Broadband white-light emission in a one-dimensional organic–inorganic hybrid cadmium chloride with face-sharing CdCl6 octahedral chains†
Received
6th October 2020
, Accepted 24th November 2020
First published on 27th November 2020
Abstract
One dimensional (1D) hybrid metal halides with core–shell quantum wire structures can display superior photoluminescence (PL) properties, e.g. high photoluminescence quantum yield and long lifetime; however, the single component white-light emissions in 1D hybrid systems composed of face-sharing octahedral chains have been only rarely reported. Herein, we report on a new 1D organic–inorganic hybrid cadmium chloride, (2cepiH)CdCl3 (2cepi = 1-(2-chloroethyl)piperidine), containing face-sharing CdCl6 octahedral chains. This incredible 1D Cd-based halide exhibited a bright white-light upon ultraviolet photoexcitation, which had an ultra-high color rendering index of 89 along with the CIE chromaticity coordinates of (0.27, 0.31). The fundamental mechanism of white-light emission was assigned to the synergistic emissions of free excitons and self-trapped excitons, as confirmed by the temperature-dependent PL spectra. To our knowledge, it is the first time that self-trapped exciton states are reported in 1D Cd-based hybrid materials with broadband white-light emission. Furthermore, both the experimental and the calculated results confirmed the semiconducting behavior of (2cepiH)CdCl3, and the band structure was determined by the inorganic frameworks. Our work provides a new idea to design novel single component white-light emitters.
Introduction
Recently, solid-state lighting (SSL) is one of the most important technologies significantly boosting our daily life because of energy saving, high efficiency, compactness, and environmental friendliness.1,2 Currently, the commercially available SSLs are typically white light-emitting diodes (LEDs); they can be realized via a blue LED coated with a yellow phosphor or an ultraviolet LED integrated with red, green, and blue phosphors.3–6 Yet, these strategies to achieve white-light emission possess many deficiencies, including efficiency losses for self-absorption, changes in emission color for the variant in degradation rates of individual components, and poor color rendition.3,4 Therefore, the development of single-component emitters stimulates the scientific community to synthesize white light-emitting materials with high stability, excellent color rendering ability, and high luminescence efficiency.
Organic–inorganic hybrid metal halides are expected to serve as ideal candidates for SSL applications owing to their structural diversity, improved stability, low cost, and easy process.7–13 It is said that the reduction of structural dimensionality increases the band gap and exciton binding energy for the strong dielectric and quantum confinement, which generally allows miscellaneous electric and optical properties.6,14–18 Typically, striking photophysical characteristics, referring to the ultrabroad and highly Stokes-shifted luminescence with pure white-light chromaticity, promote the rapid development of low-dimensional hybrid metal halides. Up to now, two-dimensional layered perovskites, (EDBE)[PbBr4] (EDBE = 2,2′-(ethylenedioxy)bis(ethylammonium))19 and α-(DMEN)PbBr4 (DMEN = 2-(dimethylamino)ethylamine),20 one-dimensional (1D) chain-typed metal halides, (2,6-dmpz)3Pb2Br10 (2,6-dmpz = 2,6-dimethylpiperazine),21 C4N2H14PbBr4,22 (TDMP)PbBr4 (TDMP = trans-2,5-dimethylpiperazine),2 and C5H14N2PbCl4·H2O,23 as well as zero-dimensional clusters, (C9NH20)6Pb3Br12,24 have been reported, and all of them showed unique broadband luminescence that was ascribed to the exciton self-trapping25 associated with the structural distortion in inorganic frameworks.26,27 In addition, metal ions with a d10 configuration, for instance Zn2+, Cd2+ and Hg2+, can also act as luminescence centres to achieve a highly efficient broadband white-light emission.6,9 Moreover, previous studies on the structure–property relationship in low dimensional hybrid metal halides proved that 1D compounds with core–shell quantum wire structures could display superior photoluminescence (PL) properties, e.g. a high photoluminescence quantum yield (PLQY) and a long lifetime,2,5,21,22,28,29 which really sparked our interest in 1D systems. In bulk assemblies of 1D hybrid metal halides, the six-coordinated octahedra generally form polyanion chains through corner-sharing, edge-sharing, face-sharing, or mixed connection fashions.26 Remarkably, the broadband white-light emission on the 1D structures constructing by corner- or/and edge-sharing octahedra has been extensively investigated in previous studies.2,21,22,30 However, the single component white-light emission in 1D hybrid systems composed of face-sharing octahedral chains has been only rarely reported.
In this context, we report on a new 1D organic–inorganic hybrid cadmium chloride, (2cepiH)CdCl3 (2cepi = 1-(2-chloroethyl) piperidine) (1), characterized by the face-sharing octahedral chains. This outstanding material, which had an ultra-high color rendering index (CRI) of 89 along with CIE chromaticity coordinates of (0.27, 0.31), was able to emit a bright white light upon ultraviolet photoexcitation. The fundamental mechanism of white-light emission was assigned to the combination of free-excitonic emission and self-trapped excitonic emission, which was confirmed by temperature-dependent PL spectra. To our knowledge, it is the first time that self-trapped exciton states are reported in the 1D Cd-based hybrid materials with broadband white-light emission. Furthermore, both the experimental and the calculated results confirmed the semiconducting behavior of 1, and the band structure was determined via the inorganic Cd–Cl framework.
Experimental section
Materials
All chemicals, including CdCl2·2.5H2O (99.0%, Kermel), 1-(2-chloroethyl)piperidine hydrochloride ((2cepiH)Cl, 98%, Aladdin), and methanol (AR, Tianjin Fengchuan Chemical Reagent Co., Ltd), were commercially available and directly used without any further purification. For the synthesis of 1, the mixture of 1.841 g (10 mmol) CdCl2·2.5H2O and 2.284 g (10 mmol) (2cepiH)Cl was dissolved in 20 mL of the mixed solvent containing methanol and deionized water with a volume ratio of 2.5
:
1. The bulk crystals of 1 were obtained by slow evaporation of the synthesized clear aqueous solution at room temperature after a few days.
X-ray crystallographic study
Single-crystal X-ray diffraction (XRD) data of 1 were collected using an Xcalibur, Eos, Gemini EOS diffractometer with Mo Kα radiation (λ = 0.71073 Å) at 150 K. The SAINT software was used for the integration of the diffraction profiles. The structure was solved by direct methods, and it was refined by the full-matrix least-squares method with minimization on F2 (SHELXL-97). All non-hydrogen atoms were anisotropically refined. All hydrogen atoms of organic molecules were generated theoretically onto the specific carbon and nitrogen atoms and refined isotropically with fixed thermal factors. Crystallographic data and structure refinements of 1 are summarized in Table S1 (ESI†), and the selected Cd–Cl bond distances and angles are listed in Table S2 (ESI†).
Characterization
The PL data of 1, including the excitation spectrum, the emission spectrum, the temperature-dependent PL spectra, and the time-resolved decay data, were measured using an Edinburgh FLS920 fluorescence spectrometer with a picosecond pulsed diode laser. The PLQY was achieved by incorporating an integrating sphere into the FLS980 spectrofluorometer. The PLQY was calculated based on the equation: ηQE = IS/(ER − ES), in which IS represents the emission spectra of the compound, ER is the spectra of the excitation light of the empty integrated sphere, and ES is the excitation spectra of the excited sample. The CIE chromaticity coordinates and CRI were calculated using the CIE calculator software based on the emission spectra. The UV-vis absorption curves of 1 were recorded on a TU-1901 Ultraviolet spectrometer at room temperature, in which BaSO4 was used as the standard reference. The corresponding optical band gap was evaluated as a function of the Kubelka–Munk equation: α/S = (1 − R)2/2R.
Fabrication of the device
Crystal samples of 1 were fully ground into powder. Then, 0.1 g powder samples of 1 were uniformly mixed with 150 mL of ethyl acetate. The suspension obtained was carefully coated onto a commercial ultraviolet LED lamp, which was placed in air for 2 h to evaporate the solvent. The fabricated device was dried at 333 K in a vacuum oven for 24 h to remove the residual solvent.
Density functional theory calculations
First-principle calculations were carried out using the Vienna ab initio simulation package 5.4 (VASP 5.4)31,32 within the MedeA software platform.33 The electron–ion interactions were treated via the projector augmented wave (PAW) method.34 The electronic exchange and correlation effects were approximated with the local density approximation (LDA).35 The valence electron configurations included in the calculations were Cd (5s24d10), Cl (3s23p5), C (2s22p2), N (2s22p3), and H (1s1). The cutoff energy of plane-wave basis was set to 400 eV. Electronic states were smeared using a Gaussian scheme36 with a width of 0.1 eV. The lattice constants and atomic positions were both fully relaxed until electronic self-consistent and Hellmann–Feynman forces on atoms converge were reached at 10−6 eV and 0.005 eV Å−1, respectively. The reciprocal space integration was performed by sampling the Brillouin zone with the Monkhorst–Pack37k-point grid with densities of 0.2 Å−1.
Result and discussion
Crystal structure descriptions
Colorless block crystals of 1 were obtained from an aqueous solution through slow evaporation for several days (Fig. S1, ESI†), see Experimental section for details), and the phase purity was confirmed by powder XRD (Fig. S2, ESI†) and Fourier transform infrared analysis (Fig. S3, ESI†). Specifically, the infrared characteristic peak of the C–Cl bond from the (2cepiH)+ cation was clearly observed at around 667 cm−1. Moreover, thermogravimetric measurements exhibited that 1 had a high thermal stability up to 473 K (Fig. S4, ESI†).
Single-crystal XRD analyses demonstrated that 1 crystallized in the monoclinic centrosymmetric space group P21/c with the point group of C2h (Table S1, ESI†), and the asymmetric unit comprised two Cd2+ ions, three Cl− ions and one protonated (2cepiH)+ cation (Fig. 1a). Note that both Cd2+ ions lay on a special position with a crystallographic inversion center (1 bar). For the inorganic moieties, both Cd atoms were coordinated by six bridged Cl atoms forming distorted CdCl6 octahedra, which are further connected by face-sharing into a 1D infinite chain frameworks along the [100] direction (Fig. 1b). This type of chain based on face-sharing octahedra has been extensively used to exploit high-performance molecular ferroelectrics.38,39 Moreover, the distances between two adjacent Cd sites in the inorganic anionic chain were 3.3591 Å, which were comparable to those of other reported 1D cadmium chloride hybrids.38,40,41 Furthermore, all of the Cd–Cl bond lengths ranged from 2.5771(9) to 2.7145(8) Å, and the range of Cl–Cd–Cl bond angles that represent the inclined angle between one metal center and two adjacent Cl atoms was 81.96(3)–98.04(3)° (Fig. S5 and Table S2, ESI†). The three axial Cl–Cd–Cl bond angles for the single octahedra CdCl6 in our reported data were 180° (Table S2, ESI†), and all of the Cd atoms in the inorganic polyanion [CdCl3]n− chains were arranged along a straight line (Fig. S5, ESI†). In addition, Fig. 1c shows 3D packing patterns of 1 along a axis, in which each of 1D infinite [CdCl3]n− chains was surrounded by the four adjacent organic cations. The crystal structure of 1 could be viewed as the bulk assembly of core–shell quantum wires, where the organic shells surrounded the core cadmium chloride wires through intermolecular interactions.
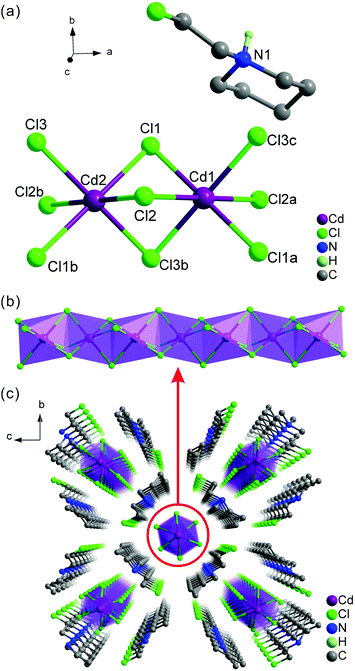 |
| Fig. 1 (a) Asymmetric unit of 1 and the coordination geometry of Cd atoms. (b) 1D inorganic infinite [CdCl3]n− chain composed of face-sharing CdCl6 octahedra. (c) Packing diagram of 1 along the [100] direction. | |
Photophysical properties
The absorbance and the photoluminescence of our reported 1D hybrid cadmium chloride were examined to investigate the photophysical properties (see Experimental section for details). The optical absorbance spectrum of 1 in Fig. 2a showed an absorption peak at 330 nm (3.76 eV), similar to that of the reported Cd-based hybrid halides,9,42–44 which could be assigned to the excitons confined into the [CdCl3]n− inorganic chain, and could be attributed to the electronic transition from the top of the valence band including Cl(3p) orbitals to the bottom of the conduction band made of the Cd(5s) level.9,42–44 Then, upon excitation at 360 nm, 1 exhibited two emission peaks spanning the entire visible spectrum, which contained a higher-energy peak at 451 nm and another broadband located at 550 nm similar to the previous observations in the zero-dimensional Cd-based halides, (HMEDA)CdBr4 (HMEDA = hexamethylenediamine).45 Significantly, it is the first time that broadband white-light emission was observed in 1D Cd-based hybrid materials. As shown in Fig. 2b, the CIE 1931 chromaticity coordinates of the broadband emission of 1 were determined to be (0.27, 0.31), which was just located in the white-light region (Fig. 2b, inset). 1 showed an ultra-high CRI of 89, superior to the CRI of the most commercial light-emitting diodes, which satisfies the demand for color-critical lighting devices.4,23 Moreover, the PLQY of 1 was measured to be 1.88%, which was comparable to those of previous reported 1D hybrid white-light materials, C5H14N2PbCl4·H2O (1%)23 and [4-methylpiperidinium]2SbCl5 (1%).46 We measured the time-resolved PL decay of two emission peaks in 1. Fig. 2c revealed the PL decay data for the broadband at 550 nm, which was characterized as a double exponential with a short lifetime of 1.23 ns (τ1) and a long lifetime of 6.44 ns (τ2). The resulting average lifetime (τ) obtained according to τ = τ1α1 + τ2α2, where the occupancy of each decay time was 57.63% (α1) and 42.37% (α2), respectively, was 3.44 ns. This value was comparable to those of previously reported 1D metal halide hybrids23,46,47 because of the populated self-trapped excitons (STEs). The measured lifetime for the narrow band at 451 nm was 1.52 ns (Fig. S6, ESI†). To confirm the white-light emission of this hybrid further, we coated a thin layer of the sample onto an ultraviolet LED lamp with an emission wavelength of 360–365 nm (see Experimental section for details). Fig. 2d shows that 1 was able to emit a bright white light upon illumination. It is known that stability is a critical issue that should be taken seriously for the applications of organic–inorganic metal halide hybrids, especially for solid-state lighting.14 Our reported hybrid material displayed excellent phase purity exposed to ambient condition for two months, which was proved by powder XRD measurements (Fig. S2, ESI†). The corresponding PL intensity showed a slight decrease unlike the original emission for the as-synthesized samples (Fig. S7, ESI†). These results unambiguously illustrated that the better stability of our reported material confers its application in stable electronic devices.
 |
| Fig. 2 (a) Absorption spectrum and emission spectrum of 1. (b) CIE coordinates of 1. Inset: PL photograph at the exciton wavelength of 360 nm (c) Time-resolved PL decay and fitting of 1 at 550 nm. (d) Photographs of ultraviolet LED lamps (top) and LED coated with a thin layer (bottom) of 1, in the off and on states. | |
To get an insight into the origin of broadband white-light emission in 1, we firstly measured the PL spectra of our reported hybrids with different sample size to verify the influence of surface defects.48 The measured data in Fig. 3a showed that the emission spectra of the cm-sized bulk single crystal (Fig. S1, ESI†) were almost identical to that of the μm-sized particles (Fig. S8, ESI†), which evidenced that the white-light emission is independent from the particle size. Therefore, we argued that white-light emission in our samples was not associated with surface defects, but may originate from intrinsic trap states. In this regard, it is now broadly recognized that the high energy narrowband emission derives from excitonic emission, while the low energy broadband emission stems from the STEs resulting from the highly distorted layered frameworks.
 |
| Fig. 3 (a) Emission spectra of 1 for bulk crystals (black line) and microscale sample (red line). (b) Temperature-dependent emission spectra of 1. All of the selected excitation wavelengths are 360 nm. (c) Schematic diagram of PL mechanism between FEs and STEs dependent upon the temperature. | |
Previous studies on the optical properties of pure CdX2 (X = Cl, Br, I) and their halide solid solutions showed that CdX2 showed a strong luminescence observed in the wavelength range of 326–620 nm with peak positions depending on the nature of the halogen, the temperature and the excitation wavelength.49–51 Fluorescence emission behaviors of reported Cd-based hybrid halides were very similar to those of CdX2.42,52–54 Based on these previous literatures, we concluded that the high-energy emission peak at 451 nm for our reported material (Fig. 2a) was possibly because of the radiative transition of photoinduced excitons confined into the [CdCl3]n− inorganic chain.9
Then, previous investigations on the optical properties of low dimensional hybrid perovskites have found that structural deformations of the inorganic lattice within low dimensional hybrid perovskites played a crucial role in the carrier localization, the formation of STEs and the subsequent broadband emission.55 To structurally understand the emissive properties of 1, we quantitatively evaluated the deformation degree of the cadmium chloride octahedra (Δd) based on the length of the Cd–Cl bond lengths using the following equation.
where
dn is the six individual Cd–Cl bond distances, and
d stands for the mean Cd–Cl bond length. The calculated values of Δ
d were 2.58 × 10
−4 for Cd(1) and 5.15 × 10
−4 for Cd(2), which were comparable with that of the reported for hybrid lead halide perovskite with broadband fluorescence, (mpz)
2Pb
3Br
10 (∼4.5 × 10
−4, mpz = 1-methylpiperazine),
21 which indicated that both CdCl
6 octahedra owned a moderate degree of distortion. Also, we compared the Raman spectra of the pure organic chlorate salts and as-synthesized cadmium chloride hybrids (see ESI,
† for details). We noted that besides small changes in the relative intensities of the peaks, new vibrational modes appear in (2cepiH)Cl when co-crystallized with CdCl
2 (Fig. S9, ESI
†). This implied that (2cepiH)
+ was forced into a different conformation because of the greater intermolecular interaction with inorganic polyanion chains, which led to the distortion of the Cd–Cl frameworks.
55 Therefore, we could speculate that the distorted inorganic octahedra lattice and the specific quantum wire structure in
1 may contribute to the electron–phonon coupling and favor to the exiton self-trapping, which was responsible for the broadband located at 550 nm in
Fig. 2a.
To further verify the above speculation on the fundamental mechanism of broadband emission in 1, we performed the temperature-dependent PL spectra (Fig. 3b). It was found that variable-temperature emission spectra of 1 showed a major contribution from the broad emission upon cooling. Specifically, the integrated intensity of the broad emission increased rapidly with the testing temperature decreasing, and it was accompanied by the full width at half maximum getting narrow. This photoluminescence evolution with the temperature illustrated that the broadband emission originated from the exiton self-trapping because of the electron–phonon coupling, and meanwhile at a lower temperature, the thermal energy was insufficient to de-trap carries from the STE states to free exiton (FE) states (Fig. 3c).56 This is the first report of STE states that were observed in the 1D Cd-based hybrid materials with broadband white-light emission. We further evaluated the STE binding energy according to the following Arrhenius equation:57
where
I0 is the integrated PL intensity at room temperature,
kB is the Boltzmann constant, Δ
E is the STE binding energy, and
A is the pre-exponential factor. The calculated Δ
E was 48.5 meV (Fig. S10, ESI
†), which was smaller than the reported two-dimensional Cd-based halide.
57 Then, we also noted that the FEs emission at higher energy did not trend to fade away,
21 but showed a slight increase of the intensity, suggesting that the distorted inorganic lattices in
1 did not completely prevent the STE states from tunnelling back to the FE states (
Fig. 3c).
21 Therefore, the white-light emission in
1 at ambient condition could be ascribed to the combination of free-excitonic emission and STEs emission.
Semiconductor properties
Besides, we carried out the solid-state UV–vis absorption spectroscopy of 1 at room temperature (see Experimental section for details) to explore the semiconductor properties. As shown in Fig. 4a, the optical bandgap of 1 was estimated to be 3.35 eV through the Tauc equation, which was an indicator of a wide bandgap semiconductor. The characteristic photocatalysis capability of 1 was further confirmed by performing transient short-circuit photocurrent tests (Fig. S11, ESI†). Furthermore, we calculated the band structure and partial density of states by using the first-principles density functional theory method (see Experimental Section for details). Fig. 4b revealed that both valence band maximum (VBM) and conduction band minimum (CBM) were located at G point, indicating that 1 was a direct bandgap semiconductor. The calculated band gap was about 3.95 eV, which was slightly larger than the experimental value (Fig. 4a). The partial density of states of 1 in Fig. 4c indicated that CBM and VBM were composed of Cd 5s and Cl 3p orbits, respectively. Moreover, the Cl 3s and C 2s states overlapped at ∼−15.6 eV, and the Cl 3p and C 2p states overlapped in the ranges of both −6.5–−2.5 eV and 4.6–8.7 eV, which indicated the interaction between C and Cl atoms in organic cation (2cepiH)+. No overlap between C and Cl orbits in the vicinity of VBM (−1.7–0 eV) was observed. Therefore, we considered that the band structure of 1 was determined by the inorganic frameworks.
 |
| Fig. 4 (a) Diffuse reflectance spectrum, (b) band structure, and (c) partial density of states of 1. | |
Conclusions
In summary, a new 1D organic–inorganic hybrid cadmium chloride, (2cepiH)CdCl3, was reported and structurally characterized by face-sharing CdCl6 octahedral chains. 1 exhibited a bright white-light emission upon ultraviolet photoexcitation, and possessed an ultra-high CRI of 89 and CIE chromaticity coordinates of (0.27, 0.31). The temperature-dependent PL spectra demonstrated that the white-light emission stemmed from the radiative combination of FEs and STEs confined in the inorganic Cd–Cl frameworks. Moreover, 1 was a direct bandgap semiconductor evidenced by the experimental and the calculated results, and its band structures were composed of Cd 5s orbits (CBM) and Cl 3p orbits (VBM) from inorganic lattices. Our work represents the first broadband white-light emission in 1D Cd-based hybrid materials, and provides a design solution to explore single component white-light emitters with more brilliant photophysical properties.
Conflicts of interest
The authors declare no competing financial interests.
Acknowledgements
This work was financially supported by the Natural Science Foundation of China (21871167), the 1331 Project of Shanxi Province, Science and Technology Innovative Project in Colleges and Universities of Shanxi Province (2019L0451) and the SXNU Startup. The authors would like to thank Dr Linfeng Liang from Shanxi University for measuring the PLQY, and Qiang Liu from Chemtest Lab (http://www.chemtest.cn) for measuring the temperature-dependent emission spectra.
Notes and references
- J. Luo, X. Wang, S. Li, J. Liu, Y. Guo, G. Niu, L. Yao, Y. Fu, L. Gao, Q. Dong, C. Zhao, M. Leng, F. Ma, W. Liang, L. Wang, S. Jin, J. Han, L. Zhang, J. Etheridge, J. Wang, Y. Yan, E. H. Sargent and J. Tang, Nature, 2018, 563, 541–545 CrossRef CAS PubMed.
- R. Gautier, F. Massuyeau, G. Galnon and M. Paris, Adv. Mater., 2019, 31, 1807383 CrossRef PubMed.
- E. R. Dohner, E. T. Hoke and H. I. Karunadasa, J. Am. Chem. Soc., 2014, 136, 1718–1721 CrossRef CAS PubMed.
- S. Wang, L. Li, Z. Sun, C. Ji, S. Liu, Z. Wu, S. Zhao, A. Zeb, M. Hong and J. Luo, J. Mater. Chem. C, 2017, 5, 4731–4735 RSC.
- C. Sun, Y.-H. Guo, Y. Yuan, W.-X. Chu, W.-L. He, H.-X. Che, Z.-H. Jing, C.-Y. Yue and X.-W. Lei, Inorg. Chem., 2020, 59, 4311–4319 CrossRef CAS PubMed.
- A. Yangui, R. Roccanova, T. M. McWhorter, Y. Wu, M.-H. Du and B. Saparov, Chem. Mater., 2019, 31, 2983–2991 CrossRef CAS.
- M. D. Smith and H. I. Karunadasa, Acc. Chem. Res., 2018, 51, 619–627 CrossRef CAS PubMed.
- L. N. Quan, F. P. García de Arquer, R. P. Sabatini and E. H. Sargent, Adv. Mater., 2018, 30, 1801996 CrossRef PubMed.
- A. Yangui, S. Pillet, E.-E. Bendeif, A. Lusson, S. Triki, Y. Abid and K. Boukheddaden, ACS Photonics, 2018, 5, 1599–1611 CrossRef CAS.
- Z. Song, J. Zhao and Q. Liu, Inorg. Chem. Front., 2019, 6, 2969–3011 RSC.
- C. Sun, Y.-H. Guo, S.-S. Han, J.-Z. Li, K. Jiang, L.-F. Dong, Q.-L. Liu, C.-Y. Yue and X.-W. Lei, Angew. Chem., Int. Ed., 2020, 59, 16465–16469 CrossRef CAS PubMed.
- C.-Q. Jing, J. Wang, H.-F. Zhao, W.-X. Chu, Y. Yuan, Z. Wang, M.-F. Han, T. Xu, J.-Q. Zhao and X.-W. Lei, Chem. – Eur. J., 2020, 26, 10307–10313 CrossRef CAS PubMed.
- C. Sun, K. Jiang, M.-F. Han, M.-J. Liu, X.-K. Lian, Y.-X. Jiang, H.-S. Shi, C.-Y. Yue and X.-W. Lei, J. Mater. Chem. C, 2020, 8, 11890–11895 RSC.
- C. Zhou, H. Lin, Q. He, L. Xu, M. Worku, M. Chaaban, S. Lee, X. Shi, M.-H. Du and B. Ma, Mater. Sci. Eng., R, 2019, 137, 38–65 CrossRef.
- C. Ji, S. Wang, L. Li, Z. Sun, M. Hong and J. Luo, Adv. Funct. Mater., 2019, 29, 1805038 CrossRef.
- L.-J. Xu, S. Lee, X. Lin, L. Ledbetter, M. Worku, H. Lin, C. Zhou, H. Liu, A. Plaviak and B. Ma, Angew. Chem., Int. Ed., 2020, 59, 14120–14123 CrossRef CAS PubMed.
- E. P. Booker, T. H. Thomas, C. Quarti, M. R. Stanton, C. D. Dashwood, A. J. Gillett, J. M. Richter, A. J. Pearson, N. J. L. K. Davis, H. Sirringhaus, M. B. Price, N. C. Greenham, D. Beljonne, S. E. Dutton and F. Deschler, J. Am. Chem. Soc., 2017, 139, 18632–18639 CrossRef CAS PubMed.
- G. Wu, C. Zhou, W. Ming, D. Han, S. Chen, D. Yang, T. Besara, J. Neu, T. Siegrist, M.-H. Du, B. Ma and A. Dong, ACS Energy Lett., 2018, 3, 1443–1449 CrossRef CAS.
- E. R. Dohner, A. Jaffe, L. R. Bradshaw and H. I. Karunadasa, J. Am. Chem. Soc., 2014, 136, 13154–13157 CrossRef CAS PubMed.
- L. Mao, Y. Wu, C. C. Stoumpos, M. R. Wasielewski and M. G. Kanatzidis, J. Am. Chem. Soc., 2017, 139, 5210–5215 CrossRef CAS PubMed.
- L. Mao, P. Guo, M. Kepenekian, I. Hadar, C. Katan, J. Even, R. D. Schaller, C. C. Stoumpos and M. G. Kanatzidis, J. Am. Chem. Soc., 2018, 140, 13078–13088 CrossRef CAS PubMed.
- Z. Yuan, C. Zhou, Y. Tian, Y. Shu, J. Messier, J. C. Wang, L. J. van de Burgt, K. Kountouriotis, Y. Xin, E. Holt, K. Schanze, R. Clark, T. Siegrist and B. Ma, Nat. Commun., 2017, 8, 14051 CrossRef CAS PubMed.
- Y. Peng, Y. Yao, L. Li, Z. Wu, S. Wang and J. Luo, J. Mater. Chem. C, 2018, 6, 6033–6037 RSC.
- J. Zhou, M. Li, L. Ning, R. Zhang, M. S. Molokeev, J. Zhao, S. Yang, K. Han and Z. Xia, J. Phys. Chem. Lett., 2019, 10, 1337–1341 CrossRef CAS PubMed.
- R. T. Williams and K. S. Song, J. Phys. Chem. Solids, 1990, 51, 679–716 CrossRef CAS.
- D. Cortecchia, J. Yin, A. Petrozza and C. Soci, J. Mater. Chem. C, 2019, 7, 4956–4969 RSC.
- G. Zhou, B. Su, J. Huang, Q. Zhang and Z. Xia, Mater. Sci. Eng., R, 2020, 141, 100548 CrossRef.
- H. Barkaoui, H. Abid, S. Zelewski, J. Urban, M. Baranowski, A. Mlayah, S. Triki, P. Plochocka and Y. Abid, Adv. Opt. Mater., 2019, 7, 1900763 CrossRef CAS.
- W.-F. Zhang, W.-J. Pan, T. Xu, R.-Y. Song, Y.-Y. Zhao, C.-Y. Yue and X.-W. Lei, Inorg. Chem., 2020, 59, 14085–14092 CrossRef CAS PubMed.
- H. Lin, C. Zhou, J. Neu, Y. Zhou, D. Han, S. Chen, M. Worku, M. Chaaban, S. Lee, E. Berkwits, T. Siegrist, M.-H. Du and B. Ma, Adv. Opt. Mater., 2019, 7, 1801474 CrossRef.
- G. Kresse and D. Joubert, Phys. Rev. B: Condens. Matter Mater. Phys., 1999, 59, 1758–1775 CrossRef CAS.
- G. Kresse and J. Furthmüller, Comput. Mater. Sci., 1996, 6, 15–50 CrossRef CAS.
- E. Wimmer, M. Christensen, V. Eyert, W. Wolf, D. Reith, X. Rozanska, C. Freeman and P. Saxe, J. Korean Ceram. Soc., 2016, 53, 263–272 CrossRef CAS.
- P. E. Blöchl, Phys. Rev. B: Condens. Matter Mater. Phys., 1994, 50, 17953–17979 CrossRef PubMed.
- J. P. Perdew and Y. Wang, Phys. Rev. B: Condens. Matter Mater. Phys., 1992, 45, 13244–13249 CrossRef PubMed.
- M. Methfessel and A. T. Paxton, Phys. Rev. B: Condens. Matter Mater. Phys., 1989, 40, 3616–3621 CrossRef CAS PubMed.
- J. D. Pack and H. J. Monkhorst, Phys. Rev. B: Solid State, 1977, 16, 1748–1749 CrossRef.
- W.-Q. Liao, D. Zhao, Y.-Y. Tang, Y. Zhang, P.-F. Li, P.-P. Shi, X.-G. Chen, Y.-M. You and R.-G. Xiong, Science, 2019, 363, 1206 CrossRef CAS PubMed.
- Z.-X. Wang, Y. Zhang, Y.-Y. Tang, P.-F. Li and R.-G. Xiong, J. Am. Chem. Soc., 2019, 141, 4372–4378 CrossRef CAS PubMed.
- Y.-Y. Tang, Y. Ai, W.-Q. Liao, P.-F. Li, Z.-X. Wang and R.-G. Xiong, Adv. Mater., 2019, 1902163 CrossRef PubMed.
- A. Zeb, Z. Sun, T. Khan, M. A. Asghar, Z. Wu, L. Li, C. Ji and J. Luo, Inorg. Chem. Front., 2017, 4, 1485–1492 RSC.
- M. S. Lassoued, M. S. M. Abdelbaky, A. Lassoued, A. Gadri, S. Ammar, A. Ben Salah and S. García-Granda, J. Mol. Struct., 2017, 1141, 390–399 CrossRef CAS.
- A. Kessentini, M. Belhouchet, J. J. Suñol, Y. Abid and T. Mhiri, J. Mol. Struct., 2013, 1039, 207–213 CrossRef CAS.
- A. Jellibi, I. Chaabane and K. Guidara, Phys. E, 2016, 79, 167–172 CrossRef CAS.
- C. Sun, W.-L. He, M.-J. Liu, W.-J. Pan, L.-F. Dong, G. Chen, G.-D. Liu and X.-W. Lei, Chem. – Asian J., 2020, 15, 3050–3058 CrossRef CAS PubMed.
- A. Khan, A. Zeb, L. Li, W. Zhang, Z. Sun, Y. Wang and J. Luo, J. Mater. Chem. C, 2018, 6, 2801–2805 RSC.
- D. Li, W. Wu, S. Wang, X. Zhang, L. Li, Y. Yao, Y. Peng and J. Luo, J. Mater. Chem. C, 2020, 8, 6710–6714 RSC.
- M. J. Bowers, J. R. McBride and S. J. Rosenthal, J. Am. Chem. Soc., 2005, 127, 15378–15379 CrossRef CAS PubMed.
- H. Nakagawa, K. Hayashi and H. Matsumoto, J. Phys. Soc. Jpn., 1977, 43, 1655–1663 CrossRef CAS.
- H. Matsumoto and H. Nakagawa, J. Lumin., 1979, 18–19, 19–22 CrossRef CAS.
- M. Kitaura, H. Nakagawa, K. Fukui, M. Fujita, T. Miyanaga and M. Watanabe, J. Electron Spectrosc. Relat. Phenom., 1996, 79, 175–178 CrossRef CAS.
- A. Ohnishi, T. Yamada, T. Yoshinari, I. Akimoto, K. Kan'no and T. Kamikawa, J. Electron Spectrosc. Relat. Phenom., 1996, 79, 163–166 CrossRef CAS.
- K. Chu, Y.-H. Zhou, J.-L. Song and C. Zhang, Polyhedron, 2017, 131, 22–26 CrossRef CAS.
- R. Roccanova, W. Ming, V. R. Whiteside, M. A. McGuire, I. R. Sellers, M.-H. Du and B. Saparov, Inorg. Chem., 2017, 56, 13878–13888 CrossRef CAS PubMed.
- D. Cortecchia, S. Neutzner, A. R. Srimath Kandada, E. Mosconi, D. Meggiolaro, F. De Angelis, C. Soci and A. Petrozza, J. Am. Chem. Soc., 2017, 139, 39–42 CrossRef CAS PubMed.
- S. Wang, Y. Yao, J. Kong, S. Zhao, Z. Sun, Z. Wu, L. Li and J. Luo, Chem. Commun., 2018, 54, 4053–4056 RSC.
- B. Luo, D. Liang, S. Sun, Y. Xiao, X. Lian, X. Li, M. D. Li, X. C. Huang and J. Z. Zhang, J. Phys. Chem. Lett., 2020, 11, 199–205 CrossRef CAS PubMed.
Footnote |
† Electronic supplementary information (ESI) available: Details of method and addition figures and tables. CCDC 2011052. For ESI and crystallographic data in CIF or other electronic format see DOI: 10.1039/d0tc04731g |
|
This journal is © The Royal Society of Chemistry 2021 |
Click here to see how this site uses Cookies. View our privacy policy here.