, Q. Song, H. Song, J. Huang, X. Gu, X. Wang, J. Chen, T. Kang, X. Feng, D. Jiang, G. Zheng, H. Chen and X. Gao, GM1-Modified Lipoprotein-like Nanoparticle: Multifunctional Nanoplatform for the Combination Therapy of Alzheimer's Disease, ACS Nano, 2015, 9, 10801–10816 CrossRef CAS.
- S. Fernandez-de-Retana, M. Cano-Sarabia, P. Marazuela, J. L. Sanchez-Quesada, A. Garcia-Leon, A. Montanola, J. Montaner, D. Maspoch and M. Hernandez-Guillamon, Characterization of ApoJ-reconstituted high-density lipoprotein (rHDL) nanodisc for the potential treatment of cerebral beta-amyloidosis, Sci. Rep., 2017, 7, 14637 CrossRef.
- H. Takase, M. Tanaka, Y. Nakamura, S. Y. Morita, T. Yamada and T. Mukai, Effects of lipid composition on the structural properties of human serum amyloid A in reconstituted high-density lipoprotein particles, Chem. Phys. Lipids, 2019, 221, 8–14 CrossRef CAS.
- C. Eichmann, P. Kumari and R. Riek, High-density lipoprotein-like particle formation of Synuclein variants, FEBS Lett., 2017, 591, 304–311 CrossRef CAS PubMed.
- K. A. Rye and P. J. Barter, Regulation of high-density lipoprotein metabolism, Circ. Res., 2014, 114, 143–156 CrossRef CAS PubMed.
- V. Gogonea, Structural Insights into High Density Lipoprotein: Old Models and New Facts, Front. Pharmacol., 2015, 6, 318 Search PubMed.
- J. T. Melchior, R. G. Walker, A. L. Cooke, J. Morris, M. Castleberry, T. B. Thompson, M. K. Jones, H. D. Song, K. A. Rye, M. N. Oda, M. G. Sorci-Thomas, M. J. Thomas, J. W. Heinecke, X. Mei, D. Atkinson, J. P. Segrest, S. Lund-Katz, M. C. Phillips and W. S. Davidson, A consensus model of human apolipoprotein A-I in its monomeric and lipid-free state, Nat. Struct. Mol. Biol., 2017, 24, 1093–1099 CrossRef CAS PubMed.
- K. A. McGuire, W. S. Davidson and A. Jonas, High yield overexpression and characterization of human recombinant proapolipoprotein A-I, J. Lipid Res., 1996, 37, 1519–1528 CrossRef CAS.
- M. Eriksson, L. A. Carlson, T. A. Miettinen and B. Angelin, Stimulation of fecal steroid excretion after infusion of recombinant proapolipoprotein A-I. Potential reverse cholesterol transport in humans, Circulation, 1999, 100, 594–598 CrossRef CAS PubMed.
- J. H. Graversen, J. M. Laurberg, M. H. Andersen, E. Falk, J. Nieland, J. Christensen, M. Etzerodt, H. C. Thogersen and S. K. Moestrup, Trimerization of apolipoprotein A-I retards plasma clearance and preserves antiatherosclerotic properties, J. Cardiovasc. Pharmacol., 2008, 51, 170–177 CrossRef CAS PubMed.
- G. M. Anantharamaiah, J. L. Jones, C. G. Brouillette, C. F. Schmidt, B. H. Chung, T. A. Hughes, A. S. Bhown and J. P. Segrest, Studies of synthetic peptide analogs of the amphipathic helix. Structure of complexes with dimyristoyl phosphatidylcholine, J. Biol. Chem., 1985, 260, 10248–10255 CrossRef CAS.
- M. Navab, G. M. Anantharamaiah, S. T. Reddy, S. Hama, G. Hough, V. R. Grijalva, N. Yu, B. J. Ansell, G. Datta, D. W. Garber and A. M. Fogelman, Apolipoprotein A-I mimetic peptides, Arterioscler., Thromb., Vasc. Biol., 2005, 25, 1325–1331 CrossRef CAS PubMed.
- J. A. Gazzara, M. C. Phillips, S. Lund-Katz, M. N. Palgunachari, J. P. Segrest, G. M. Anantharamaiah and J. W. Snow, Interaction of class A amphipathic helical peptides with phospholipid unilamellar vesicles, J. Lipid Res., 1997, 38, 2134–2146 CrossRef CAS.
- W. D'Souza, J. A. Stonik, A. Murphy, S. J. Demosky, A. A. Sethi, X. L. Moore, J. Chin-Dusting, A. T. Remaley and D. Sviridov, Structure/function relationships of apolipoprotein a-I mimetic peptides: implications for antiatherogenic activities of high-density lipoprotein, Circ. Res., 2010, 107, 217–227 CrossRef PubMed.
- A. Iwata, S. Miura, B. Zhang, S. Imaizumi, Y. Uehara, M. Shiomi and K. Saku, Antiatherogenic effects of newly developed apolipoprotein A-I mimetic peptide/phospholipid complexes against aortic plaque burden in Watanabe-heritable hyperlipidemic rabbits, Atherosclerosis, 2011, 218, 300–307 CrossRef CAS.
- D. O. Sviridov, I. Z. Ikpot, J. Stonik, S. K. Drake, M. Amar, D. O. Osei-Hwedieh, G. Piszczek, S. Turner and A. T. Remaley, Helix stabilization of amphipathic peptides by hydrocarbon stapling increases cholesterol efflux by the ABCA1 transporter, Biochem. Biophys. Res. Commun., 2011, 410, 446–451 CrossRef CAS PubMed.
- Y. Uehara, S. Ando, E. Yahiro, K. Oniki, M. Ayaori, S. Abe, E. Kawachi, B. Zhang, S. Shioi, H. Tanigawa, S. Imaizumi, S. Miura and K. Saku, FAMP, a novel apoA-I mimetic peptide, suppresses aortic plaque formation through promotion of biological HDL function in ApoE-deficient mice, J. Am. Heart Assoc., 2013, 2, e000048 Search PubMed.
- R. M. Islam, M. Pourmousa, D. Sviridov, S. M. Gordon, E. B. Neufeld, L. A. Freeman, B. S. Perrin Jr., R. W. Pastor and A. T. Remaley, Structural properties of apolipoprotein A-I mimetic peptides that promote ABCA1-dependent cholesterol efflux, Sci. Rep., 2018, 8, 2956 CrossRef PubMed.
- J. B. Massey and H. J. Pownall, Cholesterol is a determinant of the structures of discoidal high density lipoproteins formed by the solubilization of phospholipid membranes by apolipoprotein A-I, Biochim. Biophys. Acta, 2008, 1781, 245–253 CrossRef CAS PubMed.
- S. A. Sanchez, M. A. Tricerri and E. Gratton, Interaction of high density lipoprotein particles with membranes containing cholesterol, J. Lipid Res., 2007, 48, 1689–1700 CrossRef CAS PubMed.
- M. Miyazaki, Y. Tajima, Y. Ishihama, T. Handa and M. Nakano, Effect of phospholipid composition on discoidal HDL formation, Biochim. Biophys. Acta, 2013, 1828, 1340–1346 CrossRef CAS PubMed.
- P. Ramos-Cabrer, F. Fay, B. L. Sanchez-Gaytan, J. Tang, J. Castillo, Z. A. Fayad and W. J. Mulder, Conformational Changes in High-Density Lipoprotein Nanoparticles Induced by High Payloads of Paramagnetic Lipids, ACS Omega, 2016, 1, 470–475 CrossRef CAS PubMed.
- P. Marmillot, S. Patel and M. R. Lakshman, Reverse cholesterol transport is regulated by varying fatty acyl chain saturation and sphingomyelin content in reconstituted high-density lipoproteins, Metabolism, 2007, 56, 251–259 CrossRef CAS.
- J. Tang, S. Baxter, A. Menon, A. Alaarg, B. L. Sanchez-Gaytan, F. Fay, Y. Zhao, M. Ouimet, M. S. Braza, V. A. Longo, D. Abdel-Atti, R. Duivenvoorden, C. Calcagno, G. Storm, S. Tsimikas, K. J. Moore, F. K. Swirski, M. Nahrendorf, E. A. Fisher, C. Perez-Medina, Z. A. Fayad, T. Reiner and W. J. Mulder, Immune cell screening of a nanoparticle library improves atherosclerosis therapy, Proc. Natl. Acad. Sci. U. S. A., 2016, 113, E6731–E6740 CrossRef CAS PubMed.
- A. J. Murphy, S. Funt, D. Gorman, A. R. Tall and N. Wang, Pegylation of high-density lipoprotein decreases plasma clearance and enhances antiatherogenic activity, Circ. Res., 2013, 113, e1–e9 CrossRef CAS PubMed.
- S. F. Gilmore, C. D. Blanchette, T. M. Scharadin, G. L. Hura, A. Rasley, M. Corzett, C. X. Pan, N. O. Fischer and P. T. Henderson, Lipid Cross-Linking of Nanolipoprotein Particles Substantially Enhances Serum Stability and Cellular Uptake, ACS Appl. Mater. Interfaces, 2016, 8, 20549–20557 CrossRef CAS PubMed.
- Y. Zhao, C. Jiang, J. He, Q. Guo, J. Lu, Y. Yang, W. Zhang and J. Liu, Multifunctional Dextran Sulfate-Coated Reconstituted High Density Lipoproteins Target Macrophages and Promote Beneficial Antiatherosclerotic Mechanisms, Bioconjugate Chem., 2017, 28, 438–448 CrossRef CAS PubMed.
- T. J. Beldman, M. L. Senders, A. Alaarg, C. Perez-Medina, J. Tang, Y. Zhao, F. Fay, J. Deichmoller, B. Born, E. Desclos, N. N. van der Wel, R. A. Hoebe, F. Kohen, E. Kartvelishvily, M. Neeman, T. Reiner, C. Calcagno, Z. A. Fayad, M. P. J. de Winther, E. Lutgens, W. J. M. Mulder and E. Kluza, Hyaluronan Nanoparticles Selectively Target Plaque-Associated Macrophages and Improve Plaque Stability in Atherosclerosis, ACS Nano, 2017, 11, 5785–5799 CrossRef CAS.
- T. J. Beldman, T. S. Malinova, E. Desclos, A. E. Grootemaat, A. L. S. Misiak, S. van der Velden, C. van Roomen, L. Beckers, H. A. van Veen, P. M. Krawczyk, R. A. Hoebe, J. C. Sluimer, A. E. Neele, M. P. J. de Winther, N. N. van der Wel, E. Lutgens, W. J. M. Mulder, S. Huveneers and E. Kluza, Nanoparticle-Aided Characterization of Arterial Endothelial Architecture during Atherosclerosis Progression and Metabolic Therapy, ACS Nano, 2019, 13, 13759–13774 CrossRef CAS PubMed.
- B. L. Sanchez-Gaytan, F. Fay, M. E. Lobatto, J. Tang, M. Ouimet, Y. Kim, S. E. van der Staay, S. M. van Rijs, B. Priem, L. Zhang, E. A. Fisher, K. J. Moore, R. Langer, Z. A. Fayad and W. J. Mulder, HDL-mimetic PLGA nanoparticle to target atherosclerosis plaque macrophages, Bioconjugate Chem., 2015, 26, 443–451 CrossRef CAS PubMed.
- C. Perez-Medina, J. Tang, D. Abdel-Atti, B. Hogstad, M. Merad, E. A. Fisher, Z. A. Fayad, J. S. Lewis, W. J. Mulder and T. Reiner, PET Imaging of Tumor-Associated Macrophages with 89Zr-Labeled High-Density Lipoprotein Nanoparticles, J. Nucl. Med., 2015, 56, 1272–1277 CrossRef CAS PubMed.
- R. Wang, C. Zhang, J. Li, J. Huang, Y. Opoku-Damoah, B. Sun, J. Zhou, L. Di and Y. Ding, Laser-triggered polymeric lipoproteins for precision tumor penetrating theranostics, Biomaterials, 2019, 221, 119413 CrossRef CAS PubMed.
- H. Zhang, Y. Zhao, M. Yu, Z. Zhao, P. Liu, H. Cheng, Y. Ji, Y. Jin, B. Sun, J. Zhou and Y. Ding, Reassembly of native components with donepezil to execute dual-missions in Alzheimer's disease therapy, J. Controlled Release, 2019, 296, 14–28 CrossRef CAS PubMed.
- R. Duivenvoorden, J. Tang, D. P. Cormode, A. J. Mieszawska, D. Izquierdo-Garcia, C. Ozcan, M. J. Otten, N. Zaidi, M. E. Lobatto, S. M. van Rijs, B. Priem, E. L. Kuan, C. Martel, B. Hewing, H. Sager, M. Nahrendorf, G. J. Randolph, E. S. Stroes, V. Fuster, E. A. Fisher, Z. A. Fayad and W. J. Mulder, A statin-loaded reconstituted high-density lipoprotein nanoparticle inhibits atherosclerotic plaque inflammation, Nat. Commun., 2014, 5, 3065 CrossRef PubMed.
- C. Jiang, Z. Qi, Y. Tang, H. Jia, Z. Li, W. Zhang and J. Liu, Rational Design of Lovastatin-Loaded Spherical Reconstituted High Density Lipoprotein for Efficient and Safe Anti-Atherosclerotic Therapy, Mol. Pharm., 2019, 16, 3284–3291 CrossRef CAS PubMed.
- Y. Guo, W. Yuan, B. Yu, R. Kuai, W. Hu, E. E. Morin, M. T. Garcia-Barrio, J. Zhang, J. J. Moon, A. Schwendeman and Y. E. Chen, Synthetic High-Density Lipoprotein-Mediated Targeted Delivery of Liver X Receptors Agonist Promotes Atherosclerosis Regression, EBioMedicine, 2018, 28, 225–233 CrossRef PubMed.
- M. C. Brulhart-Meynet, V. Braunersreuther, J. Brinck, F. Montecucco, J. C. Prost, A. Thomas, K. Galan, G. Pelli, S. Pedretti, N. Vuilleumier, F. Mach, S. Lecour, R. W. James and M. A. Frias, Improving reconstituted HDL composition for efficient post-ischemic reduction of ischemia reperfusion injury, PLoS One, 2015, 10, e0119664 CrossRef PubMed.
- M. Lameijer, T. Binderup, M. M. T. van Leent, M. L. Senders, F. Fay, J. Malkus, B. L. Sanchez-Gaytan, A. J. P. Teunissen, N. Karakatsanis, P. Robson, X. Zhou, Y. Ye, G. Wojtkiewicz, J. Tang, T. T. P. Seijkens, J. Kroon, E. S. G. Stroes, A. Kjaer, J. Ochando, T. Reiner, C. Perez-Medina, C. Calcagno, E. A. Fisher, B. Zhang, R. E. Temel, F. K. Swirski, M. Nahrendorf, Z. A. Fayad, E. Lutgens, W. J. M. Mulder and R. Duivenvoorden, Efficacy and safety assessment of a TRAF6-targeted nanoimmunotherapy in atherosclerotic mice and non-human primates, Nat. Biomed. Eng., 2018, 2, 279–292 CrossRef CAS.
- C. Wolfrum, S. Shi, K. N. Jayaprakash, M. Jayaraman, G. Wang, R. K. Pandey, K. G. Rajeev, T. Nakayama, K. Charrise, E. M. Ndungo, T. Zimmermann, V. Koteliansky, M. Manoharan and M. Stoffel, Mechanisms and optimization of in vivo delivery of lipophilic siRNAs, Nat. Biotechnol., 2007, 25, 1149–1157 CrossRef CAS PubMed.
- T. Nakayama, J. S. Butler, A. Sehgal, M. Severgnini, T. Racie, J. Sharman, F. Ding, S. S. Morskaya, J. Brodsky, L. Tchangov, V. Kosovrasti, M. Meys, L. Nechev, G. Wang, C. G. Peng, Y. Fang, M. Maier, K. G. Rajeev, R. Li, J. Hettinger, S. Barros, V. Clausen, X. Zhang, Q. Wang, R. Hutabarat, N. V. Dokholyan, C. Wolfrum, M. Manoharan, V. Kotelianski, M. Stoffel and D. W. Sah, Harnessing a physiologic mechanism for siRNA delivery with mimetic lipoprotein particles, Mol. Ther., 2012, 20, 1582–1589 CrossRef CAS.
- L. F. Cuesta Torres, W. Zhu, G. Ohrling, R. Larsson, M. Patel, C. B. Wiese, K. A. Rye, K. C. Vickers and F. Tabet, High-density lipoproteins induce miR-223–3p biogenesis and export from myeloid cells: Role of scavenger receptor BI-mediated lipid transfer, Atherosclerosis, 2019, 286, 20–29 CrossRef CAS PubMed.
- Y. Zhao, H. Gao, J. He, C. Jiang, J. Lu, W. Zhang, H. Yang and J. Liu, Co-delivery of LOX-1 siRNA and statin to endothelial cells and macrophages in the atherosclerotic lesions by a dual-targeting core-shell nanoplatform: A dual cell therapy to regress plaques, J. Controlled Release, 2018, 283, 241–260 CrossRef CAS PubMed.
- C. Jiang, Z. Qi, W. He, Z. Li, Y. Tang, Y. Wang, Y. Huang, H. Zang, H. Yang and J. Liu, Dynamically enhancing plaque targeting via a positive feedback loop using multifunctional biomimetic nanoparticles for plaque regression, J. Controlled Release, 2019, 308, 71–85 CrossRef CAS.
- D. P. Cormode, T. Skajaa, M. M. van Schooneveld, R. Koole, P. Jarzyna, M. E. Lobatto, C. Calcagno, A. Barazza, R. E. Gordon, P. Zanzonico, E. A. Fisher, Z. A. Fayad and W. J. Mulder, Nanocrystal core high-density lipoproteins: a multimodality contrast agent platform, Nano Lett., 2008, 8, 3715–3723 CrossRef CAS PubMed.
- T. Skajaa, Y. Zhao, D. J. van den Heuvel, H. C. Gerritsen, D. P. Cormode, R. Koole, M. M. van Schooneveld, J. A. Post, E. A. Fisher, Z. A. Fayad, C. de Mello Donega, A. Meijerink and W. J. Mulder, Quantum dot and Cy5.5 labeled nanoparticles to investigate lipoprotein biointeractions via Forster resonance energy transfer, Nano Lett., 2010, 10, 5131–5138 CrossRef CAS.
- W. Chen, D. P. Cormode, Y. Vengrenyuk, B. Herranz, J. E. Feig, A. Klink, W. J. Mulder, E. A. Fisher and Z. A. Fayad, Collagen-specific peptide conjugated HDL nanoparticles as MRI contrast agent to evaluate compositional changes in atherosclerotic plaque regression, JACC Cardiovasc. Imaging, 2013, 6, 373–384 CrossRef PubMed.
- A. J. Luthi, H. Zhang, D. Kim, D. A. Giljohann, C. A. Mirkin and C. S. Thaxton, Tailoring of biomimetic high-density lipoprotein nanostructures changes cholesterol binding and efflux, ACS Nano, 2012, 6, 276–285 CrossRef CAS PubMed.
- Y. Liu, K. M. Castro Bravo and J. Liu, Targeted liposomal drug delivery: a nanoscience and biophysical perspective, Nanoscale Horiz., 2021, 6, 78–94 RSC.
- R. E. Counsell and R. C. Pohland, Lipoproteins as potential site-specific delivery systems for diagnostic and therapeutic agents, J. Med. Chem., 1982, 25, 1115–1120 CrossRef CAS PubMed.
- J. J. Badimon, L. Badimon, A. Galvez, R. Dische and V. Fuster, High density lipoprotein plasma fractions inhibit aortic fatty streaks in cholesterol-fed rabbits, Lab. Invest., 1989, 60, 455–461 CAS.
- J. J. Badimon, L. Badimon and V. Fuster, Regression of atherosclerotic lesions by high density lipoprotein plasma fraction in the cholesterol-fed rabbit, J. Clin. Invest., 1990, 85, 1234–1241 CrossRef CAS PubMed.
- A. Miyazaki, S. Sakuma, W. Morikawa, T. Takiue, F. Miake, T. Terano, M. Sakai, H. Hakamata, Y. Sakamoto and M. Natio,
et al., Intravenous injection of rabbit apolipoprotein A-I inhibits the progression of atherosclerosis in cholesterol-fed rabbits, Arterioscler., Thromb., Vasc. Biol., 1995, 15, 1882–1888 CrossRef CAS PubMed.
- S. Ameli, A. Hultgardh-Nilsson, B. Cercek, P. K. Shah, J. S. Forrester, H. Ageland and J. Nilsson, Recombinant apolipoprotein A-I Milano reduces intimal thickening after balloon injury in hypercholesterolemic rabbits, Circulation, 1994, 90, 1935–1941 CrossRef CAS PubMed.
- M. N. Nanjee, J. R. Crouse, J. M. King, R. Hovorka, S. E. Rees, E. R. Carson, J. J. Morgenthaler, P. Lerch and N. E. Miller, Effects of intravenous infusion of lipid-free apo A-I in humans, Arterioscler., Thromb., Vasc. Biol., 1996, 16, 1203–1214 CrossRef CAS PubMed.
- F. M. Sacks, L. L. Rudel, A. Conner, H. Akeefe, G. Kostner, T. Baki, G. Rothblat, M. de la Llera-Moya, B. Asztalos, T. Perlman, C. Zheng, P. Alaupovic, J. A. Maltais and H. B. Brewer, Selective delipidation of plasma HDL enhances reverse cholesterol transport in vivo, J. Lipid Res., 2009, 50, 894–907 CrossRef CAS PubMed.
- R. Waksman, R. Torguson, K. M. Kent, A. D. Pichard, W. O. Suddath, L. F. Satler, B. D. Martin, T. J. Perlman, J. A. Maltais, N. J. Weissman, P. J. Fitzgerald and H. B. Brewer Jr., A first-in-man, randomized, placebo-controlled study to evaluate the safety and feasibility of autologous delipidated high-density lipoprotein plasma infusions in patients with acute coronary syndrome, J. Am. Coll. Cardiol., 2010, 55, 2727–2735 CrossRef PubMed.
- G. Romano, S. Reggi, B. Kutryb-Zajac, A. Facoetti, E. Chisci, M. Pettinato, M. R. Giuffre, F. Vecchio, S. Leoni, M. De Giorgi, F. Avezza, M. Cadamuro, L. Crippa, B. E. Leone, M. Lavitrano, I. Rivolta, D. Barisani, R. T. Smolenski and R. Giovannoni, APOA-1Milano muteins, orally delivered via genetically modified rice, show anti-atherogenic and anti-inflammatory properties in vitro and in Apoe(-/-) atherosclerotic mice, Int. J. Cardiol., 2018, 271, 233–239 CrossRef PubMed.
- J. Morton, S. Bao, L. Z. Vanags, T. Tsatralis, A. Ridiandries, C. W. Siu, K. M. Ng, J. T. M. Tan, D. S. Celermajer, M. K. C. Ng and C. A. Bursill, Strikingly Different Atheroprotective Effects of Apolipoprotein A-I in Early- Versus Late-Stage Atherosclerosis, JACC Basic Transl. Sci., 2018, 3, 187–199 CrossRef PubMed.
- S. J. Edmunds, R. Liebana-Garcia, O. Nilsson, J. Domingo-Espin, C. Gronberg, K. G. Stenkula and J. O. Lagerstedt, ApoAI-derived peptide increases glucose tolerance and prevents formation of atherosclerosis in mice, Diabetologia, 2019, 62, 1257–1267 CrossRef CAS PubMed.
- R. Kuai, D. Li, Y. E. Chen, J. J. Moon and A. Schwendeman, High-Density Lipoproteins: Nature's Multifunctional Nanoparticles, ACS Nano, 2016, 10, 3015–3041 CrossRef CAS PubMed.
- L. T. Bloedon, R. Dunbar, D. Duffy, P. Pinell-Salles, R. Norris, B. J. DeGroot, R. Movva, M. Navab, A. M. Fogelman and D. J. Rader, Safety, pharmacokinetics, and pharmacodynamics of oral apoA-I mimetic peptide D-4F in high-risk cardiovascular patients, J. Lipid Res., 2008, 49, 1344–1352 CrossRef CAS PubMed.
- C. E. Watson, N. Weissbach, L. Kjems, S. Ayalasomayajula, Y. Zhang, I. Chang, M. Navab, S. Hama, G. Hough, S. T. Reddy, D. Soffer, D. J. Rader, A. M. Fogelman and A. Schecter, Treatment of patients with cardiovascular disease with L-4F, an apo-A1 mimetic, did not improve select biomarkers of HDL function, J. Lipid Res., 2011, 52, 361–373 CrossRef CAS PubMed.
- R. L. Dunbar, R. Movva, L. T. Bloedon, D. Duffy, R. B. Norris, M. Navab, A. M. Fogelman and D. J. Rader, Oral Apolipoprotein A-I Mimetic D-4F Lowers HDL-Inflammatory Index in High-Risk Patients: A First-in-Human Multiple-Dose, Randomized Controlled Trial, Clin. Transl. Sci., 2017, 10, 455–469 CrossRef CAS PubMed.
- M. N. Nanjee, J. E. Doran, P. G. Lerch and N. E. Miller, Acute effects of intravenous infusion of ApoA1/phosphatidylcholine discs on plasma lipoproteins in humans, Arterioscler., Thromb., Vasc. Biol., 1999, 19, 979–989 CrossRef CAS PubMed.
- L. E. Spieker, I. Sudano, D. Hurlimann, P. G. Lerch, M. G. Lang, C. Binggeli, R. Corti, F. Ruschitzka, T. F. Luscher and G. Noll, High-density lipoprotein restores endothelial function in hypercholesterolemic men, Circulation, 2002, 105, 1399–1402 CrossRef CAS PubMed.
- J. C. Tardif, J. Gregoire, P. L. L'Allier, R. Ibrahim, J. Lesperance, T. M. Heinonen, S. Kouz, C. Berry, R. Basser, M. A. Lavoie, M. C. Guertin, J. Rodes-Cabau and H.D.L.o.A.-S. Effect of r I. Efficacy, Effects of reconstituted high-density lipoprotein infusions on coronary atherosclerosis: a randomized controlled trial, JAMA, J. Am. Med. Assoc., 2007, 297, 1675–1682 CrossRef PubMed.
- A. Gille, R. Easton, D. D'Andrea, S. D. Wright and C. L. Shear, CSL112 enhances biomarkers of reverse cholesterol transport after single and multiple infusions in healthy subjects, Arterioscler., Thromb., Vasc. Biol., 2014, 34, 2106–2114 CrossRef CAS PubMed.
- A. Gille, D. D'Andrea, M. A. Tortorici, G. Hartel and S. D. Wright, CSL112 (Apolipoprotein A-I [Human]) Enhances Cholesterol Efflux Similarly in Healthy Individuals and Stable Atherosclerotic Disease Patients, Arterioscler., Thromb., Vasc. Biol., 2018, 38, 953–963 CrossRef CAS PubMed.
- P. Tricoci, D. M. D'Andrea, P. A. Gurbel, Z. Yao, M. Cuchel, B. Winston, R. Schott, R. Weiss, M. A. Blazing, L. Cannon, A. Bailey, D. J. Angiolillo, A. Gille, C. L. Shear, S. D. Wright and J. H. Alexander, Infusion of Reconstituted High-Density Lipoprotein, CSL112, in Patients With Atherosclerosis: Safety and Pharmacokinetic Results From a Phase 2a Randomized Clinical Trial, J. Am. Heart Assoc., 2015, 4, e002171 Search PubMed.
- C. Michael Gibson, S. Korjian, P. Tricoci, Y. Daaboul, M. Yee, P. Jain, J. H. Alexander, P. G. Steg, A. M. Lincoff, J. J. Kastelein, R. Mehran, D. M. D'Andrea, L. I. Deckelbaum, B. Merkely, M. Zarebinski, T. O. Ophuis and R. A. Harrington, Safety and Tolerability of CSL112, a Reconstituted, Infusible, Plasma-Derived Apolipoprotein A-I, After Acute Myocardial Infarction: The AEGIS-I Trial (ApoA-I Event Reducing in Ischemic Syndromes I), Circulation, 2016, 134, 1918–1930 CrossRef CAS PubMed.
- C. M. Gibson, J. J. P. Kastelein, A. T. Phillips, P. E. Aylward, M. K. Yee, M. Tendera, S. J. Nicholls, S. Pocock, S. G. Goodman, J. H. Alexander, A. M. Lincoff, C. Bode, D. Duffy, M. Heise, G. Berman, S. J. Mears, P. Tricoci, L. I. Deckelbaum, P. G. Steg, P. Ridker and R. Mehran, Rationale and design of ApoA-I Event Reducing in Ischemic Syndromes II (AEGIS-II): A phase 3, multicenter, double-blind, randomized, placebo-controlled, parallel-group study to investigate the efficacy and safety of CSL112 in subjects after acute myocardial infarction, Am. Heart J., 2021, 231, 121–127 CrossRef CAS PubMed.
- C. L. Bisgaier, R. Ackermann, T. Rea, W. V. Rodrigueza and D. Hartman, ApoA-IMilano phospholipid complex (ETC-216) infusion in human volunteers. Insights into the phenotypic characteristics of ApoA-IMilano carriers, Pharmacol. Res., 2016, 111, 86–99 CrossRef CAS PubMed.
- S. E. Nissen, T. Tsunoda, E. M. Tuzcu, P. Schoenhagen, C. J. Cooper, M. Yasin, G. M. Eaton, M. A. Lauer, W. S. Sheldon, C. L. Grines, S. Halpern, T. Crowe, J. C. Blankenship and R. Kerensky, Effect of recombinant ApoA-I Milano on coronary atherosclerosis in patients with acute coronary syndromes: a randomized controlled trial, JAMA, J. Am. Med. Assoc., 2003, 290, 2292–2300 CrossRef CAS PubMed.
- J. A. A. Reijers, D. G. Kallend, K. E. Malone, J. W. Jukema, P. L. J. Wijngaard, J. Burggraaf and M. Moerland, MDCO-216 Does Not Induce Adverse Immunostimulation, in Contrast to Its Predecessor ETC-216, Cardiovasc. Drugs Ther., 2017, 31, 381–389 CrossRef CAS PubMed.
- S. J. Nicholls, R. Puri, C. M. Ballantyne, J. W. Jukema, J. J. P. Kastelein, W. Koenig, R. S. Wright, D. Kallend, P. Wijngaard, M. Borgman, K. Wolski and S. E. Nissen, Effect of Infusion of High-Density Lipoprotein Mimetic Containing Recombinant Apolipoprotein A-I Milano on Coronary Disease in Patients With an Acute Coronary Syndrome in the MILANO-PILOT Trial: A Randomized Clinical Trial, JAMA Cardiol., 2018, 3, 806–814 CrossRef PubMed.
- G. K. Hovingh, L. P. Smits, C. Stefanutti, H. Soran, S. Kwok, J. de Graaf, D. Gaudet, C. H. Keyserling, H. Klepp, J. Frick, J. F. Paolini, J. L. Dasseux, J. J. Kastelein and E. S. Stroes, The effect of an apolipoprotein A-I-containing high-density lipoprotein-mimetic particle (CER-001) on carotid artery wall thickness in patients with homozygous familial hypercholesterolemia: The Modifying Orphan Disease Evaluation (MODE) study, Am. Heart J., 2015, 169, 736–742 CrossRef CAS PubMed.
- Y. Kataoka, J. Andrews, M. Duong, T. Nguyen, N. Schwarz, J. Fendler, R. Puri, J. Butters, C. Keyserling, J. F. Paolini, J. L. Dasseux and S. J. Nicholls, Regression of coronary atherosclerosis with infusions of the high-density lipoprotein mimetic CER-001 in patients with more extensive plaque burden, Cardiovasc. Diagn. Ther., 2017, 7, 252–263 CrossRef PubMed.
- S. J. Nicholls, J. Andrews, J. J. P. Kastelein, B. Merkely, S. E. Nissen, K. K. Ray, G. G. Schwartz, S. G. Worthley, C. Keyserling, J. L. Dasseux, L. Griffith, S. W. Kim, A. Janssan, G. Di Giovanni, A. D. Pisaniello, D. J. Scherer, P. J. Psaltis and J. Butters, Effect of Serial Infusions of CER-001, a Pre-beta High-Density Lipoprotein Mimetic, on Coronary Atherosclerosis in Patients Following Acute Coronary Syndromes in the CER-001 Atherosclerosis Regression Acute Coronary Syndrome Trial: A Randomized Clinical Trial, JAMA Cardiol., 2018, 3, 815–822 CrossRef PubMed.
- R. S. Kootte, L. P. Smits, F. M. van der Valk, J. L. Dasseux, C. H. Keyserling, R. Barbaras, J. F. Paolini, R. D. Santos, T. H. van Dijk, G. M. Dallinga-van Thie, A. J. Nederveen, W. J. Mulder, G. K. Hovingh, J. J. Kastelein, A. K. Groen and E. S. Stroes, Effect of open-label infusion of an apoA-I-containing particle (CER-001) on RCT and artery wall thickness in patients with FHA, J. Lipid Res., 2015, 56, 703–712 CrossRef CAS PubMed.
- L. Liu, H. He, M. Zhang, S. Zhang, W. Zhang and J. Liu, Hyaluronic acid-decorated reconstituted high density lipoprotein targeting atherosclerotic lesions, Biomaterials, 2014, 35, 8002–8014 CrossRef CAS PubMed.
- H. He, M. Zhang, L. Liu, S. Zhang, J. Liu and W. Zhang, Suppression of Remodeling Behaviors with Arachidonic Acid Modification for Enhanced in vivo Antiatherogenic Efficacies of Lovastatin-loaded Discoidal Recombinant High Density Lipoprotein, Pharm. Res., 2015, 32, 3415–3431 CrossRef CAS PubMed.
- A. Shaish, G. Keren, P. Chouraqui, H. Levkovitz and D. Harats, Imaging of aortic atherosclerotic lesions by (125)I-LDL, (125)I-oxidized-LDL, (125)I-HDL and (125)I-BSA, Pathobiology, 2001, 69, 225–229 CrossRef CAS PubMed.
- J. C. Frias, K. J. Williams, E. A. Fisher and Z. A. Fayad, Recombinant HDL-like nanoparticles: a specific contrast agent for MRI of atherosclerotic plaques, J. Am. Chem. Soc., 2004, 126, 16316–16317 CrossRef CAS PubMed.
- J. C. Frias, Y. Ma, K. J. Williams, Z. A. Fayad and E. A. Fisher, Properties of a versatile nanoparticle platform contrast agent to image and characterize atherosclerotic plaques by magnetic resonance imaging, Nano Lett., 2006, 6, 2220–2224 CrossRef CAS PubMed.
- D. P. Cormode, K. C. Briley-Saebo, W. J. Mulder, J. G. Aguinaldo, A. Barazza, Y. Ma, E. A. Fisher and Z. A. Fayad, An ApoA-I mimetic peptide high-density-lipoprotein-based MRI contrast agent for atherosclerotic plaque composition detection, Small, 2008, 4, 1437–1444 CrossRef CAS PubMed.
- D. P. Cormode, R. Chandrasekar, A. Delshad, K. C. Briley-Saebo, C. Calcagno, A. Barazza, W. J. Mulder, E. A. Fisher and Z. A. Fayad, Comparison of synthetic high density lipoprotein (HDL) contrast agents for MR imaging of atherosclerosis, Bioconjugate Chem., 2009, 20, 937–943 CrossRef CAS PubMed.
- A. B. Sigalov, Nature-inspired nanoformulations for contrast-enhanced in vivo MR imaging of macrophages, Contrast Media Mol. Imaging, 2014, 9, 372–382 CrossRef CAS PubMed.
- Z. T. Shen, S. Zheng, M. J. Gounis and A. B. Sigalov, Diagnostic Magnetic Resonance Imaging of Atherosclerosis in Apolipoprotein E Knockout Mouse Model Using Macrophage-Targeted Gadolinium-Containing Synthetic Lipopeptide Nanoparticles, PLoS One, 2015, 10, e0143453 CrossRef PubMed.
- R. Sriram, J. O. Lagerstedt, J. Petrlova, H. Samardzic, U. Kreutzer, H. Xie, G. A. Kaysen, J. F. Desreux, D. Thonon, V. Jacques, M. Van Loan, J. C. Rutledge, M. N. Oda, J. C. Voss and T. Jue, Imaging apolipoprotein AI in vivo, NMR Biomed., 2011, 24, 916–924 CrossRef CAS PubMed.
- C. Perez-Medina, T. Binderup, M. E. Lobatto, J. Tang, C. Calcagno, L. Giesen, C. H. Wessel, J. Witjes, S. Ishino, S. Baxter, Y. Zhao, S. Ramachandran, M. Eldib, B. L. Sanchez-Gaytan, P. M. Robson, J. Bini, J. F. Granada, K. M. Fish, E. S. Stroes, R. Duivenvoorden, S. Tsimikas, J. S. Lewis, T. Reiner, V. Fuster, A. Kjaer, E. A. Fisher, Z. A. Fayad and W. J. Mulder, In Vivo PET Imaging of HDL in Multiple Atherosclerosis Models, JACC Cardiovasc. Imaging, 2016, 9, 950–961 CrossRef PubMed.
- E. Kawachi, Y. Uehara, K. Hasegawa, E. Yahiro, S. Ando, Y. Wada, T. Yano, H. Nishikawa, M. Shiomi, S. Miura, Y. Watanabe and K. Saku, Novel molecular imaging of atherosclerosis with gallium-68-labeled apolipoprotein A-I mimetic peptide and positron emission tomography, Circ. J., 2013, 77, 1482–1489 CrossRef CAS PubMed.
- J. Yong-Sang, F. Dioury, V. Meneyrol, I. Ait-Arsa, J. P. Idoumbin, F. Guibbal, J. Patche, F. Gimie, I. Khantalin, J. Couprie, P. Giraud, S. Benard, C. Ferroud, E. Jestin and O. Meilhac, Development, synthesis, and (68)Ga-Labeling of a Lipophilic complexing agent for atherosclerosis PET imaging, Eur. J. Med. Chem., 2019, 176, 129–134 CrossRef CAS PubMed.
- D. P. Cormode, E. Roessl, A. Thran, T. Skajaa, R. E. Gordon, J. P. Schlomka, V. Fuster, E. A. Fisher, W. J. Mulder, R. Proksa and Z. A. Fayad, Atherosclerotic plaque composition: analysis with multicolor CT and targeted gold nanoparticles, Radiology, 2010, 256, 774–782 CrossRef PubMed.
- K. H. Zheng, F. M. van der Valk, L. P. Smits, M. Sandberg, J. L. Dasseux, R. Baron, R. Barbaras, C. Keyserling, B. F. Coolen, A. J. Nederveen, H. J. Verberne, T. E. Nell, D. J. Vugts, R. Duivenvoorden, Z. A. Fayad, W. J. M. Mulder, G. van Dongen and E. S. G. Stroes, HDL mimetic CER-001 targets atherosclerotic plaques in patients, Atherosclerosis, 2016, 251, 381–388 CrossRef CAS PubMed.
- S. Jebari-Benslaiman, K. B. Uribe, A. Benito-Vicente, U. Galicia-Garcia, A. Larrea-Sebal, I. Alloza, K. Vandenbroeck, H. Ostolaza and C. Martin, Cholesterol Efflux Efficiency of Reconstituted HDL Is Affected by Nanoparticle Lipid Composition, Biomedicines, 2020, 8, 373 CrossRef CAS PubMed.
- E. E. Morin, Y. Guo, H. He, W. Yuan, W. N. Souery, M. V. Fawaz, Y. E. Chen and A. Schwendeman, Synergetic Effect of rHDL and LXR Agonist on Reduction of Atherosclerosis in Mice, Front. Pharmacol., 2020, 11, 513031 CrossRef CAS PubMed.
Footnote |
† Electronic supplementary information (ESI) available. See DOI: 10.1039/d0bm01838d |
|
This journal is © The Royal Society of Chemistry 2021 |