DOI:
10.1039/D0AY02121K
(Paper)
Anal. Methods, 2021,
13, 636-641
Analysis of pyrethroids in cereals by HPLC with a deep eutectic solvent-based dispersive liquid–liquid microextraction with solidification of floating organic droplets†
Received
16th November 2020
, Accepted 29th December 2020
First published on 30th December 2020
Abstract
This work presents a novel and green analytical procedure involving a deep eutectic solvent-based dispersive liquid–liquid microextraction with solidification of floating organic droplets (DES-DLLME-SFOD) followed by HPLC to measure three pyrethroids (bifenthrin, β-cypermethrin, and deltamethrin) in cereal samples. Firstly, a low-density hydrophobic DES was synthesized from thymol and octanoic acid in the molar ratio of 1/4 and this was applied as a green extraction solvent in the DLLME procedure to avoid the use of a toxic extractant. After centrifugation and placing it on an ice bath, it is transformed into a solid phase on the top of the sample solution to reduce the loss of extractant, conducive to convenient collection thereafter. This procedure required the optimal conditions (including the type, proportion, and amount of DES as the extractant, the volume of the dispersant acetonitrile, the amount of salt, and the pH value) to be evaluated. Under optimized variates, the proposed method provided good linearity with a correlation coefficient greater than 0.997 and limits of quantification within the range of 6.6–8.9 μg kg−1. The recoveries of pyrethroids in corn, wheat, barley, and oats were 75.6–87.2%, and the relative standard deviation was less than 3.6%. The method, therefore, offers a green, efficient, and convenient approach for the determination of pesticides in cereals.
1 Introduction
Cereals are the foundation of the human diet and they are a major source of macro and micronutrients essential to human life. A cereal-related diet could reduce the risk of chronic diseases, including cancer, gastrointestinal disorders, and cardiovascular diseases.1 To improve the productivity of cereals, farmers prefer to use pesticides during planting. Nowadays, pyrethroids that are easy to degrade and have low toxicity are widely applied in the field.2 In China, it is reported that more than 3700 tons of pyrethroid pesticides are used for pest control in agriculture, forestry, horticulture, and industry every year;3 however, unreasonable use has resulted in excessive pesticide residues in harvested cereals,4 posing a threat to consumer health, including the suppression of the immune system and damage to the nervous system.5 Long-term consumption of cereals with pyrethroid residues by consumers can result in chronic poisoning. Many international organizations limit the number of pyrethroids in cereals by setting maximum residue limits (MRLs).6 Consequently, a fast and effective approach to analyze pyrethroid pesticide residues in cereals to ensure food safety is essential.7
In recent years, liquid–liquid extraction (LLE)8 and solid-phase extraction (SPE)9 have been the most prevalent procedures for pre-treatment of pesticides in food samples; however, a large amount of toxic organic solvent and time are involved in SPE and LLE methods.
Dispersive liquid–liquid microextraction (DLLME) is a simple, rapid, low-cost, and high enrichment factor sample pre-treatment technique, which has attracted much research attention.10 Meanwhile, the extraction solvent applied in this method is a halogenated organic solvent. It is potentially harmful to the operator and the environment, therefore, choosing a green extraction solvent is particularly important.
New developments in the extraction technology are influenced by the concepts of green, sustainable solvents.11,12 The extraction solvent, therefore, should be effective, cheap, readily available, and environmentally friendly.13,14 A deep eutectic solvent (DES), as a class of green solvents, is a eutectic mixture of more than two components composed of a tested stoichiometric ratio of a hydrogen bond acceptor (e.g. quaternary ammonium salt) and a hydrogen bond donor (e.g. amides, carboxylic acids, polyols, etc.).15 DESs exhibit characteristics akin to those of ionic liquids; these include non-flammability, low vapor pressure, low toxicity, and the ability to be recycled.16 However, DESs have the advantages of low cost, easy preparation, green credentials, and ready biodegradability, which to some extent reduce damage to the environment.17 Thus, they are a good alternative to the traditional toxic or inefficient extraction solvents.18 Most of the DESs studied at present are hydrophilic and easily destroyed in water due to their hydrogen bonding.19 The development of a hydrophobic DES will greatly expand the utilization range of DESs.
Another improvement in the DLLME technique was the incorporation of the solidification of a floating organic drop (DLLME-SFOD).20 In this procedure, micro-drops of extraction solvent with low density and melting point close to room temperature can be centrifuged, and the drops migrate to the top of the sample: the extraction solvent is converted into the solid phase at low temperature in an ice bath for ease of collection.21 The benefits of the approach are ease of operation, rapidity, and low utilization of organic solvent;22 however, there are a limited range of extraction solvents that can be used for solidification. In the present work, a hydrophobic eutectic solvent synthesized from thymol–octanoic acid was used as an extraction solvent because it is eco-friendly and its freezing point is close to room temperature.
In this work, green and efficient hydrophobic DESs were chosen as the extraction solvent of DLLME, based on solidification for collection, and coupled with HPLC for the analysis of pyrethroids in cereals.
2 Materials and methods
2.1 Instruments and reagents
The Agilent 1260 HPLC and the Eclipse Plus C18 chromatographic column (250 × 4.6 mm, 5 μm) were obtained from Agilent Technologies (Beijing, China). The mobile phase was acetonitrile and water (volume fractions: 95% and 5%, respectively), with a flow rate of 0.5 mL min−1, and an injection volume of 20 μL. The detector is a diode array detector with a detection wavelength of 220 nm. The retention times recorded for bifenthrin, β-cypermethrin, and deltamethrin were 9.4, 10.3, and 14.7 min, respectively (Fig. S1†).
The standard products of bifenthrin, β-cypermethrin, and deltamethrin (all 98%) were sourced from the Institute for Control of Agrochemicals, Ministry of Agriculture (Beijing, China). Thymol, octanoic acid, nonanoic acid, decanoic acid, sodium chloride, methanol, and acetonitrile were obtained from Aladdin Biochemical Technology (Shanghai, China). Corn, wheat, barley, and oats were harvested in the experimental field at Shanxi Agricultural University (Taigu, China).
2.2 Synthesis of DESs
This procedure was carried out by mixing 1 mmol of thymol as a hydrogen bond acceptor (HBA) with 4 mmol of octanoic acid as a hydrogen bond donor (HBD) in a 10 mL glass tube. The glass tube was fixed in a thermostatic water bath to maintain the temperature at 70 °C for 10 min to form a colorless, homogeneous phase DES. After cooling, it was stored at room temperature.
2.3 Preparation of sample solution
Cereals were crushed using a pulverizer and 1 g of sample was placed in a 10 mL centrifuge tube, and 1.5 mL of acetonitrile was added for vortex-assisted extraction at 3500 rpm for 5 min. The supernatant was removed as the sample solution for the DES-DLLME-SFOD procedure (Fig. 1).
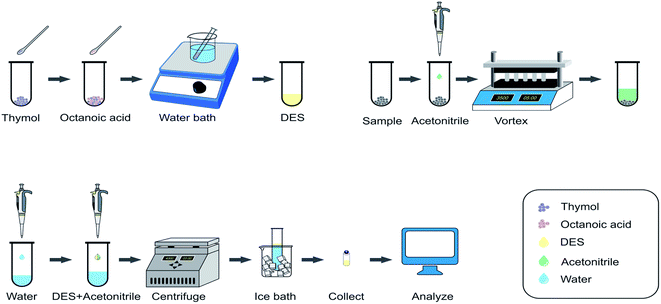 |
| Fig. 1 Schematic illustration of the DES-DLLME-SFOD procedure. | |
2.4 DES-DLLME-SFOD procedure
After mixing 0.8 mL of sample solution as the dispersant with 60 μL of DES as the extraction solvent, the mixture was injected into a 10 mL centrifuge tube including 5 mL of water. The dispersive liquid–liquid microextraction (DLLME) process was completed and then centrifugation was carried out at 4000 rpm for 3 min. The fine droplets dispersed by the extraction phase floated on the surface of the tube. After that, the centrifuge tube was placed in an ice bath for 15 min and the droplets were collected after the extracted phase had solidified. After it had melted to liquid at room temperature, HPLC was used for the determination of analytes (Fig. 1).
3 Results and discussion
3.1 Optimization of the DES-DLLME-SFOD procedure
To acquire the best conditions for DLLME, a series of optimizations were conducted to assess the influences of several parameters on the extraction efficiency, regarding the type of DES, the molar ratio between the hydrogen bond acceptor and hydrogen bond donor, the volume of DES, the volume of acetonitrile, amount of salt, and pH of the solution. The experimental parameters were optimized using the sample containing 2.5 mg kg−1 of each analyte and triplicate tests were undertaken.
3.1.1 Effect of DES type.
In the process of DLLME, a precise DES as an extraction solvent is significant to the extraction efficiency.23 Some properties of DES such as insolubility, low density, a low freezing point, and low toxicity, have been considered.24 In this study, thymol was mixed with octanoic, nonanoic, and decanoic acids to form three DESs. Other invariant conditions were set as follows: 60 μL of DES synthesized using thymol and fatty acid in the molar ratio of 1/4 and 0.8 mL of acetonitrile. Broadening of the O–H stretching vibration band of thymol (3188 cm−1) and shifting of the C
O stretching vibration band of fatty acids (1700 cm−1) were observed, indicating the formation of DES via hydrogen bonding (Fig. S2†).25,26 As shown in Fig. S3,† there is no significant difference in the extraction efficiency of each extraction solvent towards the three analytes. The solidification time of thymol–octanoic acid is relatively short in these three DESs. Thus, thymol–octanoic acid was chosen to be used as the extraction solvent.
3.1.2 Effect of the molar ratio of HBA to HBD.
To obtain a DES with a high extraction efficiency, the molar ratio of its constituent compounds was considered. This is because different molar ratios are important factors affecting the dissolution and extraction recovery of analytes from a food matrix.27 In this work, thymol–octanoic acids with different molar ratios (1/5, 1/4, 1/3, 1/2, 1/1, 2/1, and 3/1) were prepared. Other invariant conditions were set as follows: 60 μL of DES synthesized using thymol and octanoic acid and 0.8 mL of acetonitrile. Results are shown in Fig. S4† and the extraction efficiency was high when the molar ratio was 1/4. The different molar ratios are important in terms of their effects on the characteristics (density and viscosity) of the DES, the interactions between DES and analytes, and therefore the dissolution and extraction recovery of analytes.28–30 Therefore, the optimal ratio of thymol to octanoic acid was deemed to be 1/4.
3.1.3 Effect of DES volume.
The volume of DES is another critical factor that affects the extraction efficiency of pyrethroids, and further affects the quantification and detection limit of the method.31 A series of volumes of DES in the range of 40 to 100 μL were investigated. Other invariant conditions were set as follows: DES synthesized using thymol and octanoic acid in the molar ratio of 1/4 and 0.8 mL of acetonitrile. As shown in Fig. 2, as the volume of DES increased from 40 μL to 60 μL, the recovery of pyrethroid pesticides increased and the extraction efficiency gradually increased. Volumes of DES exceeding 60 μL did not lead to a significant increase, therefore, 60 μL of DES was used as the extraction solvent volume to achieve a high extraction efficiency.
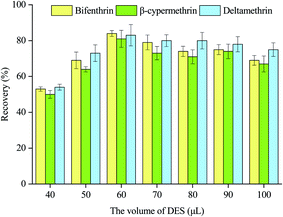 |
| Fig. 2 Effect of DES volume. | |
3.1.4 Effect of acetonitrile volume.
The volume of the dispersant is also an essential factor affecting extraction efficiency. The amount of acetonitrile used was changed in the range of 0.4 mL to 1.0 mL. Other invariant conditions were set as follows: 60 μL of DES synthesized using thymol and octanoic acid in the molar ratio of 1/4. As shown in Fig. 3, when the volume of acetonitrile was increased from 0.4 mL to 0.8 mL, the extraction efficiency generally increased because the increasing acetonitrile content improves the dispersing effect and the extraction efficiency increases; however, when the amount of acetonitrile was increased from 0.8 mL to 1.0 mL, the solubility of the compounds in acetonitrile aqueous solution was enhanced due to the excessive use of acetonitrile, rendering extraction more difficult, so the extraction efficiency decreased.10 A higher volume of acetonitrile led to a decreased extraction efficiency of pyrethroids due to a decrease in the distribution coefficient.32 In further experiments, 0.8 mL of acetonitrile was selected as the optimal dispersant.
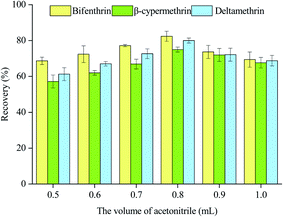 |
| Fig. 3 Effect of acetonitrile volume. | |
3.1.5 Effect of salt addition.
The addition of salt in the water phase changes the ionic strength and may increase the recovery of compounds from the sample.33 The effects of different salt contents on recovery were studied. Fig. S5† demonstrates that the recovery gradually decreased with the increase of sodium chloride dose in the range of 0 g to 1.5 g. Other invariant conditions were set as follows: 60 μL of DES was synthesized using thymol and octanoic acid in the molar ratio of 1/4 and 0.8 mL of acetonitrile. This may be because chlorine ions in the solution form hydrogen bonds with hydrogen bond donors, which may destroy the extraction solvent (thymol–octanoic acid) and lead to a lower extraction recovery,19 therefore, subsequent analyses were undertaken without salt.
3.1.6 Effect of pH value.
The pH value of the solution affects the ionization of the analytes and the distribution coefficient of the analytes between two phases, ultimately affecting the extraction efficiency.2 The pH value of the water was changed from 5 to 9 with 0.1 mol L−1 HCl and 0.1 mol L−1 NaOH before DLLME. Other invariant conditions were set as follows: 60 μL of DES synthesized using thymol and octanoic acid in the molar ratio of 1/4 and 0.8 mL of acetonitrile. Fig. S6† shows that the pH value has no significant effect on the recovery of analytes. This may be because the detected molecular state of pyrethroids and the phase behavior of DLLME are both pH-insensitive.10 The pH value did not, therefore, need to be adjusted in subsequent experiments.
3.2 Analysis and verification of the method
The analytical parameters of this method were explored in terms of linear range, intra-day and inter-day relative standard deviations (RSDs), correlation coefficient (R), the limit of detection (LOD), and limit of quantification (LOQ). The results in Table 1 show that the linearity of all analytes and the correlation coefficient R were greater than 0.997. The intra-day range was 1.4–3.5%, and the inter-day range was 1.5–3.7%. The LODs and the LOQs were calculated from S/N = 3 and 10, respectively. The LODs and LOQs ranged from 2.0 to 2.7 μg kg−1 and 6.6 to 8.9 μg kg−1, which were lower than the MRLs for the European Union and China. These results indicated that the approach has broad application prospects in the analysis of pyrethroid pesticides in cereal samples.
Table 1 Analytical performance of the DES-DLLME-SFOD procedure
Analyte |
Sample |
Linear equation |
Intra-day RSD (%, n = 3) |
Inter-day RSD (%, n = 3) |
R
|
LOD (μg kg−1) |
LOQ (μg kg−1) |
Bifenthrin |
Corn |
y = 111.92x + 6.304 |
2.8 |
2.9 |
0.998 |
2.6 |
8.7 |
Wheat |
y = 97.459x + 15.367 |
2.5 |
2.8 |
0.999 |
2.6 |
8.8 |
Barley |
y = 119.03x − 9.594 |
3.2 |
2.5 |
0.998 |
2.7 |
8.9 |
Oats |
y = 106.81x − 14.262 |
1.4 |
2.1 |
0.999 |
2.6 |
8.8 |
β-Cypermethrin |
Corn |
y = 92.415x + 27.432 |
3.0 |
2.9 |
0.997 |
2.0 |
6.6 |
Wheat |
y = 77.721x + 30.42 |
3.5 |
2.8 |
0.998 |
2.1 |
6.9 |
Barley |
y = 104.66x − 8.4726 |
2.8 |
3.2 |
0.999 |
2.2 |
7.3 |
Oats |
y = 93.728x − 6.257 |
2.9 |
3.6 |
0.999 |
2.1 |
7.1 |
Deltamethrin |
Corn |
y = 129.72x − 12.906 |
3.5 |
3.7 |
0.999 |
2.2 |
7.3 |
Wheat |
y = 116.24x + 32.797 |
2.3 |
2.4 |
0.997 |
2.2 |
7.4 |
Barley |
y = 144.29x − 13.934 |
2.8 |
1.5 |
0.999 |
2.2 |
7.2 |
Oats |
y = 130.76x − 15.262 |
3.3 |
3.7 |
0.999 |
2.2 |
7.3 |
3.3 Analysis of real samples
The proposed DES-DLLME-SFOD procedure was used for the analysis of pyrethroid pesticides in four cereals (corn, wheat, barley, and oats) under optimal conditions. Under optimal conditions, four types of cereal samples with spiked concentrations of 0.25, 2.5, and 25 mg kg−1 were evaluated. The results (Table 2) show that the recoveries of the spiked cereals ranged from 75.6% to 87.2%, and the RSD in the sample solution ranged from 1.5% to 3.6%. Therefore, the proposed technique could be applied to the detection of pyrethroids in cereal samples.
Table 2 Analysis of pyrethroid pesticides in cereal samples
Analyte |
Spiked level (mg kg−1) |
Corn |
Wheat |
Barley |
Oats |
Recovery (%) |
RSD (%, n = 3) |
Recovery (%) |
RSD (%, n = 3) |
Recovery (%) |
RSD (%, n = 3) |
Recovery (%) |
RSD (%, n = 3) |
Bifenthrin |
25 |
80.9 |
2.9 |
81.9 |
3.1 |
85.2 |
1.5 |
82.6 |
2.9 |
2.5 |
80.0 |
1.6 |
82.8 |
3.6 |
85.0 |
3.3 |
84.2 |
1.5 |
0.25 |
85.0 |
3.3 |
84.5 |
3.6 |
83.5 |
3.3 |
87.2 |
2.0 |
β-Cypermethrin |
25 |
83.3 |
1.9 |
80.7 |
3.3 |
82.9 |
3.5 |
80.4 |
2.6 |
2.5 |
84.9 |
3.6 |
79.1 |
2.8 |
83.8 |
2.8 |
80.5 |
2.1 |
0.25 |
84.4 |
3.4 |
82.9 |
3.5 |
81.6 |
2.4 |
77.3 |
1.8 |
Deltamethrin |
25 |
82.8 |
2.9 |
81.8 |
2.5 |
80.1 |
1.5 |
78.4 |
3.0 |
2.5 |
75.6 |
2.5 |
78.9 |
2.1 |
75.7 |
2.8 |
78.5 |
3.4 |
0.25 |
85.3 |
2.8 |
83.9 |
1.9 |
79.0 |
3.0 |
77.3 |
1.9 |
3.4 Comparison of methods
Comparisons between the DES-DLLME-SFOD method proposed in this paper and the other previously reported microextraction methods for pyrethroid are shown in Table 3.34–36 In this study, a novel hydrophobic DES was adopted as the extraction solvent, which is safer and more environmentally friendly than chlorobenzene and dodecanol. Meanwhile, the consumption of extraction solvent and the collection difficulty of extracts could be greatly reduced by using thymol–octanoic acid (1/4) DES as the extraction solvent. The method excludes the use of auxiliary equipment required elsewhere. In conclusion, the comparative results show that this experiment is eco-friendly, efficient, and convenient.
Table 3 Comparison of the proposed method with other microextraction methods for HPLC determination of pyrethroids in food samples
Extraction method |
Analytical instrument |
Matrix |
Extraction solvent |
Auxiliary equipment |
RSD (%) |
LOQ (μg kg−1) |
Recovery (%) |
References |
UA-DLLME: ultrasonic-assisted dispersive liquid–liquid microextraction.
VA-EME: vortex-assisted emulsification microextraction.
VA-DLLME: vortex-assisted dispersive liquid–liquid microextraction.
|
UA-DLLMEa |
HPLC-UV |
Litchi fruit |
Chlorobenzene (310 μL) |
Ultrasonic bath (3 min) |
2.6–5.6 |
4.4–6.2 |
83.3–91.5 |
34
|
VA-EMEb |
HPLC-DAD |
Mushroom |
Dodecanol (300 μL) |
Vortex stirrer (10 min) |
3.1–8.1 |
320–620 |
70.2–89.2 |
35
|
VA-DLLMEc |
HPLC-DAD |
Longan fruit |
Dodecanol (200 μL) |
Vortex stirrer (1.5 min) |
1.2–7.0 |
18.5–50.1 |
77.0–98.6 |
36
|
DES-DLLME-SFOD |
HPLC-DAD |
Cereal |
Deep eutectic solvent (60 μL) |
— |
1.5–3.6 |
6.6–8.9 |
75.6–87.2 |
This study |
4 Conclusion
In this study, a DES-DLLME-SFOD procedure coupled with HPLC was designed to detect pyrethroid pesticides in cereal samples. The choice of extraction solvent should be informed by the principles of green chemistry to enhance the eco-friendliness of the microextraction technique. In this procedure, a novel type of extraction solvent – DES (thymol–octanoic acid) was used, which is a potential substitute for the commonplace halogenated organic solvent used in the process of microextraction. This solvent has the advantages of low cost, easy preparation, eco-friendliness, biodegradability, and the ability to adapt to the needs of green chemistry. With the assistance of solidification, the difficulty of collection and loss of extractants are greatly reduced. This study shows good linearity, sensitivity, extraction recovery, and repeatability. The proposed technique is an eco-friendly, efficient, and convenient method, which has been effectively used for the analysis of pyrethroid pesticides in cereal samples and has the potential for application to other pesticides in future research.
Author contributions
Beiqi Wu: methodology, investigation. Zhengyan Guo: investigation, writing – original draft. Xiuting Li: software, project administration. Xin Huang: investigation, validation. Chao Teng: formal analysis, data curation. Zhenjia Chen: investigation, validation. Xu Jing: conceptualization, writing – review & editing. Wenting Zhao: writing – review & editing, supervision.
Conflicts of interest
There are no conflicts of interest to declare.
Acknowledgements
This work was supported by the Beijing Municipal Education Commission Science and Technology Program General Project [grant number KM201810020002]; the Shanxi Province Key R&D Plan [grant numbers 201703D211001-06, 201903D211006]; and the open fund from Beijing Advanced Innovation Center for Food Nutrition and Human Health [grant number 20161019].
References
- A. S. M. Saleh, P. Wang, N. Wang, S. Yang and Z. G. Xiao, Crit. Rev. Food Sci. Nutr., 2019, 59, 207–227 CrossRef CAS.
- X. H. Hou, X. Zheng, C. L. Zhang, X. W. Ma, Q. Y. Ling and L. S. Zhao, J. Chromatogr. B: Anal. Technol. Biomed. Life Sci., 2014, 969, 123–127 CrossRef CAS.
- S. Chen, S. Gu, Y. Wang, Y. L. Yao, G. Q. Wang, Y. Jin and Y. M. Wu, Environ. Pollut., 2016, 218, 1128–1134 CrossRef CAS.
- A. P. Vonderheide, B. Boyd, A. Ryberg, E. Yilmaz, T. E. Hieber, P. E. Kauffman, S. T. Garris and J. N. Morgan, J. Chromatogr. A, 2009, 1216, 4633–4640 CrossRef CAS.
- L. Huizhen, M. J. Lydy and Y. Jing, Chemosphere, 2016, 144, 2427–2435 CrossRef.
- P. F. Zhao, X. Y. Dong, X. M. Chen, X. J. Guo and L. S. Zhao, J. Agric. Food Chem., 2019, 67, 9362–9370 CrossRef CAS.
- D. D. Han, B. Tang and K. H. Row, J. Chromatogr. Sci., 2014, 52, 232–237 CAS.
- D. B. Martínez, P. P. Vázquez, M. M. Galera and M. D. G. García, Chromatographia, 2006, 63, 487–491 CrossRef.
- F. G. Ye, Z. G. Xie, X. P. Wu and X. C. Lin, Talanta, 2006, 69, 97–102 CrossRef CAS.
- W. W. Deng, L. Yu, X. Li, J. Chen, X. X. Wang, Z. X. Deng and Y. X. Xiao, Food Chem., 2019, 274, 891–899 CrossRef CAS.
- W. Tang and K. H. Row, J. Cleaner Prod., 2020, 268, 122306 CrossRef CAS.
- W. Y. Tang, Y. N. An and K. H. Row, J. Mol. Liq., 2020, 306, 7 CrossRef.
- P. Makos, E. Slupek and J. Gebicki, Microchem. J., 2020, 152, 16 CrossRef.
- A. Bazmandegan-Shamili, S. Dadfarnia, A. M. H. Shabani, M. R. Moghadam and M. Saeidi, J. Iran. Chem. Soc., 2018, 15, 1181–1189 CrossRef CAS.
- E. L. Smith, A. P. Abbott and K. S. Ryder, Chem. Rev., 2014, 114, 11060–11082 CrossRef CAS.
- W. Y. Tang and K. H. Row, Bioresour. Technol., 2020, 296, 9 CrossRef.
- A. Shishov, P. Terno, L. Moskvin and A. Bulatov, Talanta, 2020, 206, 120209 CrossRef CAS.
- Q. H. Zhang, K. D. Vigier, S. Royer and F. Jerome, Chem. Soc. Rev., 2012, 41, 7108–7146 RSC.
- Q. Lu, L. Qiu, L. Yu, S. Zhang, R. A. de Toledo, H. Shim and S. Wang, J. Hazard. Mater., 2019, 368, 849–861 CrossRef CAS.
- M. Shamsipur, N. Fattahi, Y. Assadi, M. Sadeghi and K. Sharafi, Talanta, 2014, 130, 26–32 CrossRef CAS.
- H. L. Wang, H. Yan, C. J. Wang, F. Chen, M. P. Ma, W. W. Wang and X. D. Wang, J. Chromatogr. A, 2012, 1253, 16–21 CrossRef CAS.
- T. Asadollahi, S. Dadfarnia and A. M. H. Shabani, Talanta, 2010, 82, 208–212 CrossRef CAS.
- D. van Osch, L. F. Zubeir, A. van den Bruinhorst, M. A. A. Rocha and M. C. Kroon, Green Chem., 2015, 17, 4518–4521 RSC.
- J. G. March and V. Cerda, Talanta, 2016, 156, 204–208 CrossRef.
- K. Li, Y. Jin, D. Jung, K. Park, H. Kim and J. Lee, J. Chromatogr. A, 2020, 1614, 9 Search PubMed.
- H. Sereshti, S. S. Jazani, N. Nouri and G. Shams, Microchem. J., 2020, 158, 105269 CrossRef CAS.
- R. A. Zounr, M. Tuzen, N. Deligonul and M. Y. Khuhawar, Food Chem., 2018, 253, 277–283 CrossRef CAS.
- K. Shahbaz, S. Baroutian, F. S. Mjalli, M. A. Hashim and I. M. AlNashef, Thermochim. Acta, 2012, 527, 59–66 CrossRef CAS.
- G. Li and K. H. Row, TrAC, Trends Anal. Chem., 2019, 120, 16 Search PubMed.
- H. Musarurwa and N. T. Tavengwa, Food Chem., 2020, 127943, DOI:10.1016/j.foodchem.2020.127943.
- M. Torbati, A. Mohebbi, M. A. Farajzadeh and M. R. A. Mogaddam, Anal. Chim. Acta, 2018, 1032, 48–55 CrossRef CAS.
- A. M. Carro, S. Fernandez, I. Racamonde, D. Garcia-Rodriguez, P. Gonzalez and R. A. Lorenzo, J. Chromatogr. A, 2012, 1253, 134–143 CrossRef CAS.
- Y. F. Zhang and H. K. Lee, J. Chromatogr. A, 2013, 1274, 28–35 CrossRef CAS.
- K. Wang, X. J. Xie, Y. Zhang, Y. X. Huang, S. Y. Zhou, W. Zhang, Y. Y. Lin and H. J. Fan, Food Chem., 2018, 240, 1233–1242 CrossRef CAS.
- W. F. Zhao, X. Jing, M. C. Chang, J. L. Meng and C. P. Feng, Bull. Korean Chem. Soc., 2019, 40, 943–950 CrossRef CAS.
- Z. X. Chen, Q. Li, T. C. Yang, Y. F. Zhang, M. H. He, H. Y. Zeng, X. M. Mai, Y. T. Liu and H. J. Fan, J. Chromatogr. A, 2020, 1619, 11 Search PubMed.
Footnote |
† Electronic supplementary information (ESI) available. See DOI: 10.1039/d0ay02121k |
|
This journal is © The Royal Society of Chemistry 2021 |