DOI:
10.1039/D0AY01732A
(Paper)
Anal. Methods, 2021,
13, 327-336
Perchlorate detection via an invertebrate biosensor
Received
11th September 2020
, Accepted 14th December 2020
First published on 15th December 2020
Abstract
Improvised explosive devices (IEDs) are constructed from easily obtainable ingredients that are often unregulated and difficult to trace. Salts of the oxyhalide perchlorate are frequently used as oxidisers in IEDs and in commercially available munitions, thus a reliable detection is needed to aid forensic investigations and the tracing of environmental ground or surface water contamination. We introduce the nematode Caenorhabditis elegans as a biosensor for the presence of perchlorate, a promising alternative to the costly, technically challenging and time-consuming current perchlorate detection methods. Perchlorate uptake dynamics in C. elegans were first validated using ion exchange chromatography followed by assessing the effects of perchlorate on key life-point indices to verify the suitability of the nematodes as a forensic biosensor. Whole genome microarrays and qPCR analyses established that a set of immune and stress response genes were enriched during perchlorate exposure. A nematode strain (agIs219) containing an integrated copy of the significantly overexpressed t24b8.5 gene promoter followed by a GFP reporter gene was shown to fluoresce in a perchlorate dose dependent manner with a limit of detection (LOD) of 0.5 mg mL−1. Whilst chemicals commonly used in the construction of IEDs did not induce fluorescence, exposure to other oxyhalides did, highlighting the presence of possible shared stress response pathways. Burnt wire sparklers containing potassium perchlorate elicited fluorescence while other non-perchlorate containing post-blast explosion matrices did not. This demonstrates how C. elegans can be used to screen for perchlorate at environmental hotspots, an optimization, possibly with other target transgenes, is required to enable the detection of perchlorate at concentrations below 0.5 mg mL−1.
Perchlorate (ClO4−) is a powerful oxidiser used widely in explosives, fireworks and munitions. It is also a naturally occurring anion at very low concentrations in the environment that can be formed by atmospheric deposition following ozone/chlorine reactions in lightning storms and is found associated in deposits of nitrate and potash.1,2 Although perchlorate is a powerful oxidant, it is very stable under normal environmental conditions. For any reduction to occur, an oxygen atom is removed thereby eliminating the direct interaction of the chlorine atom with the reducing agent which causes perchlorate to persist in the environment.3–5 Exposure to such contaminated water sources can impair, in humans, the normal function of the thyroid gland. This is caused by perchlorate binding to the thyroid iodine receptors which can result in hypothyroidism and foetal retardation.6–10 Improvised explosive devices (IEDs), which are frequently utilized during acts of terrorism,11–13 typically consist of an oxidizer, a fuel source such as sugar, and a fuse for ignition.14 Inorganic oxidizers including perchlorate and chlorate are categorized as low-order explosives and their detection is often challenging due to the limited availability of techniques that enable their detection. Indeed, most efforts have focused on the detection of organic high-order explosives, such as TNT (2,4,6-trinitrotoluene) and RDX (Royal Demolition eXplosive).15–20 According to the Technical Working Group for Fire and Explosives Analysis (TWGFEX), the most appropriate approach for confirmatory analysis is to employ combinations of uncorrelated techniques across four categories. The characterisation of inorganic salt-based explosives in forensic science casework is typically performed using ion exchange chromatography (IC) and/or capillary electrophoresis (CE) with suppressed conductivity, ultraviolet or mass spectrometric detection (MS) as well as a selection of spot tests.21–26 Whilst some of the instrumental techniques are particularly powerful for high assurance analysis, more than one of each is often unavailable together in practicing forensic laboratories. Development of additional uncorrelated approaches to IC and/or CE that are also inexpensive, specific and deployable on massive scales are therefore desirable to increase the capacity in laboratories that do not house such advanced analytical capabilities. One such approach that has not yet been explored is the utilization of a perchlorate biosensor, a detection technique that transduces the response generated by the biological component to perchlorate into optical or electrical signals.27 Numerous biosensors have been developed to detect high order explosives, including canines, rats, bees, wasps, moths and even genetically modified microorganisms,28–32 however a biosensor for perchlorate is, to date, elusive. To enable the detection of perchlorate, the candidate biosensor should be amenable for genetic manipulation and the yielded optical signals easily accessible. We therefore propose the use of the model organism Caenorhabditis elegans, as it possesses a very simple anatomical structure, is transparent, easy to cultivate in a laboratory setting, has a compact genome and a very short life cycle, and most importantly is suitable for genetic analysis and manipulation.33
Experimental section
Reagents and materials
All reagents used were of analytical or reagent grade. All chemicals (unless stated) were prepared from their sodium salts, including perchlorate, iodide, periodate, chlorate, chloride, bromate, sulphate, ammonium, nitrate, nitrite, phosphate and sucrose (Sigma-Aldrich, Gillingham, Dorset, UK). Perchlorate, nitrite, chlorate, periodate, iodide, bromate and phosphate were prepared fortnightly (100 mg mL−1) in ultrapure water and were stored in the dark at 4 °C from which working solutions were prepared daily. Eluents for IC were prepared using a 50% w/v solution of sodium hydroxide in water. Commercial products containing perchlorate as an oxidizer used here for biosensor application were the black powder substitute Pyrodex® (Wilson & Wilson, Fieldsports Ltd., Ramsgate, Kent, UK) and burnt wire sparklers (i.e., fireworks). RDX post-explosion residues (exploded car parts were provided by Event Horizon Precision Energetics, Ashcott, Somerset) were also utilised as standards were prepared by dissolving the residues in ultrapure water overnight. The chemicals were prepared by dissolving the appropriate amount of their powders/salts in filtered double autoclaved ultrapure deionized water (18.2 MΩ cm) delivered from a Millipore Synergy water ultra-purification system (Millipore, Bedford, MA, USA). All chemicals were kept in glass bottles at 4 °C and filtered before each use with a 0.22 μM filter. The wild type C. elegans strain N2 (Bristol, Great Britain) was utilized as well as a transgenic strain agIs219 (Pt24b8.5::GFP::unc-54-3′UTR; Pttx-3:GFP::unc-54-3′UTR) obtained from the Caenorhabditis Genetic Centre (CGC) and constructed by Shivers et al., (2009).34 The E. coli strain OP50 was grown on Nematode Growth Medium (NGM) plates as a source of food to the worms, which were inoculated on the plates to conduct experiments at 20 °C.
Measuring the uptake dynamics of perchlorate in C. elegans using IC
Perchlorate was quantitatively determined in C. elegans using a Dionex DX500 IC system comprising of a GP40 analytical pump, AS40 autosampler and an ED40 conductivity detector fitted with a Dionex ASRS 300 (2 mm ID) electrolytic suppressor (current = 50 mA). Instrument control and data acquisition were performed using Chromeleon 6.6. All separations were performed on a Thermo Scientific™ Dionex™ IonPac™ AS20 anion exchange column (2 × 250 mm) with an injection volume of 30 μL and a flow rate of 0.30 mL min−1. Isocratic elution was performed using an eluent containing 30 mM NaOH. Daily calibration curves were constructed for perchlorate and run by IC over a 100–1000 ng mL−1 range (Fig. 1A).
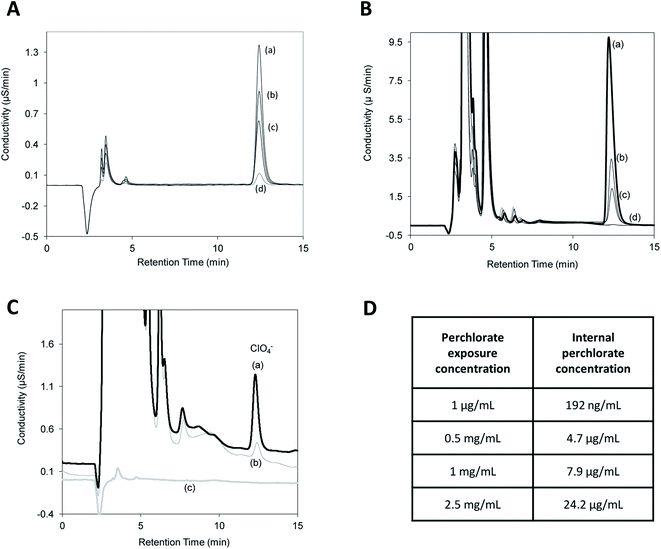 |
| Fig. 1 (A) Overlaid IC chromatogram of sodium perchlorate standard at (a) 1000 ng mL−1, (b) 750 ng mL−1, (c) 500 ng mL−1 and (d) 100 ng mL−1. (B) Matrix-matched standards of unexposed nematodes with extracts spiked with (a) 2.5 mg mL−1, (b) 1 mg mL−1, (c) 0.5 mg mL−1 or (d) 1 μg mL−1 perchlorate for 24 hours from L1 stage. (C) Overlaid IC chromatograms of (a) a 1 μg mL−1 sodium perchlorate fortified lysate retrieved from nematodes exposed to 0.5 mg mL−1 perchlorate, (b) an extract of nematodes exposed to 0.5 mg mL−1 perchlorate only and (c) last washout solution of the exposed nematodes. (D) A comparison between external perchlorate concentration (applied dose) and internal perchlorate concentration (ingested). | |
A synchronized population of L1 stage worms was exposed to 0.5 mg mL−1 perchlorate, added to both the agar and E. coli OP50, for 24 hours at 20 °C. The worms were washed 7 times with ultrapure deionized water to eliminate any exogenous sources of perchlorate including the agar and the E. coli OP50. The last washout solution was saved to verify using IC that the perchlorate peaks originated from the worms and not from the exposure medium. The washed worms were subsequently flash frozen in liquid nitrogen and stored at −80 °C for 1 hour. The worm pellet was then submerged in 3 mL of ultrapure deionized water supplemented with glass beads and lysed by ultra-sonication for 10 minutes. The lysate was passed through a 0.45 micron filter prior to sample injection. The worm lysate was analysed directly by IC in triplicate followed by a matrix-matched standard containing 1 μg mL−1 perchlorate.
Sample normalisation by Bradford assay
The nematodes were normalised to protein content via the Bradford Coomassie brilliant blue assay prior to IC analysis. The worms (∼40
000 L1 nematodes) were treated with perchlorate (1 μg mL−1, 0.5 mg mL−1, 1 mg mL−1 or 2.5 mg mL−1) for 24 hours at 20 °C. The samples were washed and collected as stated previously and the total protein concentration in the nematodes' lysate solutions was quantified using the Bradford assay at 595 nm. The lysates were then measured for their perchlorate content using IC.
Perchlorate effect on key life-point indices
The effects of perchlorate on the growth, eating behaviour, reproduction and lifespan were investigated. Synchronized L1 worms were added to plates containing 1 ng mL−1, 0.5 μg mL−1, 1 μg mL−1, 0.5 mg mL−1, 1 mg mL−1 or 3 mg mL−1 sodium perchlorate in both the agar and the E. coli OP50 bacteria. Randomly selected worms (n = 10 worms per condition) were photographed once a day for 5 days using an upright microscope/camera system (SMZ1500, Nikon). The growth was assessed by measuring the flat volumetric surface area of the photographed nematodes using Image-pro Express 5.1 Software (Media Cybernetics). The total brood size of sodium perchlorate challenged worms was determined until the egg laying period was completed by plating synchronized L1 stage worms on NGM plates containing perchlorate concentrations equal to those utilised in the growth assay. The worms were then allowed to grow until they reached L4 stage and individual worms (12 worms per condition) were transferred to single NGM agar containing wells seeded with E. coli OP50 supplemented with the relevant perchlorate concentration. Worms were allowed to reach adulthood and lay eggs for one day, thereafter each worm was transferred to a new well in a new 12 well plate and allowed to lay eggs for the second day of adulthood. The number of hatched progeny of each worm was counted one day after egg laying. The process of transferring the worms and counting the progeny was performed for 6 days during which the total number of eggs per worm in each condition was counted. The life span of approximately 400 synchronized L1 stage worms was investigated upon addition of 1 ng mL−1, 1 μg mL−1 or 1 mg mL−1 sodium perchlorate to both the agar and the E. coli OP50. The worms were transferred on a daily basis to fresh NGM plates containing the same concentration of sodium perchlorate until egg laying ceased. Thereafter, the worms were transferred to new plates every two days. The number of dead worms was scored on a daily basis for approximately three weeks (18 days) until no viable worms were observed. To assess the effects of perchlorate on the eating behaviour of the nematodes, synchronized L1 stage worms were placed onto unexposed NGM plates or plates containing sodium perchlorate (1 ng mL−1, 10 ng mL−1, 100 ng mL−1, 1 μg mL−1, 75 μg mL−1, 0.5 mg mL−1 or 1 mg mL−1 added to both the agar and the E. coli OP50). The worms were allowed to grow at 20 °C until they reached late L4 stage when their internal organs can be easily visualised under a microscope. The number of pharyngeal pumps was determined by manual counting over a 30 seconds time frame from at least 10 worms per condition. To assess if perchlorate disrupts the defecation cycles of C. elegans, the time required for ten worms to defecate in two successive defecation cycles was recorded.
DNA microarrays and qPCR analysis
A whole genome microarray was utilised from cDNA samples derived from nematodes raised from L1 to L4 stage in the presence or absence of 1 mg mL−1 perchlorate. In detail, the total RNA of the synchronized nematodes was extracted and subsequently converted to cDNA which was then hybridized to the Affymetrix GeneChip™ C. elegans Gene 1.0 ST array according to the manufacturer's protocol. The microarray data was normalised using the RMA algorithm, and the Principal Component Analysis (PCA) and gene expression data analysis were performed using Transcriptome Analysis Console (TAC) Software 4.0 and Qlucore Omics Explorer version 3.4, respectively. The differentially expressed genes that met the cut-off criteria (>1.4 or <0.7) were analysed using the Search Tool for the Retrieval of Interacting Genes/Proteins (STRING), software version 10.5. The microarray data were validated using qPCR analysis of t24b8.5, zip-10, lys-7, sma-9, sek-1 and vhp-1 in multiple cDNA samples of worms treated with 1 mg mL−1 perchlorate for 48 hours from L1 to L4 stage.
Exposure of transgenic worms and assessment of fluorescence
A synchronized L1 population of the agIs219 transgenic worms was raised for 24 hours at 20 °C with or without perchlorate (unexposed or 1 μg mL−1, 0.5 mg mL−1, 1 mg mL−1, 2.5 mg mL−1 or 5 mg mL−1 added to both the E. coli OP50 and the agar). Worms (n = 15 per condition) were immobilized on glass slides using sodium azide and their fluorescence emission was investigated using a Nikon (Eclipse TE2000) inverted fluorescence microscope with blue laser scanning fluorescence (λex = 450–490 nm).
Assessing the specificity of the agIs219 worms to perchlorate
The agIs219 nematodes (n = 10–15 per condition) were exposed to 2.5 mg mL−1 ammonium chloride (NH4Cl), sodium sulphate (Na2SO4), trisodium phosphate (Na3PO4), sucrose (C12H22O11), sodium nitrite (NaNO2), or sodium nitrate (NaNO3) from L1 stage for 24 hours. The worms were also exposed to sodium bromate (NaBrO3), sodium iodide (NaI), sodium chlorate (NaClO3) or sodium periodate (NaIO4) at 1 mg mL−1 for 24 hours at 20 °C. A 1 mg mL−1 concentration was used instead of 2.5 mg mL−1 because of the relevant toxicity of these chemicals. Imaging was conducted using the Nikon (Eclipse TE2000) inverted fluorescence microscope with blue laser scanning fluorescence (λex = 450–490 nm) after immobilisation on a glass slide using sodium azide. The nematodes were also exposed to Pyrodex®, a black powder substitute containing potassium perchlorate as an oxidizer, burnt wire sparklers initially containing potassium perchlorate in their unburnt form and RDX post-explosion residues.
Results and discussion
Uptake dynamics of perchlorate
Biosensors, if optimised correctly, offer a fast, inexpensive and highly sensitive alternative to traditional detection methods. Here we propose the use of genetically engineered Caenorhabditis elegans strains to serve as an alternative detector for perchlorate. The ability of the worms to serve as a biosensor for perchlorate depends on the uptake of perchlorate by nematodes and any subsequent effects of perchlorate on the animal. It was important to also assess the effects of the drug on the bacterial food source. After obtaining which perchlorate concentrations are tolerated by the bacteria, the actual effects of perchlorate on the nematodes' growth were investigated. The growth of E. coli OP50 was not affected when perchlorate was added at doses up to 10 mg mL−1, whilst a dose of 25 mg mL−1 was growth inhibitory (data not shown). Although microbes such as proteobacteruim Azospira suillum strain PS (formally Dechlorosoma suillum strain PS) can use perchlorate as an electron donor for microbial metabolism, E. coli OP50 is neither a halophile nor a perchlorate reducing bacteria.35–40
To assess the suitability of utilizing C. elegans as a perchlorate biosensor required the uptake dynamics of perchlorate in the nematodes to first be assessed via IC analysis of unexposed worm lysates spiked with perchlorate and compared to perchlorate standards (Fig. 1A and B). The lysates of worms exposed to mg mL−1 of perchlorate contained μg mL−1 quantities of perchlorate revealed a dose dependent increase in perchlorate but indicated that the worms are most likely not able to bio-concentrate perchlorate from the outside environment (Fig. 1C and D) and therefore present a similar model of perchlorate activity as in humans.6 Several studies have reported that perchlorate is excreted with a half-life of 6–8 hours and is not metabolized because the chemical reduction induced by the strong chlorine–oxygen bonds is rare.6–41 In addition, although perchlorate exhibits a high redox potential, it was shown to be excreted virtually unchanged in rats.42
Perchlorate effects on key life-cycle endpoints
Evaluating the physiological effects that perchlorate exerts on the worms was of paramount importance, as an understanding of its toxicity is required to define the worms limitations and in turn assess their suitability as perchlorate biosensors. In detail, exposure to increasing concentrations of perchlorate resulted in a gradual decrease in body size (Fig. 2A). The decreased body size is a common concept in the toxicology of C. elegans where various toxins have been shown to affect the growth of the nematodes by decreasing the growth rate or reducing the nematodes size as observed, for example, with Aflatoxin B1 (AFB1).43 In addition, the nematodes were reported to shrink in hypertonic solutions which might have been caused by elevated perchlorate salt concentrations.44 However, a perchlorate dose of 5 mg mL−1 is very toxic to the worms as indicated by their developmental arrest at the L1 stage.
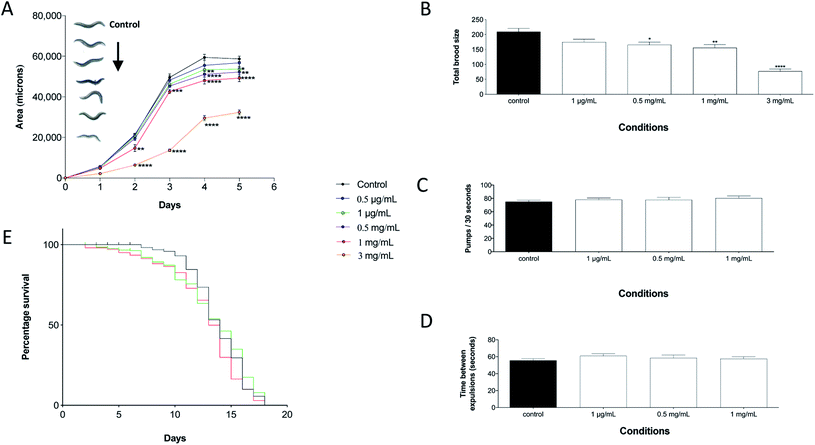 |
| Fig. 2 The physiological effects of perchlorate on C. elegans. (A) The growth of C. elegans (area measurements) exposed to perchlorate at 0.5 μg mL−1, 1 μg mL−1, 0.5 mg mL−1, 1 mg mL−1 or 3 mg mL−1. The statistical significance of each treatment in comparison to control (n = 10) was calculated using a two-way ANOVA with Dunnett's multiple comparison test. Error bars represent ± SEM (*P ≤ 0.05, **P ≤ 0.01, ***P ≤ 0.001 and ****P ≤ 0.0001). (B) The total brood size of perchlorate treated worms. The data were analysed using a one-way ANOVA with Dunnett's multiple comparison test (n = 12). Error bars indicate ± SEM (*P ≤ 0.05, **P ≤ 0.01 and ****P ≤ 0.0001). (C) The pharyngeal pumping frequency (pumps/30 seconds) of worms exposed to perchlorate for 72 hours from L1 stage. (D) The time required for worms exposed to perchlorate for 72 hours from L1 stage to complete two successive defecation cycles. The data in (C) and (D) were analysed using one-way ANOVA with Dunnett's multiple comparison test (n = 10). (E) The percentage survival of C. elegans in the presence of 1 μg mL−1 or 1 mg mL−1 perchlorate. The survival curve was plotted by the Kaplan–Meier method (n > 200) and the data was analysed using the log-rank (Mantel–Cox test) statistical analysis. | |
A direct indication of the effects of potential toxic compounds on nematodes can be indicated by a change in reproduction.45 The total brood size of sodium perchlorate treated worms decreased upon perchlorate exposure and it was reduced by almost 50% at 3 mg mL−1 with a 24 hour delay in the onset of reproduction (Fig. 2B). This dose caused a significantly reduced growth rate of the treated worms which explains the delay in the onset of egg laying. However, many other compounds confer reproductive toxicity in C. elegans, etoposide for example, a clinical anticancer drug, was found to severely decrease the reproduction rate of the worms.46 Upon testing the effects of perchlorate on the life span of C. elegans, it was evident that there were no significant changes upon exposure to 1 μg mL−1 sodium perchlorate in comparison to unchallenged control worms. Exposure to the same dose did not alter the median survival time, namely 14 days was equivalent to the median survival of unexposed worms (Fig. 2E). In contrast, worms treated with 1 mg mL−1 sodium perchlorate were marked by a statistically significant decrease in median survival (by one day), indicating the potency of this dose on the life span of the nematodes. A one-day decrease, despite being statistically significant, will not limit C. elegans utilisation in subsequent experiments to test for the ability of the worms to detect perchlorate. As both pharyngeal pumping and defecation rates were not affected by perchlorate exposure, the reduced growth, brood size and survival after perchlorate exposure were the result of the chemical itself and not a consequence of an altered eating behaviour (Fig. 2C and D). For example, it was reported that Reductil®, a drug used to treat obesity, can affect key life point indices by altering the eating behaviour of the worms as nematodes exhibited decreased growth rates as a consequence of reduced defecation and pharyngeal pumping frequencies.47 The analyses presented here therefore demonstrate that perchlorate is well tolerated at high levels which indicate that the worms can be used as a biosensor of perchlorate exposure over a wide range of doses. However, growth and reproduction are affected at doses reaching 3 mg mL−1 and lethality is observed at concentrations higher than 5 mg mL−1, which are levels that are rarely encountered in nature and can only be present at extreme spill or explosion sites.5
Microarray, qPCR analysis
In search for a perchlorate responsive gene, a whole genome microarray was conducted comparing unexposed worms with counterparts challenged with 1 mg mL−1 perchlorate. This revealed the enrichment of pathways implicated in growth, morphogenesis, stress and defense response as reflected in the observed phenotypic changes induced by perchlorate (Fig. 3A and B). Interestingly, perchlorate was previously reported to influence the mitochondrial metabolic activity and to even enhance the formation of Reactive Oxygen Species (ROS) in the mitochondria and increase the mitochondrial permeability transition pore (mPTP) openings in the goldfish Carassius auratus causing hepatocytes destruction.48,49 The generated ROS were hypothesized to enhance DNA damage based on perchlorate induced DNA fragmentation in the isolated mitochondria.50,51 Indeed, several antioxidant genes and oxidoreductases were enriched following perchlorate exposure in the nematodes. In fact, 2.4% of the enriched genes were oxidoreductases indicating that perchlorate may well be implicated in the formation of ROS which might have induced oxidative stress in the worms. Incidentally, one of the mechanisms for pathogen elimination is through the generation of ROS. For instance, in Drosophila, a NADPH dual oxidase system (DUOX) is activated in response to infection by the bacterial derived uracil which induces the formation of ROS in the intestines as a defence mechanism.52 This is also true for C. elegans,53 whereby a reduced expression of the DUOX, bli-3 for example, was reported to lead to increased susceptibility to pathogens and to reduce hydrogen peroxide production.54 Moreover, immunity is linked to stress response primarily through the activation of p38 MAPK pathway and JNK pathway in mammals, and in fact, C. elegans innate immunity has been reported to be linked to abiotic stress, including fluctuations in temperatures, osmolarity or exposure to immunity and response to xenobiotic stress.55 The expression of t24b8.5 exhibited a consistent increase in expression in 5 independently generated samples of worms treated with 1 mg mL−1 perchlorate (Fig. 3C). For this reason we opted to use a transgenic worm strain to investigate the utility and sensitivity as a putative perchlorate biosensor.
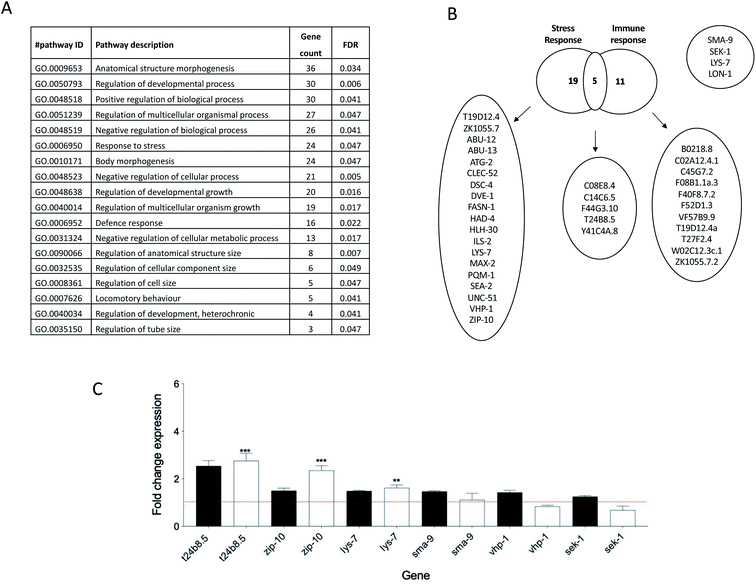 |
| Fig. 3 The effect on gene expression profiles in worms exposed to 1 mg mL−1 perchlorate. (A) The most enriched pathways with False Discovery Rate (FDR) and the gene count (based on STRING analysis) in nematodes challenged with perchlorate. (B) The differentially expressed genes that are implicated in innate immunity, stress response or both in worms exposed to perchlorate. (C) The qPCR analysis of the upregulated immunity/stress response genes as revealed by the microarray analysis of the cDNA samples of perchlorate treated worms. The fold change expression of the innate immunity genes was normalised to rla-1, an invariant housekeeping gene, and was calculated using the delta–delta Ct method. For each gene, the data consists of four different experiments (with the exception of t24b8.5 gene, which represents 5 different experiments) and was calculated using a two-tailed t-test in comparison to control samples (n = 4 or 5). The error bars represent ± SEM (**P ≤ 0.01 and ***P ≤ 0.001). The qPCR results are presented in the white bars while black bars indicate the expression of the genes as denoted in the microarray profile. | |
Biosensor sensitivity
The transgenic strain agIs219 (Pt24b8.5::GFP::unc-54-3′UTR and Pttx-3:GFP::unc-54-3′UTR) expresses GFP driven by the t24b8.5 promoter but also Pttx-3:GFP as a positive selection marker (ttx-3 expression is limited to AIY interneurons and surrounding muscles in the head of the worm). AgIs219 has been used to study innate immunity response of C. elegans during infection as it is strongly inducible upon the activation of PMK-1, the major regulator of innate immunity in the nematodes. Moreover, T24B8.5 is a ShK-like toxin peptide and its expression is regulated by the PMK-1/p38 MAPK pathway and the transcription factor ATF-7.34 The agIs219 worms responded to a perchlorate challenge via a gradual increase in fluorescence intensity at 0.5 mg mL−1, 1 mg mL−1 and 2.5 mg mL−1. Although the toxicity of perchlorate affected the fluorescence at the 5 mg mL−1, the signal remained statistically significantly higher than the unexposed controls (Fig. 4A and B). Whether perchlorate induces its effects through the activation of PMK-1 via ROS or through other effectors remains to be elucidated. Indeed many toxic compounds can induce stress through PMK-1 by either the TIR-1, NSY-1 and SEK-1 module, through PMK-1 directly, through VHP-1 or by means of the KGB-1 pathway. Other stress pathways that may be involved in the stress response to perchlorate may include the activation of DAF-16 or JNK-1 pathways. The absolute LOD of the biosensor was determined to be 0.1 ng (by mass), which is the more frequent means to report LOD in explosives analysis of bulk materials. This lack of sensitivity somewhat limits its utility for trace analysis of explosives and certainly cannot claim to match the sensitivity of IC and IC-MS (LOD: 0.7 pg to 1.2 ng).56 Nevertheless this proof of concept highlights the potential of biosensors as an alternative/complementary approach to classical forensic analysis.
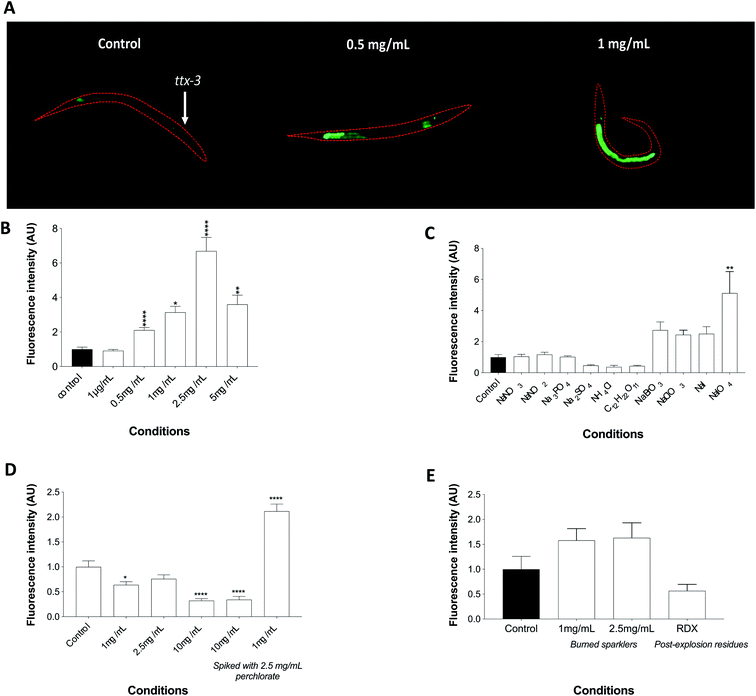 |
| Fig. 4 The sensitivity and specificity of the transgenic nematode agIs219 (Pt24b8.5::GFP::unc-54-3′UTR and Pttx-3:GFP::unc-54-3′UTR) to perchlorate and other chemicals. (A) Representative fluorescence microscopy images of transgenic nematodes exposed to perchlorate (0.5 mg mL−1 and 1 mg mL−1) for 24 hour (L1 to L4 stage) at 20 °C. The microscopic images were obtained by means of a Nikon (Eclipse TE2000) inverted fluorescence microscope equipped with a blue laser scanning fluorescence (λex = 450–490 nm). Quantification of fluorescence signals emitted by worms challenged with (B) pure perchlorate (0–5 mg mL−1), (C) chemicals (all 2.5 mg mL−1) commonly used in explosives and oxyhalides displaying major structural similarities to perchlorate, (D) Pyrodex® spiked with 2.5 mg mL−1 perchlorate, and (E) burned sparklers and RDX post explosion residues. The statistical analysis of the data was performed via a one-way ANOVA with Tukey's multiple comparison test. The data were normalised to control. Error bars indicate ± SEM (*P ≤ 0.05, **P ≤ 0.01 and ****P ≤ 0.0001). | |
Biosensor specificity
IEDs contain other constituents such as sugar, phosphate, sulphur or nitrate, therefore an experiment was required to test for the reactivity of the worms to a set of chemicals commonly used in homemade explosives. An exposure to 2.5 mg mL−1 of each compound did not induce fluorescence, thereby verifying the selectivity of the transgenic nematodes to perchlorate. Perchlorate therefore seems to generate a stress response that is most likely quite specific to at least its class of chemicals. An exposure to other oxyhalides that are closely related to perchlorate and share common chemical characteristics was also conducted. Namely, the nematodes were exposed to bromate, chlorate and periodate in addition to sodium iodide since perchlorate competes with the latter for binding to the thyroid receptors in vertebrates and therefore may act in a similar manner. The exposures were performed at 1 mg mL−1, as these compounds are highly toxic to the worms, but with the exception of periodate (perchlorate's most similar compound) none were able to trigger a significant increase in fluorescence (Fig. 4C). Whilst the limited range in dose-dependent response and cross-reactivity with periodate would preclude its consideration in higher category tests by TWGFEX, for example, its selectivity in the presence of a number of other closely related compounds could still enable its classification as a new Category 3 or 4 test for intact bulk explosive material for forensic purposes.57
While exposure to perchlorate in its pure form elicited a significant response, such scenario is far from reality as a complicated matrix is more likely to be encountered. The nematodes were therefore challenged with various concentrations of Pyrodex®, all of which failed to elicit fluorescence. Interestingly, low doses of Pyrodex® spiked with perchlorate caused a significant increase in fluorescence in comparison to high doses of Pyrodex® also spiked with perchlorate indicating that the latter might not be bioavailable for immediate uptake by the nematodes in complex matrices (Fig. 4D). However, exposure to burnt wire sparklers invoked fluorescence which, although was statistically not very robust, increased in comparison to worms challenged with RDX containing post explosion residues, a high order explosive that does not contain perchlorate (Fig. 4E).
Conclusion
The ability of the worms to detect perchlorate and other similar compounds is a major advantage within a forensic and environmental context. We believe this to be the first whole animal biosensor that is able to detect this class of chemicals at low cost with the ability to be deployed on a large(er) scales. The transgenic worms have the potential to be developed as a presumptive/indicative test before a confirmatory test is performed for bulk forensic explosives analysis (e.g. using a conventional ion chromatography technique) to reveal the culprit anion. However, the current LOD of the current biosensor is 0.5 mg mL−1, which is a concentration encountered in environmental hotspots.5 To increase the specificity of the nematodes additional, perhaps other more sensitive transgenes should be explored or could be optimized through targeted gene deletions of up or downstream enhancers. In addition, further mechanistic studies would aid in defining the underlying cause for the observed decrease in perchlorate bioavailability in complex mixtures. Despite these caveats, this study introduces the nematode's potential as an orthogonal biosensor for the forensic detection an environmental contamination of perchlorate.
Author contributions
Conceptualization – SAA, LB and SS; formal analysis – SAA; investigation – SAA; methodology – SAA, LB and SS; project administration – SS; resources – SS and LB; supervision – SS and LB; validation – SAA; visualization – SAA; writing (original draft) – SAA; writing (review and editing) – SS; writing (final refinement) – SAA, LB and SS.
Conflicts of interest
The authors declare no competing financial interest.
Acknowledgements
The study was supported via a PhD studentship (to SAA) funded by Kuwait University.
References
- M. L. Smith, M. W. Claire, D. C. Catling and K. J. Zahnle, The formation of sulfate, nitrate and perchlorate salts in the martian atmosphere, Icarus, 2014, 231, 51–64 CrossRef CAS.
- C. W. Trumpolt, M. Crain, G. D. Cullison, S. J. P. Flanagan, L. Siegel and S. Lathrop, Perchlorate: Sources, uses, and occurrences in the environment, Remediation Journal, 2005, 16(1), 65–89 CrossRef.
-
H. F. Stroo, R. C. Loehr and C. H. Ward, In Situ Bioremediation of Perchlorate in Groundwater: An Overview, in In Situ Bioremediation of Perchlorate in Groundwater, ed. H. F. Stroo and C. H. Ward, Springer New York, New York, NY, 2009, pp. 1–13 Search PubMed.
-
K. Sellers, K. Weeks, W. R. Alsop, S. R. Clough, M. Hoyt, B. Pugh, et al., Perchlorate: Environmental problems and solutions, CRC Press, 2006 Search PubMed.
- E. T. Urbansky, Perchlorate chemistry: implications for analysis and remediation, Biorem. J., 1998, 2(2), 81–95 CrossRef CAS.
-
N. R. Council, Health implications of perchlorate ingestion, National Academies Press, 2005 Search PubMed.
- R. A. Clewell, E. A. Merrill, L. Narayanan, J. M. Gearhart and P. J. Robinson, Evidence for competitive inhibition of iodide uptake by perchlorate and translocation of perchlorate into the thyroid, Int. J. Toxicol., 2004, 23(1), 17–23 CrossRef CAS.
-
J. J. Clark, Toxicology of Perchlorate. Perchlorate in the Environment, Springer, 2000, pp. 15–29 Search PubMed.
- W. Trotter, The relative toxicity of antithyroid drugs, J. New Drugs, 1962, 2(6), 333–343 CrossRef CAS.
- J. Wolff, Perchlorate and the thyroid gland, Pharmacol. Rev., 1998, 50(1), 89–106 CAS.
-
I. Overton, Improvised explosive device (IED) monitor 2017 report, Tech. Rep, Action on Armed Violence (AOAV), 2017 Search PubMed.
-
Parsons Green: Underground blast a terror incident, say police, https://www.bbc.co.uk/news/uk-41278545, accessed May 24, 2020 Search PubMed.
-
July 7 2005 London Bombings Fast Facts, https://www.cnn.com/2013/11/06/world/europe/july-7-2005-london-bombings-fast-facts/index.html, accessed May 24, 2020 Search PubMed.
- J. P. Hutchinson, C. J. Evenhuis, C. Johns, A. A. Kazarian, M. C. Breadmore and M. Macka,
et al., Identification of inorganic improvised explosive devices by analysis of postblast residues using portable capillary electrophoresis instrumentation and indirect photometric detection with a light-emitting diode, Anal. Chem., 2007, 79(18), 7005–7013 CrossRef CAS.
- M. E. Sigman, C. D. Clark, R. Fidler, C. L. Geiger and C. A. Clausen, Analysis of triacetone triperoxide by gas chromatography/mass spectrometry and gas chromatography/tandem mass spectrometry by electron and chemical ionization, Rapid Commun. Mass Spectrom., 2006, 20(19), 2851–2857 CrossRef CAS.
- L. Song and J. E. Bartmess, Liquid chromatography/negative ion atmospheric pressure photoionization mass spectrometry: a highly sensitive method for the analysis of organic explosives, Rapid Commun. Mass Spectrom., 2009, 23(1), 77–84 CrossRef CAS.
- E. Holmgren, H. Carlsson, P. Goede and C. Crescenzi, Determination and characterization of organic explosives using porous graphitic carbon and liquid chromatography-atmospheric pressure chemical ionization mass spectrometry, J. Chromatogr. A, 2005, 1099(1–2), 127–135 CrossRef CAS.
- X. Pan, B. Zhang, K. Tian, L. E. Jones, J. Liu and T. A. Anderson,
et al., Liquid chromatography/electrospray ionization tandem mass spectrometry analysis of octahydro-1,3,5,7-tetranitro-1,3,5,7-tetrazocine (HMX), Rapid Commun. Mass Spectrom., 2006, 20(14), 2222–2226 CrossRef CAS.
- J. M. Perr, K. G. Furton and J. R. Almirall, Gas chromatography positive chemical ionization and tandem mass spectrometry for the analysis of organic high explosives, Talanta, 2005, 67(2), 430–436 CrossRef CAS.
- L. Barron and E. Gilchrist, Ion chromatography-mass spectrometry: a review of recent technologies and applications in forensic and environmental explosives analysis, Anal. Chim. Acta, 2014, 806, 27–54 CrossRef CAS.
-
P. Herbert, V. Barry, P. Chris, L. Douglas, J. Robert, S. Kannan, et al., Determination of perchlorate in drinking water using inline column concentration/matrix elimination ion chromatography with suppressed conductivity detection, US EPA method, 2005, p. 314 Search PubMed.
-
S. Wendelken, D. Munch, B. Pepich, D. Later and C. Pohl, Method 331.0, Determination of Perchlorate in Drinking Water by Liquid Chromatography Electrospray Ionization Mass Spectrometry. Revision 1.0, US Environmental Protection Agency, Cincinnati, OH, USA, 2005 Search PubMed.
-
E. Hedrick, T. Behymer, R. Slingsby and D. Munch, Method 332.0, Determination of Perchlorate in Drinking Water by Ion Chromatography with Suppressed Conductivity and Electrospray Ionization Mass Spectrometry, Revision 1.0, Report EPA/600/R-05, 2005 Search PubMed.
-
S. Nawaz, An Investigation of Perchlorate Levels in Fruit and Vegetables Consumed in the UK, FERA, 2016, pp. 1–39 Search PubMed.
- C. A. Groom, A. Halasz, L. Paquet, S. Thiboutot, G. Ampleman and J. Hawari, Detection of nitroaromatic and cyclic nitramine compounds by cyclodextrin assisted capillary electrophoresis quadrupole ion trap mass spectrometry, J. Chromatogr. A, 2005, 1072(1), 73–82 CrossRef CAS.
- K. Brensinger, C. Rollman, C. Copper, A. Genzman, J. Rine and I. Lurie,
et al., Novel CE-MS technique for detection of high explosives using perfluorooctanoic acid as a MEKC and mass spectrometric complexation reagent, Forensic Sci. Int., 2016, 258, 74–79 CrossRef CAS.
-
H. H. Weetall, Biosensor technology what? where? when? and why?, Elsevier, 1996 Search PubMed.
-
J. Yinon, Counterterrorist detection techniques of explosives, Elsevier, 2011 Search PubMed.
- A. Poling, B. J. Weetjens, C. Cox, N. W. Beyene and A. Sully, Using giant African pouched rats (Cricetomys gambianus) to detect landmines, The Psychological Record, 2010, 60(4), 715–728 CrossRef.
-
Humanitarian demining mine detection and sensors, 2011 IEEE International Symposium on Industrial Electronics, ed. M. K. Habib, IEEE, 2011 Search PubMed.
- J. K. Tomberlin, M. Tertuliano, G. Rains and W. J. Lewis, Conditioned Microplitis croceipes Cresson (Hymenoptera: Braconidae) detect and respond to 2,4-DNT: development of a biological sensor, J. Forensic Sci., 2005, 50(5), 1187–1190 CrossRef CAS.
- T. L. King, F. M. Horine, K. C. Daly and B. H. Smith, Explosives detection with hard-wired moths, IEEE Trans. Instrum. Meas., 2004, 53(4), 1113–1118 CrossRef.
- B. S. W. WB, The nematode Caenorhabditis elegans, Cold Spring Harbor Laboratory, 1988, 1, 988 Search PubMed.
- R. P. Shivers, T. Kooistra, S. W. Chu, D. J. Pagano and D. H. Kim, Tissue-specific activities of an immune signaling module regulate physiological responses to pathogenic and nutritional bacteria in C. elegans, Cell Host Microbe, 2009, 6(4), 321–330 CrossRef CAS.
- S. K. Chaudhuri, S. M. O'Connor, R. L. Gustavson, L. A. Achenbach and J. D. Coates, Environmental factors that control microbial perchlorate reduction, Appl. Environ. Microbiol., 2002, 68(9), 4425–4430 CrossRef CAS.
- L. A. Achenbach, U. Michaelidou, R. A. Bruce, J. Fryman and J. D. Coates,
Dechloromonas agitata gen. nov., sp. nov. and Dechlorosoma suillum gen. nov., sp. nov., two novel environmentally dominant (per)chlorate-reducing bacteria and their phylogenetic position, Int. J. Syst. Evol. Microbiol., 2001, 51(2), 527–533 CrossRef CAS.
- K. G. Byrne-Bailey and J. D. Coates, Complete genome sequence of the anaerobic perchlorate-reducing bacterium Azospira suillum strain PS, J. Bacteriol., 2010, 192(5), 1475–1476 CrossRef CAS.
- A. Oren, R. E. Bardavid and L. Mana, Perchlorate and halophilic prokaryotes: implications for possible halophilic life on Mars, Extremophiles, 2014, 18(1), 75–80 CrossRef CAS.
- T. Matsubara, K. Fujishima, C. W. Saltikov, S. Nakamura and L. J. Rothschild, Earth analogues for past and future life on Mars: isolation of perchlorate resistant halophiles from Big Soda Lake, Int. J. Astrobiol., 2017, 16(3), 218–228 CrossRef CAS.
- J. D. Coates and L. A. Achenbach, Microbial perchlorate reduction: rocket-fuelled metabolism, Nat. Rev. Microbiol., 2004, 2(7), 569–580 CrossRef CAS.
- M. Anbar, S. Guttmann and Z. Lewitus, The mode of action of perchlorate ions on the iodine uptake of the thyroid gland, Int. J. Appl. Radiat. Isot., 1959, 7(2), 87–96 CrossRef CAS.
- O. Eichler and E. Hackenthal, Über ausscheidung und stoffwechsel von perchlorat gemessen mit 36ClO4, Naunyn-Schmiedebergs Arch. Exp. Pathol. Pharmakol., 1962, 243(6), 554–565 CAS.
- W.-H. Feng, K. S. Xue, L. Tang, P. L. Williams and J.-S. Wang, Aflatoxin B1-induced developmental and DNA damage in Caenorhabditis elegans, Toxins, 2017, 9(1), 9 CrossRef.
- S. T. Lamitina, R. Morrison, G. W. Moeckel and K. Strange, Adaptation of the nematode Caenorhabditis elegans to extreme osmotic stress, Am. J. Physiol.: Cell Physiol., 2004, 286(4), C785–C791 CrossRef CAS.
- W. A. Boyd, S. J. McBride, J. R. Rice, D. W. Snyder and J. H. Freedman, A high-throughput method for assessing chemical toxicity using a Caenorhabditis elegans reproduction assay, Toxicol. Appl. Pharmacol., 2010, 245(2), 153–159 CrossRef CAS.
- S. Y. Lee and K. Kang, Measuring the Effect of Chemicals on the Growth and Reproduction of Caenorhabditis elegans, J. Visualized Exp., 2017,(128), e56437 Search PubMed.
- L. Aitlhadj and S. R. Stürzenbaum,
Caenorhabditis elegans in regenerative medicine: a simple model for a complex discipline, Drug Discovery Today, 2014, 19(6), 730–734 CrossRef.
- D. M. Schreinemachers, A. J. Ghio, J. R. Sobus and M. A. Williams, Perchlorate exposure is associated with oxidative stress and indicators of serum iron homeostasis among NHANES 2005–2008 subjects, Biomarker Insights, 2015, 10, BMI.S20089 CrossRef.
- X. Zhao, P. Zhou, X. Chen, X. Li and L. Ding, Perchlorate-induced oxidative stress in isolated liver mitochondria, Ecotoxicology, 2014, 23(10), 1846–1853 CrossRef CAS.
- Y. Liu, Q. Zhou, X. Xie, D. Lin and L. Dong, Oxidative stress and DNA damage in the earthworm Eisenia fetida induced by toluene, ethylbenzene and xylene, Ecotoxicology, 2010, 19(8), 1551–1559 CrossRef CAS.
- B. Shao, L. Zhu, M. Dong, J. Wang, J. Wang and H. Xie,
et al., DNA damage and oxidative stress induced by endosulfan exposure in zebrafish (Danio rerio), Ecotoxicology, 2012, 21(5), 1533–1540 CrossRef CAS.
- E.-M. Ha, C.-T. Oh, Y. S. Bae and W.-J. Lee, A direct role for dual oxidase in Drosophila gut immunity, Science, 2005, 310(5749), 847–850 CrossRef CAS.
- K.-A. Lee, S.-H. Kim, E.-K. Kim, E.-M. Ha, H. You and B. Kim,
et al., Bacterial-derived uracil as a modulator of mucosal immunity and gut-microbe homeostasis in Drosophila, Cell, 2013, 153(4), 797–811 CrossRef CAS.
- V. Chávez, A. Mohri-Shiomi and D. A. Garsin, Ce-Duox1/BLI-3 generates reactive oxygen species as a protective innate immune mechanism in Caenorhabditis elegans, Infect. Immun., 2009, 77(11), 4983–4989 CrossRef.
-
D. H. Kim, Signaling in the innate immune response, WormBook, 2015 Search PubMed.
- E. S. Gilchrist, P. N. Nesterenko, N. W. Smith and L. P. Barron, Organic solvent and temperature-enhanced ion chromatography-high resolution mass spectrometry for the determination of low molecular weight organic and inorganic anions, Anal. Chim. Acta, 2015, 865, 83–91 CrossRef CAS.
- TWGFEX (Technical Working Group for Fire and Explosives), Laboratory Explosion Group: Standards & Protocols Committee, Recommended Guidelines for Forensic Identification of Intact Explosives, Revision #8.
Footnotes |
† Current address: Kuwait University, College of Science, Kuwait. |
‡ Current address: Environmental Research Group, School of Public Health, Faculty of Medicine, Imperial College London, London, SW7 2AZ. |
|
This journal is © The Royal Society of Chemistry 2021 |
Click here to see how this site uses Cookies. View our privacy policy here.