DOI:
10.1039/D0BM00617C
(Review Article)
Biomater. Sci., 2021,
9, 10-22
Photothermal bactericidal surfaces: killing bacteria using light instead of biocides
Received
18th April 2020
, Accepted 22nd May 2020
First published on 22nd May 2020
Abstract
Endowing the surfaces of synthetic materials with bactericidal activity is a direct and effective way to prevent bacterial colonization and biofilm formation, solving the related serious problems such as contamination, infection and biofouling. Conventional bactericidal surfaces are usually based on biocidal agents such as antibiotics to kill attached bacteria; however, such surfaces have inherent limitations from their respective biocidal agents and most of them become less effective against the so-called “super bacteria” with multidrug-resistance. In recent years, photothermal bactericidal surfaces have become a promising alternative for combating surface-attached bacteria. These surfaces rely on immobilized photothermal agents, which can convert light energy into thermal energy to effectively eliminate bacteria through various hyperthermia effects, showing several advantages including broad-spectrum sterilization ability, no drug resistance and few side effects. In this review, we highlight the recent development of these photothermal bactericidal surfaces, which are categorized into three types according to the photothermal agents. Multi-functional photothermal bactericidal surfaces with either integrated synergistic killing mechanisms or capability to switch function between killing bacteria and releasing bacteria are also introduced. A brief perspective is finally presented on the directions that show promise for the future.
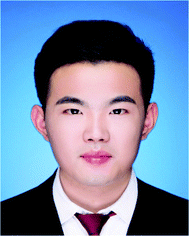 Yi Zou | Yi Zou is a master's candidate under the supervision of Prof. Qian Yu at the College of Chemistry, Chemical Engineering and Materials Science at Soochow University. He received his BS degree from the Nanjing Institute of Technology in 2019. His research is focused on multifunctional biointerfaces. |
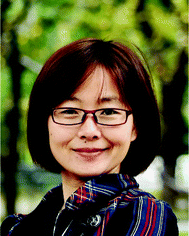 Yanxia Zhang | Yanxia Zhang is an associate professor at the Institute for Cardiovascular Science at Soochow University. She received her Ph.D. degree from the Wuhan University of Technology in 2011, and then worked in the Department of Forest Biomaterials at the North Carolina State University as a postdoctoral researcher (2012–2014). In 2015 she joined Soochow University and was appointed as an associate professor. Her research interests include bioactive surfaces and biomaterials based on cellulose, biomaterials for the treatment of myocardial infarction, and label-free biosensors. |
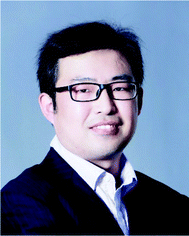 Qian Yu | Qian Yu is a professor at the College of Chemistry, Chemical Engineering and Materials Science at Soochow University. He received his Ph.D. degree from the Wuhan University of Technology in 2011, and then worked in the Department of Biomedical Engineering in Duke University as a postdoctoral associate (2011–2014). In 2014, he joined Soochow University. He became the editor of Colloid and Interface Science Communications (Elsevier) in 2017. His research interests include the development of stimuli-responsive polymers for applications in biomedical and biotechnology fields, antibacterial surfaces, and surface-mediated intracellular delivery. |
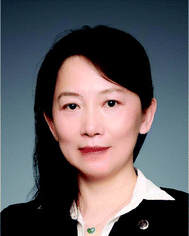 Hong Chen | Hong Chen is a professor at the College of Chemistry, Chemical Engineering and Materials Science at Soochow University. She earned her Ph.D. degree from Nanjing University. She was a postdoc at McMaster University (2001–2004) and then held a full professor position at the Wuhan University of Technology (2004–2009). Her research group moved to Soochow University in 2010. She was the associate editor of Polymer Chemistry (RSC) from 2016 to 2020 and then she became the editor of Colloids and Surface B: Biointerfaces (Elsevier). Her research interests include surface modification and functionalization of biomaterials, hemocompatibility of biomaterials, and interaction of protein/cell and biomaterials. |
1 Introduction
Attachment and subsequent colonization of bacteria on the material surfaces usually result in the formation of biofilms, which not only cause the deterioration of surface properties and functions to limit the service life of industrial devices, but also pose serious problems in public health due to the related contamination and biofouling.1–3 In particular, biofilms resulting from pathogenic bacteria on the surface of implant materials and surgical devices cause severe chronic infections, clinical complications, and even death.4 The development of antibacterial surfaces to counter bacterial colonization and biofilm formation has been proven to be an effective solution to deal with these problems, and thus has attracted considerable attention from both scientists and engineers.5–7 Over the past decades, various antibacterial surfaces with different working mechanisms have been developed (i) to resist the initial attachment of bacteria, (ii) to kill the attached bacteria, or (iii) to release the loosely-attached bacteria by applying external stimuli.8 Among these antibacterial approaches, endowing the surfaces with bactericidal ability to eliminate the bacteria that either attach on the surface or suspend in the surrounding environment is the most straightforward method, and remarkable progress has been made in the development of such bactericidal surfaces.9,10
In general, conventional bactericidal surfaces are based on the biocidal agents that are either permanently immobilized on the surfaces or pre-loaded/embedded within the surfaces before being released, including natural antibacterial molecules (e.g. antimicrobial enzymes and antimicrobial peptides), antimicrobial peptide-mimicking compounds, synthetic cationic polymers, nitrogen oxide, metal nanoparticles, and the most commonly used biocidal agents such as antibiotics.11,12 Although these bactericidal surfaces exhibited effectiveness against most common bacterial strains, they still have inherent limitations resulting from their respective biocidal agents. For example, natural antibacterial molecules usually do not have a wide variety of sources, and their bioactivities are always affected by environmental factors such as temperature and pH.13 Antimicrobial peptide-mimicking compounds are limited due to their difficult synthesis, complex purification, and high cost.14 Synthetic cationic compounds/polymers and metal nanoparticles typically suffer from potential toxicity for certain types of mammalian cells and tissues.15,16 Worst of all, although antibiotics have played critical roles in treating pathogenic infections caused by bacteria for a long time, the overuse or misuse of broad spectrum antibiotics has led to the emergence and rapid increase of the so-called “super bacteria” with multidrug-resistance (such as methicillin-resistant Staphylococcus aureus (MRSA), vancomycin-resistant Enterococcus faecium (VREF), and multidrug-resistant Acinetobacter baumannii (MRAB)), resulting in a serious threat to global public health.17,18 Therefore, it is urgent to develop novel bactericidal surfaces based on other killing mechanisms without using traditional biocidal agents.
Antibacterial photothermal therapy (APTT) is a kind of physical sterilization method that relies on the local heat generated by photothermal agents (PTAs) under proper light irradiation to efficiently kill bacteria through various hyperthermia effects such as cell membrane rupture, protein/enzyme denaturation, cell cavitation, and evaporation of cellular liquid.19,20 Compared to traditional bactericidal methods based on biocidal agents, APTT has unique advantages such as broad-spectrum sterilization ability, no drug resistance and few side effects.21 The use of PTAs under light irradiation has been widely adopted to eliminate suspended bacteria for solution sterilization.22–24 It is thus expected that the immobilization of PTAs on the material surfaces to achieve photothermal bactericidal properties will solve the shortcomings of biocidal agent-based bactericidal surfaces, showing great promise for combining surface-attached bacteria, in particular the multidrug-resistant bacteria.
In this review, we focus on the recent developments of photothermal bactericidal surfaces. We firstly introduce the fabrication of photothermal bactericidal surfaces and categorize these surfaces into three types according to the PTAs including (i) gold nanomaterials, (ii) graphene derivatives, and (iii) other PTAs. We then introduce two types of multi-functional photothermal bactericidal surfaces with either integrated synergistic killing mechanisms or capability to switch function between killing bacteria and releasing bacteria in response to an external stimulus. Finally, we conclude with a brief perspective on approaches that show promise for the future.
2 Fabrication of photothermal bactericidal surfaces
The main component of photothermal bactericidal surfaces is appropriate PTAs that can absorb light of appropriate wavelength and convert part of absorbed light energy into thermal energy. Conventional PTAs include noble metal nanomaterials such as gold nanomaterials,25 two-dimensional layered nanomaterials such as graphene derivatives,26–28 and other organic or inorganic compounds,29 which have been used for the fabrication of photothermal bactericidal surfaces (representative examples are listed in Table 1).
Table 1 Summary of photothermal bactericidal surfaces fabricated using different PTAsa
PTA |
Substrate |
Combination method |
Microorganism |
Ref. |
Abbreviations: GNP, gold nanoparticle; GNR, gold nanorod; GNSt, gold nanostar; GNSh, gold nanoshell; PVA, polyvinyl alcohol; PA, polyamide; PDMS, polydimethylsiloxane; GO, graphene oxide; rGO, reduced graphene oxide; PES, polyether sulfone; BNC, bacterial nanocellulose; RP, red phosphorus; PDA, polydopamine; PANI, polyaniline; PEDOT:PSS, poly3,4-ethylenediox-ythiophene:polystyrene-sulfonate; TA, tannic acid; CA, cellulose acetate; PET, polyethylene terephthalate; CS, chitosan; SF, silk fibroin; E. coli, Escherichia coli; S. aureus, Staphylococcus aureus; P. aeruginosa, Pseudomonas aeruginosa; S. epidermidis, Staphylococcus epidermidis; E. faecalis, Enterococcus faecalis; B. subtilis, Bacillus subtilis; C. albicans, Candida albicans; MRSA, methicillin-resistant Staphylococcus aureus.
|
Gold nanomaterials
|
GNP |
Silicone rubber |
Physical deposition |
S. aureus
|
30
|
GNP |
Titanium |
Physical deposition |
S. aureus, E. coli |
31
|
GNP |
Gold |
Chemical deposition |
S. aureus, E. coli, MRSA |
32
|
GNR |
Glass |
Physical deposition |
E. coli
|
33
|
GNR |
Titanium |
Electrostatic self-assembly |
E. coli, S. aureus, P. aeruginosa, S. epidermidis |
34
|
GNSt |
Glass |
Covalent bonding |
S. aureus, E. coli |
35–37
|
GNSt |
PVA |
Blending |
E. coli
|
38
|
GNSt |
PA |
Blending |
E. coli
|
39
|
GNSh |
PDMS |
Covalent bonding |
E. faecalis
|
40
|
Two-dimensional nanomaterials
|
GO |
Glass |
LBL |
E. coli
|
41
|
GO |
PES |
LBL |
S. aureus, E. coli |
42
|
rGO |
Quartz |
LBL |
E. coli, S. aureus, P. aeruginosa, B. subtilis |
43
|
rGO |
BNC |
Blending |
E. coli
|
44
|
MoS2 |
Titanium |
Physical deposition |
S. aureus, E. coli |
45 and 46
|
MoS2 |
Titanium |
Hydrothermal growth |
S. aureus, E. coli |
47
|
MnO2 |
Titanium |
Hydrothermal growth |
S. aureus, E. coli |
48
|
Other materials
|
Bi2S3 |
Titanium |
Hydrothermal growth |
S. aureus, E. coli |
49
|
Cs0.33WO3 |
PDMS |
Covalent bonding |
S. aureus, E. coli |
50
|
Cu12Sb4S13 |
CA membrane |
Physical deposition |
E. coli
|
51
|
RP |
Titanium |
Chemical deposition |
S. aureus, E. coli |
52–54
|
PDA |
Glass |
Chemical deposition |
S. aureus, E. coli, C. albicans |
55
|
PANI |
PET |
Ionic interaction |
S. aureus, E. coli |
56
|
PEDOT:PSS |
Agarose |
Hydrogen bonding |
S. aureus, E. coli |
57
|
TA/Fe3+ |
CS/SF |
Chemical deposition |
S. aureus, E. coli |
58
|
TA/Fe3+ |
Gold |
Chemical deposition |
E. coli, MRSA |
59
|
In general, photothermal bactericidal surfaces are fabricated by the immobilization of PTAs on the substrate surfaces through diverse physical and chemical methods. Physical methods usually rely on noncovalent interactions (e.g. hydrophobic interactions, electrostatic interactions or hydrogen bonding), which are flexible, reversible, and easy to operate. One typical example is the layer-by-layer (LBL) assembly based on electrostatic interactions to simply incorporate PTAs into multilayered films on the surfaces. Differently, chemical methods usually rely on the covalent bonds formed between PTAs and surfaces or PTAs and surface-tethered spacer/linker molecules, which enhance the stability of the surface-immobilized PTAs and thus are beneficial for long-term use. In the following section, we will introduce the recent progress of photothermal bactericidal surfaces according to the PTAs used.
2.1 Photothermal bactericidal surfaces based on gold nanomaterials
Gold nanomaterials are a family of typical PTAs.60 The photothermal effect of gold nanomaterials is based on the localized surface plasmon resonance (LSPR), which arises from the collective oscillation of free carriers in nanomaterials driven by the electromagnetic field of incident light, resulting in an increase in the kinetic energy and causing the local environment around light-absorbing species to overheat.61 Previous studies have shown that the absorption and scattering rates of gold nanomaterials and the location of plasmon bands are related to their shape, size, and structure.62 The transmission electron microscopy (TEM) images of some typical gold nanomaterials including gold nanoparticles (GNPs),63 gold nanorods (GNRs),64 gold nanostars (GNSts),65 and gold nanoshells (GNShs)66 are shown in Fig. 1.
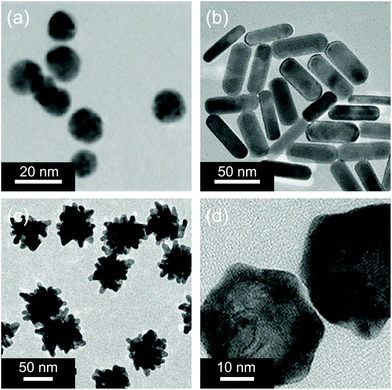 |
| Fig. 1 Representative TEM images of (a) gold nanoparticles, (b) gold nanorods, (c) gold nanostars, and (d) gold nanoshells. Reproduced with permission from (a) ref. 63, copyright 2015, American Chemical Society; (b) ref. 64, copyright 2018, American Chemical Society; (c) ref. 65, copyright 2014, Wiley-VCH; and (d) ref. 66, copyright 2017, Wiley-VCH. | |
2.1.1 Gold nanoparticles.
Gold nanoparticles (GNPs) usually are capable of absorbing light of specific wavelength (from visible light to near-infrared (NIR) light) based on their size and morphology, and release energy in the form of heat.67 Alvarez-Lorenzo and coworkers fabricated silicon rubber films grafted with poly(acrylic acid) (PAA) brushes for the immobilization of GNPs via in situ reduction of gold ions trapped by PAA.30 The resulting GNP immobilized films exhibited good stability against ultrasonic treatment and high-pressure sterilization and could kill attached bacteria under the 5 min exposure to a 514 nm laser. Although this proof-of-concept work demonstrated the use of photothermal surfaces for bacterial elimination, it is difficult to be extended for the commonly used indwelling devices because visible light can easily be absorbed and scattered by biological tissues and high-dose irradiation always results in severe tissue damage.
Compared with visible light, NIR light in the range of 700–1100 nm is more attractive for photothermal therapy, because it is capable of penetrating tissues with negligible damage.25 Therefore, it is better to increase the size of GNPs to transfer the absorption wavelength to the NIR region for broader biological applications. To this end, GNPs with an average size of 15 nm were synthesized and then deposited on the TiO2 nanotube arrays.31 After NIR laser (808 nm) irradiation for 15 min, the modified surface showed an excellent bactericidal efficiency of 96.19% against S. aureus and 99.89% against E. coli. Recently, we deposited a layer of GNPs with three-dimensional micro- and nanosized porous structures on the surface of diverse substrates via a chemical plating method using glucose as a reducing agent.32 The deposition process was simple and environmentally friendly and could be applied to a variety of materials with different surface properties to endow them with photothermal antibacterial activity.
2.1.2 Gold nanorods.
Gold nanorods (GNRs) have been extensively explored in photothermal therapy due to their high photothermal conversion capability, resulting from their distinctive LSPR effect.68–71 Moreover, compared with spherical GNPs, GNRs can absorb and scatter laser light wavelengths in a broad range from the visible to NIR region by simply adjusting the aspect ratio (length/width).72 Individual GNRs in solution have been used for the photothermal elimination of pathogenic bacteria and cancer cells;73 however, the dispersed GNRs are easily taken up by normal cells and may compromise cell viability.74 To solve this problem, well-packed GNRs were immobilized on the surface as two- and three-dimensional arrays using confined convective arraying techniques (Fig. 2).33 These concentrated array-type GNRs can form plate-shaped heating sources for the photothermal eradication of pathogenic bacteria, and they showed potential for integration into the water purifying system for the purification of flowing drinking water. In another study, GNRs were deposited on the titanium surface via an electrostatic surface self-assembly technique.34 Under NIR irradiation, the modified surface exhibited bactericidal activity against four kinds of bacterial strains including both Gram-negative bacilli and Gram-positive cocci and did not lead to obvious harmful effects on the mammalian cells. More importantly, during at least 6 repeated laser ON/OFF cycles, the assembling GNRs were firmly anchored on the surface and maintained the photothermal bactericidal capability, suggesting good stability and recyclability.
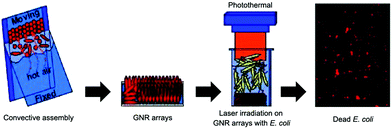 |
| Fig. 2 Schematic illustration of photothermal bactericidal surfaces assembled with ordered GNR arrays. Reproduced with permission from ref. 33, copyright 2014, American Chemical Society. | |
2.1.3 Gold nanostars.
Gold nanostars (GNSts) are a class of anisotropic gold nanomaterials with multiple sharp tips.75 The unique anisotropic structures of GNSts can concentrate the electric field around the sharp tips due to the lightning rod effect. The electromagnetic enhancement produces strong light absorption and effective light-to-heat conversion, making GNSts suitable candidates for APTT.76 For example, Scarabelli and co-workers deposited a monolayer of GNSts on the glass substrates by self-assembly and demonstrated the photothermal bactericidal activity of the resulting surfaces and it was observed that they could effectively kill S. aureus and eradicate the growing biofilm under NIR irradiation.35 On this basis, they further functionalized the GNSt monolayer with hydrophilic thiols via the formation of Au–S bonds (as shown in Fig. 3) to achieve a multifunctional antibacterial surface.36 The hydrophilic thiols did not affect the photothermal properties of the underlying GNSt monolayer, but provided three functions for this system: (i) forming a hydration layer to prevent the initial adhesion of bacteria; (ii) avoiding the recombination of GNSts towards the spherical shape to increase the stability of the GNSt monolayer; and (iii) removing the surfactant molecules used for the growth and synthesis of GNSts to reduce the cytotoxicity of the surface. It is found that pure GNSts tend to aggregate, significantly affecting their light absorption.77 To solve this problem, Jun and co-workers used graphene oxide (GO) nanosheets as templates to grow GNSts for dispersion and stabilization of GNSts. They integrated GO nanosheets, GNSts, and hydrophilic polymer polyethylene glycol into polyamide to construct a four-layered reverse osmosis membrane.39 This resulting composite membrane could efficiently kill E. coli under a 600 s exposure to a NIR laser, and eliminate mineral scale and organic pollution, greatly improving the service life and work efficiency of the membrane itself.
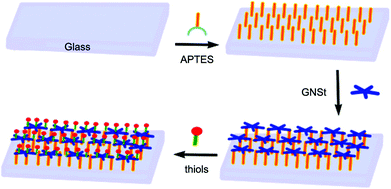 |
| Fig. 3 Schematic illustration of the preparation procedure of a glass surface grafted by the GNSt layer that is functionalized with functional thiols. Reproduced with permission from ref. 36, copyright 2019, MDPI. | |
2.1.4 Gold nanoshells.
Gold nanoshells (GNShs) are usually tunable plasmonic nanostructures consisting of spherical dielectric cores wrapped with a thin layer of gold, and their LSPR can be easily tuned to the NIR region by adjusting the thickness of the gold layer.78 Furthermore, it has been reported that GNShs are noncytotoxic at a physiological dose and do not cause short-term health risks, which make them promising candidates as PTAs for medical applications.79 Pathogenic biofilms on the surface of silicone catheters usually lead to severe infections, which remain a major concern for medical care.80 Aiming at the elimination of bacteria attached on the catheter surface, carboxylic acid-terminated GNShs were conjugated to the surface of polydimethylsiloxane (PDMS) prefunctionalized with amino groups and the NIR-induced bactericidal ability was tested against Enterococcus faecalis (a common urinary pathogen).40 The experimental results indicated that under NIR irradiation the localized overheating generated by GNShs led to the thermal decomposition and rupturing of the bacterial cell walls, suggesting an effective photothermal approach to combat catheter-related infections.
2.2 Photothermal bactericidal surfaces based on graphene derivatives
Graphene and its derivatives, such as graphene oxide (GO) and reduced graphene oxide (rGO), represent a typical family of two-dimensional nanostructured materials with unique morphological and physicochemical properties, which have attracted extensive attention since their discovery in 2004.81 In particular, with closely spaced energy levels of the loosely held π electrons, they have remarkable broad-band optical absorption ability and can generate heat when the light-excited electrons relax to the ground states.82 The high photothermal conversion efficiency, together with superior biocompatibility and easy functionality, make graphene and its derivatives suitable as PTAs for photothermal elimination of bacteria.27
GO and rGO have a large number of oxygen-containing groups and thus they have negative charges when dispersed in water.83 Therefore, they are suitable as building blocks to construct multilayered films with molecules having positive charges via the LBL technique.84 For example, an efficient and facile method was proposed for the fabrication of antibacterial coatings through alternative deposition of GO and poly(allylamine hydrochloride) (PAH) (Fig. 4a).41 The bacteria-killing mechanism of this composite coating system resulted from both the hyperthermia-induced protein denaturation and subsequent bacterial thermal decomposition, and the membrane stress is caused by the direct physical contact between the bacteria and the sharp edge of the GO tablet, leading to the membrane disturbance and irreversible damage of the cell wall. The high surface area of graphene derivatives is also beneficial for post-functionalization.85 For example, Yang and co-workers modified rGO basal planes with two different polyelectrolytes (poly(sodium 4-styrenesulfonate) (PSS) with negative charge and poly(diallyldimethylammonium chloride) (PDADMAC) with positive charge) via noncovalent adsorption, and then used these polyelectrolyte–rGO complexes to deposit an rGO-containing multilayered film on the quartz substrate (Fig. 4b).43 Under simulated solar irradiation, the multilayered film could produce sufficient local heat to kill 90% of airborne bacteria on contact within minutes. The photothermal bactericidal activity was maintained even when the film was shielded by pork tissue (3 mm in thickness), suggesting that the solar light in the NIR region with deep tissue penetration capability played a dominant role in the observed antibacterial activity.
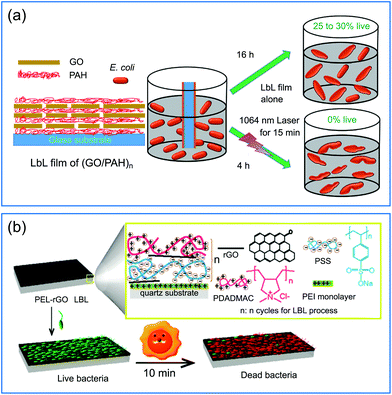 |
| Fig. 4 (a) Schematic illustration of the fabrication of a (GO/PAH)n multilayered film with antibacterial activity against E. coli under NIR irradiation. (b) Schematic illustration of a (PSS-rGO/PDADMAC-rGO)n multilayered film with photothermal bactericidal activity. Reproduced with permission from (a) ref. 41, copyright 2016, The Royal Society of Chemistry; (b) ref. 43, copyright 2015, American Chemical Society. | |
Besides as a building block for the fabrication of photothermal films, GO can also be employed as a component to develop functional membranes.86 For example, a new type of anti-biocontamination ultrafiltration membrane was prepared by the incorporation of GO flakes into bacterial nanocellulose (BNC) during its growth process.44 The BNC/GO composite membrane showed excellent bactericidal performance under NIR irradiation and good stability under different pH conditions and strong external force (mechanical agitation or ultrasound), and even under high pressure, providing a proof of concept for the design of efficient and environmentally friendly water purification membranes.
2.3 Photothermal bactericidal surfaces based on other PTAs
Apart from gold nanomaterials and graphene derivatives, there are other inorganic nanomaterials being used as effective PTAs for the fabrication of photothermal bactericidal surfaces.48,52 For example, tungsten bronze-type compounds (MxWO3, M = Cs, K, Na, etc.) have been recognized as promising candidates for photothermal elimination of bacteria because of their strong NIR absorption originating from LSPR.87 Using mussel-inspired chemistry, Park and co-workers immobilized Cs0.33WO3 nanoparticles on the surface of PDMS-based microreactors using poly(N-vinylpyrrolidone) with catechol groups as a spacer-layer, resulting in a NIR-mediated antibacterial continuous flow microreactor (Fig. 5).50 After being irradiated with 808 nm laser for 5 min, the system could kill more than 99.9% of E. coli and S. aureus. This antibacterial performance for both bacteria was well maintained after continuous operation for 30 days, indicating robust stability of the immobilized Cs0.33WO3 nanoparticles and their photothermal properties. In addition, the used Cs0.33WO3 nanoparticles could be easily released from the microchannels through acid treatment, facilitating the recycling of the used microreactors.
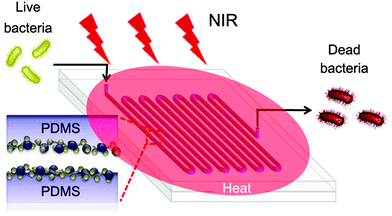 |
| Fig. 5 Schematic illustration of a NIR-mediated antibacterial continuous flow microreactor with immobilized Cs0.33WO3 nanoparticles. Reproduced with permission from ref. 50, copyright 2017, American Chemical Society. | |
Some organic materials that have light-to-heat conversion capability have also been exploited for their potential to fabricate photothermal bactericidal surfaces.57,88 For example, polycationic polymer polyaniline (PANI) with NIR absorption properties was assembled with catechol-conjugated poly(vinylpyrrolidone)sulfobetaine (PVPS) by electrostatic interactions to achieve a photothermal antibacterial coating (Fig. 6a).56 This composite coating exhibited an effective and rapid killing performance to destroy 99.9% of both Gram-negative and Gram-positive bacteria under NIR exposure for 3 min. Compared to the inorganic PTAs such as gold nanomaterials whose biodegradability was not clearly defined, the degradable PANI may have better feasibility for long-term biomedical applications.89 Polydopamine (PDA) is well-known as a mussel-inspired material, and has been extensively studied in surface chemistry. Besides having strong adhesive properties, good biocompatibility, and easy functionalization, PDA also has excellent photothermal conversion efficiency.90,91 Taking advantage of these properties, Ji and coworkers proposed a facile and effective method to fabricate photothermal bactericidal coatings by soaking the substrates in a newly prepared dopamine solution at room temperature for a period.55 After several minutes of NIR irradiation, the surface temperature of PDA coating increased rapidly, effectively killing S. aureus, E. coli, and C. albicans attached to the surface (Fig. 6b). Compared with the above mentioned methods, this method did not involve multistep procedures and could be applied onto diverse practical biomedical devices. Similar to PDA, the coordination complex of tannic acid and Fe3+ (TA/Fe) also has universal adherent properties and excellent photothermal conversion efficiency.92 Using TA/Fe complexes as PTAs and chitosan/silk fibroin as the scaffold, Zhang and co-workers fabricated a multifunctional cryogel for wound dressing applications (Fig. 6c).58 The highly porous spongy structure endowed the cryogel with strong hygroscopicity to absorb blood for rapid hemostasis. While under the illumination of NIR the incorporated TA/Fe complexes could efficiently eliminate bacteria at the wound sits and thus promote the wound healing process. Considering that all the components of this cryogel are naturally derived, it is beneficial for clinical applications as a “green” wound dressing material.
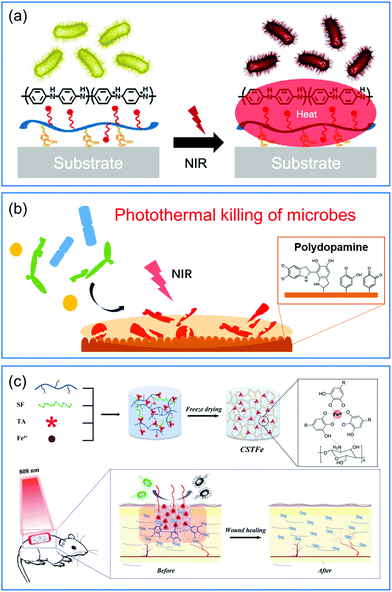 |
| Fig. 6 Schematic illustration of (a) a PVPS/PANI coating and (b) a PDA coating, and (c) a multifunctional cryogel containing TA/Fe complexes that can kill surface-attached bacteria under NIR irradiation. Reproduced with permission from (a) ref. 56, copyright 2015, American Chemical Society; (b) ref. 55, copyright 2016, Wiley-VCH; and (c) ref. 58, copyright 2019, Wiley-VCH. | |
3 Synergistic bactericidal surfaces based on the combination of APTT and other methods
Although APTT has been demonstrated to be effective in treating bacterial infections and eliminating matured biofilms, however, it should be noted that when APTT is used alone, the required irradiation intensity to fully eradicate bacteria is usually higher than the irradiance limit (0.32 W cm−2) according to the American National Standard Institute Laser Safety Standards.93 Moreover, under some circumstances, the local hyperthermia might lead to severe damage to adjacent normal cells and tissues.94 Therefore, it is desirable to combine APTT with other methods having different but complementary bactericidal mechanisms (such as traditional biocidal agents such as silver nanoparticles (AgNPs) and other light-based methods such as antibacterial photodynamic therapy (APDT)) together into one system to generate synergetic bactericidal surfaces95 (representative examples are listed in Table 2).
Table 2 Summary of photothermal bactericidal surfaces based on the combination of APTT and other antibacterial methodsa
PTA |
Biocide or PS |
Substrate |
Antibacterial mechanism |
Microorganism |
Ref. |
Abbreviations: PS, photosensitizer; PVDF, poly(vinylidene)fluoride; TNTs, TiO2 nanotube arrays; AgNPs, silver nanoparticles.
|
GNP |
AgNPs |
Glass |
APTT + Ag+ |
S. aureus, E. coli |
37
|
GNP |
Ag nanoplates |
Glass |
APTT + Ag+ |
S. aureus, E. coli |
96
|
Ag nanoplates |
Glass |
APTT + Ag+ |
S. aureus, E. coli |
97
|
GO |
AgNPs |
PES |
APTT + Ag+ |
S. aureus, E. coli |
42
|
MnO2 |
AgNPs |
Titanium |
APTT + Ag+ |
S. aureus, E. coli |
48
|
MoS2 |
Titanium |
APTT + APDT |
S. aureus, E. coli |
46
|
GO |
TiO2 |
PVDF |
APTT + APDT |
S. aureus, E. coli |
98
|
GNR |
Bi2WO6 |
Titanium |
APTT + APDT |
S. aureus, E. coli |
99
|
GNP |
TNTs |
Titanium |
APTT + APDT |
S. aureus, E. coli |
31
|
MoS2 |
IR780 |
Titanium |
APTT + APDT |
S. aureus
|
45
|
RP |
IR780 |
Titanium |
APTT + APDT |
S. aureus
|
53 and 54
|
PDA |
MoS2 |
Titanium |
APTT + APDT |
S. aureus
|
100
|
Red phosphorus |
Titanium |
APTT + APDT |
S. aureus, E. coli |
101
|
MoS2 |
Gentamicin |
Titanium |
APTT + antibiotic |
S. aureus, E. coli |
47
|
Bi2S3 |
Ag3PO4 |
Titanium |
APTT + APDT + Ag+ |
S. aureus, E. coli |
49
|
MoS2 |
AgNPs |
Titanium |
APTT + APDT + Ag+ |
S. aureus, E. coli |
102
|
3.1 Combination of APTT and AgNPs
AgNPs have been widely used as promising biocidal agents for combating pathologies previously treated with conventional antibiotics due to their enhanced broad-range antibacterial properties.103 However, traditional AgNP-based antimicrobial therapy still has some drawbacks such as (i) insufficient ability to eliminate matured biofilms because it is difficult for AgNPs to break through the protection of extracellular polysaccharides and to penetrate inside the biofilms and (ii) strong tendency to accumulate in tissues that released silver ions at high dose may cause local or systemic side effects including anaphylaxis and cytotoxicity.104 It is suggested that the combination of APTT and AgNPs may realize higher killing efficiency and lower cytotoxicity via a synergistic antibacterial mechanism that the localized heat generated from PTAs destroys bacterial membranes to facilitate the entrance of silver ions inside bacteria, reducing both the light intensity and dose of AgNPs.
Following the combinational design strategy, a multilayered antibacterial film was prepared which was composed of three different layers including a biocidal layer of AgNPs, a separation layer of SiO2, and a photothermal layer of GNSts from the outside to the inside.37 Each component maintained its intrinsic function, and the combination of localized hyperthermia generated by GNSts under NIR irradiation and the inherent bactericidal activity of the released silver ions endowed the multifunctional film with enhanced antibacterial performance. However, in this system, the AgNPs introduced during the preparation process tend to aggregate into clusters, resulting in the decrease of antibacterial activity. To solve this problem, instead of using individual AgNPs, Zhao and co-workers prepared new composite nanoagents as building blocks for the fabrication of synergistic bactericidal surfaces (Fig. 7).42 Here GO nanosheets with excellent photothermal properties were used as carriers for loading AgNPs to avoid their aggregation, and the resulting nanocomposites (named Ag@G) were further protected with sodium alginate sulfate (SAS) conjugated with dopamine to improve the hemocompatibility and cytocompatibility. Due to the negative charge from SAS, the obtained Ag@G-SAS could be co-deposited with a quaternized chitosan-coated GO nanosheet with positive charges on the poly(ether sulfone) membrane via the LBL technique. The modified membrane exhibited enhanced antibacterial activity and favorable biocompatibility at both cell and blood levels, showing great potential for applications in hemodialysis and tissue engineering.
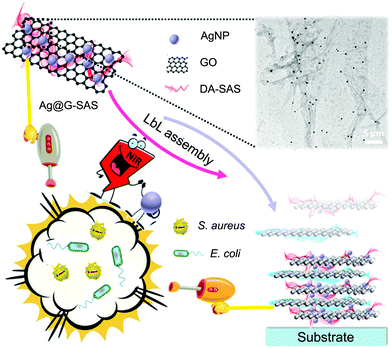 |
| Fig. 7 Schematic illustration of an antibacterial coating based on Ag@G with synergistic biocidal activity. Reproduced with permission from ref. 42, copyright 2018, American Chemical Society. | |
3.2 Combination of APTT and APDT
Besides APTT, APDT is also a promising light-based method for the treatment of localized bacterial infections by the reactive oxygen species (ROS) produced by photosensitizers (PSs) under harmless light irradiation of appropriate wavelength.105 It is a less invasive technique with less damage to healthy tissues and cells around the infected site.106,107 However, the efficacy of APDT is highly dependent on local oxygen levels, while the environment around the infection site is often hypoxic, which greatly limits the efficacy of APDT. It is found that a small amount of ROS could enhance the thermal susceptibility of bacteria to decrease the required temperature for elimination, while hyperthermia can disrupt the bacterial membrane to facilitate the permeation of ROS into bacteria.108 Therefore, combination of APTT and APDT provides an efficient and safe method to combat bacterial infection without compromising the viability of normal cells.
Based on the photodynamic and photothermal properties of MoS2, Wu and coworkers developed a series of synergetic bactericidal surfaces on titanium implant materials.45–47,102 For example, they prepared a chitosan-functionalized MoS2 hybrid coating through a simple electrophoretic deposition.46 It is found that either APDT sterilization under a single 660 nm visible light irradiation or APTT sterilization under a single 808 nm NIR light irradiation could only provide moderate antibacterial efficiency. However, when the coating was co-irradiated using two lights, a significant increase in the killing efficiency of up to 99.84% and 99.65% against E. coli and S. aureus, respectively, was observed. In the following work, in view of the need for dual-light irradiation for efficient sterilization, the NIR-activated photosensitizer IR780 was chosen. In order to address the problems of implant-related infections induced by bacterial biofilms, they fabricated a composite coating to eradicate the established biofilm in vivo in a short time with the help of NIR irradiation (Fig. 8).53 This coating is composed of multilayers with respective functions including the fibrous red phosphorus layer with photothermal ability, IR780 layer with photodynamic properties, PDA layer for further enhancement of the photothermal conversion efficiency, and arginine–glycine–aspartate–cysteine (RGDC) layer for the improvement of osteogenic cell adhesion and proliferation to accelerate bone tissue regeneration (from bottom to top). Based on the synergistic bactericidal mechanism, this composite film could eradicate the biofilm of S. aureus in vivo within a 10 min NIR irradiation. Moreover, the short-term irradiation did not lead to the damage of normal tissues around the implants.
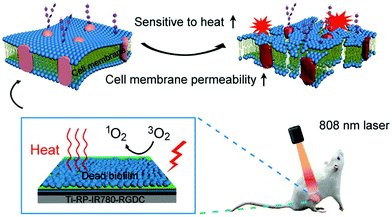 |
| Fig. 8 Schematic illustration of a red phosphorus/IR780 composite coating to eradicate S. aureus biofilm under NIR irradiation. Reproduced with permission from ref. 53, copyright 2018, Wiley-VCH. | |
Using two components to achieve the combined APTT and APDT under single NIR irradiation is usually associated with complicated fabrication processes; therefore, it is more convenient to use a single component with dual functions. It was found that semiconductor CuS nanoparticles could enhance the generation of ROS and also showed excellent photothermal performance in response to NIR light. Moreover, Cu ions could stimulate the proliferation and angiogenesis of cells to promote wound healing.109 Utilizing these properties, a hybrid thermoresponsive hydrogel embedded with CuS nanoparticles was developed to achieve rapid bacteria-killing and acceleration of wound healing at the same time.110 Under NIR irradiation for 10 min, the hybrid hydrogel generated hyperthermia and radical oxygen species as well as released copper ions; these work together to eliminate bacteria both in vitro and in vivo, with high killing efficiency.
4 Smart photothermal bactericidal surface with switchable functions
Similar to the most conventional bactericidal surfaces based on the contact-killing mechanism, one of the inherent disadvantages of the photothermal bactericidal surface is that the killed bacteria and other residues remain on the surface. The accumulation of dead bacteria causes a series of problems such as reducing the bactericidal effect due to the coverage of PTAs, providing nutrients for newly attached bacteria to induce secondary contamination, and triggering severe immune response or inflammation.111 Therefore, it is highly desired to remove the dead bacteria from the surface once they are killed, leaving a clean surface for long-term use. To this end, a smart “kill-and-release” antibacterial strategy was proposed and a series of smart antibacterial surfaces were developed with switchable surface function between killing bacteria and releasing bacteria.112–121 Interested readers may find detailed information about this promising area in several comprehensive reviews.95,122,123
In recent years, we applied this “kill-and-release” strategy and developed several smart photothermal bactericidal surfaces based on hybrid coating with both light-activated bactericidal properties and bacteria-releasing properties. The first example is a universal hybrid coating that could be prepared on various substrates by the sequential deposition of a gold nanoparticle layer (GNPL) and a phase-transitioned lysozyme film (PTLF), as shown in Fig. 9a.32 As mentioned in section 2.1.1, GNPL is composed of numerous GNP aggregates and thus exhibits an excellent photothermal effect.124–126 Moreover, the hierarchical micro-nanostructure of GNPL provides multiple contacting sites for bacteria attachment, which is beneficial for the efficient heat transfer. Therefore, under NIR irradiation for 5 min, a majority of the attached bacteria including MRSA were efficiently killed, and were then easily removed from the surface by subsequent treatment with vitamin C (Vc) solution to degrade the topmost PTLF (Fig. 9b). In particular, because the Vc-triggered degradation of PTLF followed a top-down sequence, it is possible to control the degradation extent by adjusting the concentration of Vc and the treatment time to realize the sequential degradation of PTLF for multiple uses. After three “kill-and-release” cycles, both the killing efficiency and release efficacy of the GNPL-PTLF coating were well maintained.
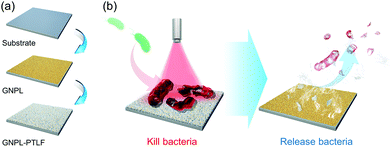 |
| Fig. 9 (a) Schematic illustration of the preparation procedure of GNPL-PTLF hybrid coating. (b) The killing and releasing performance of GNPL-PTLF coating. Reproduced with permission from ref. 32, copyright 2018, The Royal Society of Chemistry. | |
Although the use of Vc as a trigger to remove dead bacteria from the surface is effective, the introduction of extra chemicals may be disadvantageous and cumbersome in some certain biomedical applications. Alternatively, temperature, one of the most widely used stimuli to control the interaction between bacteria and surfaces, will be a better choice to actuate surface functionality.127 In the past decade, several smart antibacterial surfaces have been developed by incorporation of the typical temperature-responsive polymer poly(N-isopropylacrylamide) (PNIPAAm).128–131 In our recent work, we immobilized NH2-terminated PNIPAAm chains on the surface pre-deposited with a layer of the TA/Fe complex via chemical reactions between the NH2 groups and the phenolic hydroxyl groups of TA, achieving a temperature-responsive, photothermal antibacterial hybrid film (Fig. 10).59 The resulting film killed nearly 100% of the attached bacteria under a 5 min NIR irradiation, and more than 90% of the killed bacteria were then removed from the surface by a simple treatment with cold water (4 °C) due to the hydration and swelling of immobilized PNIPAAm chains. The antibacterial performance was not degraded after multiple “kill-and-release” cycles and the TA/Fe-PNIPAAm hybrid film was stable over several days under proper storage conditions. Considering the strong, substrate-independent adhesive properties of the TA/Fe complex,132 this hybrid film could be applied to most practical materials with different physicochemical properties, showing broad potential for various healthcare-related applications.
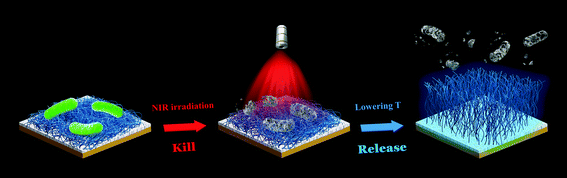 |
| Fig. 10 Schematic illustration of a smart hybrid antibacterial film with photothermal bactericidal activity and temperature-trigged bacteria-release ability. Reproduced with permission from ref. 59, copyright 2019, American Chemical Society. | |
5 Summary and perspective
The increasing resistance of bacteria to traditional biocidal agents has brought new challenges to the development of antibacterial surfaces. Fortunately, photothermal bactericidal surfaces based on hyperthermia provide a biocide-free and high-efficiency way to solve the problems related to surface-attached bacteria, in particular, the multidrug-resistant bacteria. In this review, the recent progress of photothermal bactericidal surfaces is summarized. According to the specific requirements of practical applications, various photothermal bactericidal surfaces have been developed by using proper fabrication methods to immobilize different PTAs on the substrates.
Although the remarkable achievements of photothermal bactericidal surfaces have been made, it should be noted that there are still some challenges related to this promising field and will be left as the future development directions including (i) exploiting PTAs with higher photothermal conversion efficiency and better biocompatibility to reduce the light intensity and possible cytotoxicity to normal cells and tissues; (ii) improving the stability of PTAs immobilized on the surfaces to maintain their performance for long-term use; (iii) integrating other functions such as osteogenesis and antithrombus into one system for specific practical applications; and (iv) taking the standards of simple, economic, and environmentally-friendly manufacturing methods into consideration for surface design to accelerate the practical process. Finally, the development of photothermal bactericidal surfaces is still at an early stage, and we hope that this review will attract more research groups to focus on this emerging field.
Conflicts of interest
There are no conflicts to declare.
Acknowledgements
This work was supported by the National Natural Science Foundation of China (21774086 and 21935008), the Natural Science Foundation of Jiangsu Province (BK20180093), the Suzhou Municipal Science and Technology Foundation (SYS2018026) and the Priority Academic Program Development of Jiangsu Higher Education Institutions (PAPD).
References
- L. Hall-Stoodley, J. W. Costerton and P. Stoodley, Nat. Rev. Microbiol., 2004, 2, 95–108 CrossRef CAS PubMed.
- D. Pavithra and M. Doble, Biomed. Mater., 2008, 3, 1–13 Search PubMed.
- B. Song, E. Zhang, X. Han, H. Zhu, Y. Shi and Z. Cao, ACS Appl. Mater. Interfaces, 2020, 12, 21330–21341 CrossRef CAS PubMed.
- D. Campoccia, L. Montanaro and C. R. Arciola, Biomaterials, 2013, 34, 8533–8554 CrossRef CAS PubMed.
- Z. K. Zander and M. L. Becker, ACS Macro Lett., 2017, 7, 16–25 CrossRef.
- M. Ghasemlou, F. Daver, E. P. Ivanova, J.-W. Rhim and B. Adhikari, ACS Appl. Mater. Interfaces, 2019, 11, 22897–22914 CrossRef CAS PubMed.
- T. B. Donadt and R. Yang, ACS Biomater. Sci. Eng., 2019, 6, 182–197 CrossRef.
- Q. Yu, Z. Wu and H. Chen, Acta Biomater., 2015, 16, 1–13 CrossRef CAS PubMed.
- A. Kugel, S. Stafslien and B. J. Chisholm, Prog. Org. Coat., 2011, 72, 222–252 CrossRef CAS.
- R. Kaur and S. Liu, Prog. Surf. Sci., 2016, 91, 136–153 CrossRef CAS.
- S. E. Rossiter, M. H. Fletcher and W. M. Wuest, Chem. Rev., 2017, 117, 12415–12474 CrossRef CAS PubMed.
- A. Muñoz-Bonilla and M. Fernández-García, Prog. Polym. Sci., 2012, 37, 281–339 CrossRef.
- Z. Y. Ong, N. Wiradharma and Y. Y. Yang, Adv. Drug Delivery Rev., 2014, 78, 28–45 CrossRef CAS PubMed.
- Y. Zheng, Y. Luo, K. Feng, W. Zhang and G. Chen, ACS Macro Lett., 2019, 8, 326–330 CrossRef CAS.
- Y. He, X. Wan, K. Xiao, W. Lin, J. Li, Z. Li, F. Luo, H. Tan, J. Li and Q. Fu, Biomater. Sci., 2019, 7, 5369–5382 RSC.
- J. Ahmad, X. Wen, F. Li and B. Wang, RSC Adv., 2019, 9, 6733–6744 RSC.
- S. Hernando-Amado, T. M. Coque, F. Baquero and J. L. Martinez, Nat. Microbiol., 2019, 4, 1432–1442 CrossRef CAS PubMed.
- T. P. Van Boeckel, J. Pires, R. Silvester, C. Zhao, J. Song, N. G. Criscuolo, M. Gilbert, S. Bonhoeffer and R. Laxminarayan, Science, 2019, 365, eaaw1944 CrossRef CAS PubMed.
- Z. Qi, P. Bharate, C. H. Lai, B. Ziem, C. Bottcher, A. Schulz, F. Beckert, B. Hatting, R. Mulhaupt, P. H. Seeberger and R. Haag, Nano Lett., 2015, 15, 6051–6057 CrossRef CAS PubMed.
- Y. Wang, Y. Jin, W. Chen, J. Wang, H. Chen, L. Sun, X. Li, J. Ji, Q. Yu and L. Shen, Chem. Eng. J., 2019, 358, 74–90 CrossRef CAS.
- J. W. Xu, K. Yao and Z. K. Xu, Nanoscale, 2019, 11, 8680–8691 RSC.
- D. Hu, H. Li, B. Wang, Z. Ye, W. Lei, F. Jia, Q. Jin, K.-F. Ren and J. Ji, ACS Nano, 2017, 11, 9330–9339 CrossRef CAS PubMed.
- X. Wang, C. Wang, X. Wang, Y. Wang, Q. Zhang and Y. Cheng, Chem. Mater., 2017, 29, 1370–1376 CrossRef CAS.
- K. Xiong, J. Li, L. Tan, Z. Cui, Z. Li, S. Wu, Y. Liang, S. Zhu and X. Liu, Colloid Interface Sci. Commun., 2019, 33, 100201 CrossRef CAS.
- Y. Yang, J. Aw and B. Xing, Nanoscale, 2017, 9, 3698–3718 RSC.
- H. Ji, H. Sun and X. Qu, Adv. Drug Delivery Rev., 2016, 105, 176–189 CrossRef CAS PubMed.
- K. Yang, L. Feng, X. Shi and Z. Liu, Chem. Soc. Rev., 2013, 42, 530–547 RSC.
- S. Szunerits and R. Boukherroub, J. Mater. Chem. B, 2016, 4, 6892–6912 RSC.
- Y. Feng, L. Liu, J. Zhang, H. Aslan and M. Dong, J. Mater. Chem. B, 2017, 5, 8631–8652 RSC.
- S. Cabana, C. S. Lecona-Vargas, H. I. Meléndez-Ortiz, A. Contreras-García, S. Barbosa, P. Taboada, B. Magariños, E. Bucio, A. Concheiro and C. Alvarez-Lorenzo, J. Drug Delivery Sci. Technol., 2017, 42, 245–254 CrossRef CAS.
- C. Jin, K. Su, L. Tan, X. Liu, Z. Cui, X. Yang, Z. Li, Y. Liang, S. Zhu, K. W. K. Yeung and S. Wu, Mater. Des., 2019, 177, 107845 CrossRef CAS.
- Y. Qu, T. Wei, J. Zhao, S. Jiang, P. Yang, Q. Yu and H. Chen, J. Mater. Chem. B, 2018, 6, 3946–3955 RSC.
- Y. Zhu, M. Ramasamy and D. K. Yi, ACS Appl. Mater. Interfaces, 2014, 6, 15078–15085 CrossRef CAS PubMed.
- T. Yang, D. Wang and X. Liu, Colloids Surf., B, 2019, 173, 833–841 CrossRef CAS PubMed.
- P. Pallavicini, A. Donà, A. Taglietti, P. Minzioni, M. Patrini, G. Dacarro, G. Chirico, L. Sironi, N. Bloise, L. Visai and L. Scarabelli, Chem. Commun., 2014, 50, 1969–1971 RSC.
- D. Rovati, B. Albini, P. Galinetto, P. Grisoli, B. Bassi, P. Pallavicini, G. Dacarro and A. Taglietti, Nanomaterials, 2019, 9, 1288 CrossRef CAS PubMed.
- P. Pallavicini, B. Bassi, G. Chirico, M. Collini, G. Dacarro, E. Fratini, P. Grisoli, M. Patrini, L. Sironi, A. Taglietti, M. Moritz, I. Sorzabal-Bellido, A. Susarrey-Arce, E. Latter, A. J. Beckett, I. A. Prior, R. Raval and Y. A. Diaz Fernandez, Sci. Rep., 2017, 7, 5259 CrossRef PubMed.
- M. Borzenkov, M. Moros, C. Tortiglione, S. Bertoldi, N. Contessi, S. Fare, A. Taglietti, A. D'Agostino, P. Pallavicini, M. Collini and G. Chirico, Beilstein J. Nanotechnol., 2018, 9, 2040–2048 CrossRef CAS PubMed.
- J. R. Ray, S. Tadepalli, S. Z. Nergiz, K.-K. Liu, L. You, Y. Tang, S. Singamaneni and Y.-S. Jun, ACS Appl. Mater. Interfaces, 2015, 7, 11117–11126 CrossRef CAS PubMed.
- O. Khantamat, C.-H. Li, F. Yu, A. C. Jamison, W.-C. Shih, C. Cai and T. R. Lee, ACS Appl. Mater. Interfaces, 2015, 7, 3981–3993 CrossRef CAS PubMed.
- R. Kurapati, M. Vaidyanathan and A. M. Raichur, RSC Adv., 2016, 6, 39852–39860 RSC.
- X. Fan, F. Yang, C. Nie, Y. Yang, H. Ji, C. He, C. Cheng and C. Zhao, ACS Appl. Mater. Interfaces, 2017, 10, 296–307 CrossRef PubMed.
- L. Hui, J. T. Auletta, Z. Huang, X. Chen, F. Xia, S. Yang, H. Liu and L. Yang, ACS Appl. Mater. Interfaces, 2015, 7, 10511–10517 CrossRef CAS PubMed.
- Q. Jiang, D. Ghim, S. Cao, S. Tadepalli, K.-K. Liu, H. Kwon, J. Luan, Y. Min, Y.-S. Jun and S. Singamaneni, Environ. Sci. Technol., 2018, 53, 412–421 CrossRef PubMed.
- M. Li, L. Li, K. Su, X. Liu, T. Zhang, Y. Liang, D. Jing, X. Yang, D. Zheng, Z. Cui, Z. Li, S. Zhu, K. W. K. Yeung, Y. Zheng, X. Wang and S. Wu, Adv. Sci., 2019, 6, 1900599 CrossRef PubMed.
- Z. Feng, X. Liu, L. Tan, Z. Cui, X. Yang, Z. Li, Y. Zheng, K. W. K. Yeung and S. Wu, Small, 2018, 14, 1704347 CrossRef PubMed.
- M. Ma, X. Liu, L. Tan, Z. Cui, X. Yang, Y. Liang, Z. Li, Y. Zheng, K. W. K. Yeung and S. Wu, Biomater. Sci., 2019, 7, 1437–1447 RSC.
- X. Wang, K. Su, L. Tan, X. Liu, Z. Cui, D. Jing, X. Yang, Y. Liang, Z. Li, S. Zhu, K. W. K. Yeung, D. Zheng and S. Wu, ACS Appl. Mater. Interfaces, 2019, 11, 15014–15027 CrossRef CAS PubMed.
- L. Hong, X. Liu, L. Tan, Z. Cui, X. Yang, Y. Liang, Z. Li, S. Zhu, Y. Zheng, K. W. K. Yeung, D. Jing, D. Zheng, X. Wang and S. Wu, Adv. Healthcare Mater., 2019, 8, e1900835 CrossRef PubMed.
- Y. K. Kim, E. B. Kang, S. M. Kim, C. P. Park, I. In and S. Y. Park, ACS Appl. Mater. Interfaces, 2017, 9, 3192–3200 CrossRef CAS PubMed.
- C. Song, T. Li, W. Guo, Y. Gao, C. Yang, Q. Zhang, D. An, W. Huang, M. Yan and C. Guo, New J. Chem., 2018, 42, 3175–3179 RSC.
- J. Li, X. Liu, L. Tan, Y. Liang, Z. Cui, X. Yang, S. Zhu, Z. Li, Y. Zheng, K. W. K. Yeung, X. Wang and S. Wu, Small Methods, 2019, 3, 1900048 CrossRef.
- L. Tan, J. Li, X. Liu, Z. Cui, X. Yang, S. Zhu, Z. Li, X. Yuan, Y. Zheng, K. W. K. Yeung, H. Pan, X. Wang and S. Wu, Adv. Mater., 2018, 30, 1801808 CrossRef PubMed.
- B. Huang, L. Tan, X. Liu, J. Li and S. Wu, Bioact. Mater., 2019, 4, 17–21 CrossRef PubMed.
- W. Lei, K. Ren, T. Chen, X. Chen, B. Li, H. Chang and J. Ji, Adv. Mater. Interfaces, 2016, 3, 1600767 CrossRef.
- S. H. Kim, E. B. Kang, C. J. Jeong, S. M. Sharker, I. In and S. Y. Park, ACS Appl. Mater. Interfaces, 2015, 7, 15600–15606 CrossRef CAS PubMed.
- Y. Ko, J. Kim, H. Y. Jeong, G. Kwon, D. Kim, M. Ku, J. Yang, Y. Yamauchi, H. Y. Kim, C. Lee and J. You, Carbohydr. Polym., 2019, 203, 26–34 CrossRef CAS PubMed.
- Y. Yu, P. Li, C. Zhu, N. Ning, S. Zhang and G. J. Vancso, Adv. Funct. Mater., 2019, 29, 1904402 CrossRef.
- Y. Wang, T. Wei, Y. Qu, Y. Zhou, Y. Zheng, C. Huang, Y. Zhang, Q. Yu and H. Chen, ACS Appl. Mater. Interfaces, 2020, 12, 21283–21291 CrossRef CAS PubMed.
- Z. Qin and J. C. Bischof, Chem. Soc. Rev., 2012, 41, 1191–1217 RSC.
- S. Eustis and M. A. el-Sayed, Chem. Soc. Rev., 2006, 35, 209–217 RSC.
- X. Huang and M. A. El-Sayed, J. Adv. Res., 2010, 1, 13–28 CrossRef.
- S. Kang, S. H. Bhang, S. Hwang, J. K. Yoon, J. Song, H. K. Jang, S. Kim and B. S. Kim, ACS Nano, 2015, 9, 9678–9690 CrossRef CAS PubMed.
- M. Malki, S. Fleischer, A. Shapira and T. Dvir, Nano Lett., 2018, 18, 4069–4073 CrossRef CAS PubMed.
- M. Li, J. W. Kang, R. R. Dasari and I. Barman, Angew. Chem., Int. Ed., 2014, 53, 14115–14119 CrossRef CAS PubMed.
- Z. Wang, S. Li, M. Zhang, Y. Ma, Y. Liu, W. Gao, J. Zhang and Y. Gu, Adv. Sci., 2017, 4, 1600327 CrossRef PubMed.
- L. Cheng, C. Wang, L. Feng, K. Yang and Z. Liu, Chem. Rev., 2014, 114, 10869–10939 CrossRef CAS PubMed.
- G. von Maltzahn, J.-H. Park, A. Agrawal, N. K. Bandaru, S. K. Das, M. J. Sailor and S. N. Bhatia, Cancer Res., 2009, 69, 3892–3900 CrossRef CAS PubMed.
- X. Huang, I. H. El-Sayed, W. Qian and M. A. El-Sayed, J. Am. Chem. Soc., 2006, 128, 2115–2120 CrossRef CAS PubMed.
- Y. Zheng, Y. Wu, Y. Zhou, J. Wu, X. Wang, Y. Qu, Y. Wang, Y. Zhang and Q. Yu, ACS Appl. Mater. Interfaces, 2020, 12, 7905–7914 CrossRef CAS PubMed.
- Y.-Q. Zhao, Y. Sun, Y. Zhang, X. Ding, N. Zhao, B. Yu, H. Zhao, S. Duan and F.-J. Xu, ACS Nano, 2020, 14, 2265–2275 CrossRef CAS PubMed.
- Y. Fang, W. S. Chang, B. Willingham, P. Swanglap, S. Dominguez-Medina and S. Link, ACS Nano, 2012, 6, 7177–7184 CrossRef CAS.
- J. L. Li, D. Day and M. Gu, Adv. Mater., 2008, 20, 3866–3871 CrossRef CAS.
- M. A. Abdou Mohamed, V. Raeesi, P. V. Turner, A. Rebbapragada, K. Banks and W. C. W. Chan, Biomaterials, 2016, 97, 154–163 CrossRef CAS PubMed.
- J. W. Lee, H. Jung, H. H. Cho, J. H. Lee and Y. Nam, Biomaterials, 2018, 153, 59–69 CrossRef CAS PubMed.
- X. Li, L. Xing, K. Zheng, P. Wei, L. Du, M. Shen and X. Shi, ACS Appl. Mater. Interfaces, 2017, 9, 5817–5827 CrossRef CAS PubMed.
- Y. Pei, Z. Wang, S. Zong and Y. Cui, J. Mater. Chem. B, 2013, 1, 3992–3998 RSC.
- A. M. Gobin, M. H. Lee, N. J. Halas, W. D. James, R. A. Drezek and J. L. West, Nano Lett., 2007, 7, 1929–1934 CrossRef CAS PubMed.
- S. C. Gad, K. L. Sharp, C. Montgomery, J. D. Payne and G. P. Goodrich, Int. J. Toxicol., 2012, 31, 584–594 CrossRef CAS PubMed.
- D. G. Maki, D. M. Kluger and C. J. Crnich, Mayo Clin. Proc., 2006, 81, 1159–1171 CrossRef PubMed.
- K. S. Novoselov, A. K. Geim, S. V. Morozov, D. Jiang, Y. Zhang, S. V. Dubonos, I. V. Grigorieva and A. A. Firsov, Science, 2004, 306, 666–669 CrossRef CAS PubMed.
- G. Liu, J. Xu and K. Wang, Nano Energy, 2017, 41, 269–284 CrossRef CAS.
- S. Park and R. S. Ruoff, Nat. Nanotechnol., 2009, 4, 217–224 CrossRef CAS PubMed.
- F. Wu, J. Li, Y. Su, J. Wang, W. Yang, N. Li, L. Chen, S. Chen, R. Chen and L. Bao, Nano Lett., 2016, 16, 5488–5494 CrossRef CAS PubMed.
- L. Zhang, J. Xia, Q. Zhao, L. Liu and Z. Zhang, Small, 2010, 6, 537–544 CrossRef CAS PubMed.
- S. P. Singh, Y. Li, J. Zhang, J. M. Tour and C. J. Arnusch, ACS Nano, 2018, 12, 289–297 CrossRef CAS.
- K. Manthiram and A. P. Alivisatos, J. Am. Chem. Soc., 2012, 134, 3995–3998 CrossRef CAS PubMed.
- J. Song, H. Liu, M. Lei, H. Tan, Z. Chen, A. Antoshin, G. F. Payne, X. Qu and C. Liu, ACS Appl. Mater. Interfaces, 2020, 12, 8915–8928 CrossRef CAS PubMed.
- M. Shahadat, M. Z. Khan, P. F. Rupani, A. Embrandiri, S. Sultana, S.
Z. Ahammad, S. Wazed Ali and T. R. Sreekrishnan, Adv. Colloid Interface Sci., 2017, 249, 2–16 CrossRef CAS PubMed.
- X. Zeng, M. Luo, G. Liu, X. Wang, W. Tao, Y. Lin, X. Ji, L. Nie and L. Mei, Adv. Sci., 2018, 5, 1800510 CrossRef PubMed.
- J. Wu, Y. Zheng, S. Jiang, Y. Qu, T. Wei, W. Zhan, L. Wang, Q. Yu and H. Chen, ACS Appl. Mater. Interfaces, 2019, 11, 12357–12366 CrossRef CAS PubMed.
- P. Y. Liu, Z. H. Miao, K. Li, H. Yang, L. Zhen and C. Y. Xu, Colloids Surf., B, 2018, 167, 183–190 CrossRef CAS PubMed.
-
American National Standards Institute, ANSI Z136.1-2000: American National Standard for Safe Use of Lasers, Laser Institute of America, Orlando, FL, 2000 Search PubMed.
- X. Zhu, W. Feng, J. Chang, Y.-W. Tan, J. Li, M. Chen, Y. Sun and F. Li, Nat. Commun., 2016, 7, 10437 CrossRef CAS.
- T. Wei, Q. Yu and H. Chen, Adv. Healthcare Mater., 2019, 8, 1801381 CrossRef CAS PubMed.
- A. D'Agostino, A. Taglietti, R. Desando, M. Bini, M. Patrini, G. Dacarro, L. Cucca, P. Pallavicini and P. Grisoli, Nanomaterials, 2017, 7, 7 CrossRef PubMed.
- A. D'Agostino, A. Taglietti, P. Grisoli, G. Dacarro, L. Cucca, M. Patrini and P. Pallavicini, RSC Adv., 2016, 6, 70414–70423 RSC.
- J. Sun, L. Song, Y. Fan, L. Tian, S. Luan, S. Niu, L. Ren, W. Ming and J. Zhao, ACS Appl. Mater. Interfaces, 2019, 11, 26581–26589 CrossRef CAS PubMed.
- B. Li, L. Tan, X. Liu, Z. Li, Z. Cui, Y. Liang, S. Zhu, X. Yang, K. W. Kwok Yeung and S. Wu, J. Hazard. Mater., 2019, 380, 120818 CrossRef CAS PubMed.
- Z. Yuan, B. Tao, Y. He, J. Liu, C. Lin, X. Shen, Y. Ding, Y. Yu, C. Mu, P. Liu and K. Cai, Biomaterials, 2019, 217, 119290 CrossRef CAS PubMed.
- Q. Zhang, X. Liu, L. Tan, Z. Cui, Z. Li, Y. Liang, S. Zhu, K. W. K. Yeung, Y. Zheng and S. Wu, Chem. Eng. J., 2020, 383, 123088 CrossRef.
- M. Zhu, X. Liu, L. Tan, Z. Cui, Y. Liang, Z. Li, K. W. Kwok Yeung and S. Wu, J. Hazard. Mater., 2020, 383, 121122 CrossRef CAS PubMed.
- P. Pallavicini, G. Dacarro and A. Taglietti, Eur. J. Inorg. Chem., 2018, 2018, 4846–4855 CrossRef CAS.
- L. Rizzello and P. P. Pompa, Chem. Soc. Rev., 2014, 43, 1501–1518 RSC.
- X. Li, D. Lee, J. D. Huang and J. Yoon, Angew. Chem., Int. Ed., 2018, 57, 9885–9890 CrossRef CAS.
- D. W. Felsher, Nat. Rev. Cancer, 2003, 3, 375–380 CrossRef CAS PubMed.
- W. Tong, Y. Xiong, S. Duan, X. Ding and F. J. Xu, Biomater. Sci., 2019, 7, 1905–1918 RSC.
- M. Yin, Z. Li, E. Ju, Z. Wang, K. Dong, J. Ren and X. Qu, Chem. Commun., 2014, 50, 10488–10490 RSC.
- B. Tao, C. Lin, Y. Deng, Z. Yuan, X. Shen, M. Chen, Y. He, Z. Peng, Y. Hu and K. Cai, J. Mater. Chem. B, 2019, 7, 2534–2548 RSC.
- M. Li, X. Liu, L. Tan, Z. Cui, X. Yang, Z. Li, Y. Zheng, K. W. K. Yeung, P. K. Chu and S. Wu, Biomater. Sci., 2018, 6, 2110–2121 RSC.
- L. Mi and S. Jiang, Angew. Chem., Int. Ed., 2014, 53, 1746–1754 CrossRef CAS PubMed.
- Z. Cao, L. Mi, J. Mendiola, J. R. Ella-Menye, L. Zhang, H. Xue and S. Jiang, Angew. Chem., Int. Ed., 2012, 51, 2602–2605 CrossRef CAS.
- T. Wei, Q. Yu, W. Zhan and H. Chen, Adv. Funct. Mater., 2016, 5, 449–456 CAS.
- T. Wei, W. Zhan, L. Cao, C. Hu, Y. Qu, Q. Yu and H. Chen, ACS Appl. Mater. Interfaces, 2016, 8, 30048–30057 CrossRef CAS PubMed.
- B. Wang, Z. Ye, Q. Xu, H. Liu, Q. Lin, H. Chen and K. Nan, Biomater. Sci., 2016, 4, 1731–1741 RSC.
- T. Wei, W. Zhan, Q. Yu and H. Chen, ACS Appl. Mater. Interfaces, 2017, 9, 25767–25774 CrossRef CAS PubMed.
- D. Zhang, Y. Fu, L. Huang, Y. Zhang, B. Ren, M. Zhong, J. Yang and J. Zheng, J. Mater. Chem. B, 2018, 6, 950–960 RSC.
- Y. Qu, T. Wei, W. Zhan, C. Hu, L. Cao, Q. Yu and H. Chen, J. Mater. Chem. B, 2017, 5, 444–453 RSC.
- Y. Zhou, Y. Zheng, T. Wei, Y. Qu, Y. Wang, W. Zhan, Y. Zhang, G. Pan, D. Li, Q. Yu and H. Chen, ACS Appl. Mater. Interfaces, 2020, 12, 5447–5455 CrossRef CAS PubMed.
- W. Zhan, T. Wei, L. Cao, C. Hu, Y. Qu, Q. Yu and H. Chen, ACS Appl. Mater. Interfaces, 2017, 9, 3505–3513 CrossRef CAS PubMed.
- W. Zhan, Y. Qu, T. Wei, C. Hu, Y. Pan, Q. Yu and H. Chen, ACS Appl. Mater. Interfaces, 2018, 10, 10647–10655 CrossRef CAS.
- T. Wei, Z. Tang, Q. Yu and H. Chen, ACS Appl. Mater. Interfaces, 2017, 9, 37511–37523 CrossRef CAS PubMed.
- Q. Yu and H. Chen, Acta Polym. Sin., 2020, 51, 319–325 Search PubMed.
- Z. Lyu, F. Zhou, Q. Liu, H. Xue, Q. Yu and H. Chen, Adv. Funct. Mater., 2016, 26, 5787–5795 CrossRef CAS.
- J. Wu, Y. Qu, Q. Yu and H. Chen, Mater. Chem. Front., 2018, 2, 2175–2190 RSC.
- J. Wu, H. Xue, Z. Lyu, Z. Li, Y. Qu, Y. Xu, L. Wang, Q. Yu and H. Chen, ACS Appl. Mater. Interfaces, 2017, 9, 21593–21598 CrossRef CAS.
- B. S. Gomes, B. Simões and P. M. Mendes, Nat. Rev. Chem., 2018, 2, 1–15 CrossRef.
- Q. Yu, P. Shivapooja, L. M. Johnson, G. Tizazu, G. J. Leggett and G. P. López, Nanoscale, 2013, 5, 3632–3637 RSC.
- Q. Yu, J. Cho, P. Shivapooja, L. K. Ista and G. P. López, ACS Appl. Mater. Interfaces, 2013, 5, 9295–9304 CrossRef CAS PubMed.
- Q. Yu, L. K. Ista and G. P. López, Nanoscale, 2014, 6, 4750–4757 RSC.
- L. K. Ista, Q. Yu, A. Parthasarathy, K. S. Schanze and G. P. López, Biointerphases, 2016, 11, 019003 CrossRef PubMed.
- H. Ejima, J. J. Richardson and F. Caruso, Nano Today, 2017, 12, 136–148 CrossRef CAS.
|
This journal is © The Royal Society of Chemistry 2021 |
Click here to see how this site uses Cookies. View our privacy policy here.