DOI:
10.1039/D0TC02381G
(Paper)
J. Mater. Chem. C, 2020,
8, 11540-11545
Supramolecular oligourethane gel as a highly selective fluorescent “on–off–on” sensor for ions†
Received
18th May 2020
, Accepted 16th July 2020
First published on 22nd July 2020
Abstract
Stimuli-responsive supramolecular gels (SRSGs) are an important class of smart materials. It is of practical importance to develop an SRSG which can both detect and remove toxic metal ions. We have designed and synthesized an aggregation induced emission (AIE)-active oligourethane (OU) gelator which self-assembles into a supramolecular gel (OUG), through hydrogen-bonding, π–π stacking and van der Waals interactions. By taking advantage of the weak and dynamic nature of these non-covalent bonds, OUG shows stimuli-response to multiple factors. Importantly, OUG has the capacity for real-time detection and high selectivity for Fe3+, HSO4− and F−. The lowest detection limits are in the range of 5.89 × 10−9 to 8.17 × 10−8 M, indicating high sensitivity. More importantly, OUG is shown to adsorb and separate Fe3+ from aqueous solution, with an absorbing rate of up 97.5%. A simple writing board was fabricated, which could be written repeatedly and reused. OUG acts as a reversible and recyclable “on–off–on” fluorescence sensor via competitive cation–π and cation–anion interactions. OUG has great potential as an environmentally sustainable probe for ions.
Introduction
Stimuli-responsive supramolecular gels (SRSGs) have the ability to respond to a chemical substance,1 light,2 heat,3 pH4 or pressure.5 They have been applied in chemical sensors,6 displays,7 drug deliveries8 and other fields.9 Responsive behavior can be achieved by a gel–sol state transition or by changing the luminescence.7,10 The latter response works by changing the gel's fluorescence intensity or color, and can be free from the influence of temperature,2 pH,11 an oxidizing agent,8 and other factors.12 Therefore luminescence detection has considerably higher sensitivity and more reliable real-time response.13–15 Traditional conjugated gelators usually suffer from aggregation-caused quenching (ACQ), which sharply weakens the emission behavior in aggregation or solid states, thereby limiting their applications.16 The emergence of polymers/oligomers with aggregation-induced emission (AIE) properties has been a breakthrough in the field.17,18 In addition to their excellent emission characteristics, AIE-active supramolecular gels show strong absorption activity and synergistic effects because of their large contact area with analytes.19
Recently, our group has explored AIE-active poly/oligourethane-based unconventional luminophores, which are without typical polycyclic π-conjugated units. These materials show obvious advantages like environmental friendliness, excellent hydrophilicity, chain flexibility, ease of synthesis and structural versatility compared with traditional organic luminescent materials.20–22
Fe3+ is an indispensable element in the process of oxygen uptake and metabolism.23 However, an excess of Fe3+ might cause pathological diseases like cancer and organ dysfunction.24 F− and HSO4− also play essential roles in human biological processes,25,26 although undue fluoride may cause kidney problems, dental and skeletal fluorosis.27 HSO4− can produce poisonous SO42− under acidic conditions, which will stimulate the skin and eyes and can even cause respiratory paralysis.28 Thus, methods to efficiently detect these ions have received extensive attention. The established detection techniques, such as inductively coupled plasma spectroscopy,29 high performance liquid chromatography (HPLC)30 and electrochemical methods,31 all require tedious sample preparations, sophisticated instruments and professional operators. However, fluorescent sensor molecules, which convert and amplify the signals into a visible and easily recognized fluorescent output, offer a more significant practical method.6,32
Herein, we report an AIE-active supramolecular oligourethane gel (OUG) and demonstrate its usage as a specific Fe3+ sensor in an aqueous environment. The material is based on the following design criteria: (i) inserting benzophenone into an oligourethane (OU) backbone provides C
O units with prominent hydrogen-bonding sites for self-assembly, and formation of oxygen clusters, which could enhance fluorescent emission.33 (ii) Inserting linear 1,6-diisocyanatohexane offers strong van der Waals interactions among alkyl chains, limiting internal rotation of the molecular chains, thereby blocking the non-radiative pathways and favoring AIE. Taking advantage of the rich hydrogen bond acceptors/donors (C
O/N–H) among the oligourethane skeleton,34–41 we introduced solvents with hydrogen-bonding acceptor units (C
O or S
O) as external crosslinking agents, to self-assemble a supramolecular oligourethane gel (OUG) relying on multiple hydrogen bonds.
Results and discussion
Synthesis and characterization
The OU was synthesized through a facile procedure as shown in Scheme S1 (ESI†), by the reaction of 4,4′-dihydroxybenzophenone, hexamethylene diisocyanate and DABCO in anhydrous tetrahydrofuran and end-capping with polyethylene glycol monomethyl ether to give a viscous solution. The product was purified by a counter precipitation method. 1H NMR and FTIR characterization data are given in Fig. 1a and in the ESI,† confirming the structure of OU. The Mn value of 1814 g mol−1, calculated from the 1H NMR data, established that OU should be classified as an oligomer.42 The FTIR spectra (Fig. S1, ESI†) showed absorbance bands at 3323 cm−1 and 1706 cm−1, assigned to stretching vibrations of N–H and C
O, indicating the formation of amide bonds. Absorbance bands at 2936 cm−1 and 2860 cm−1 correspond to v(–CH2–) and at 1163 cm−1 correspond to v(C–O–C) stretching vibrations. The UV-Vis absorption spectrum of OU in the solid-state (Fig. S2, ESI†) showed a major peak at 277 nm from a π–π* transition of the aromatic rings.43
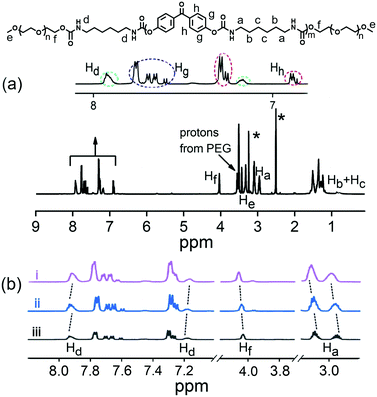 |
| Fig. 1 (a) 1H NMR spectrum of OU in DMSO-d6 (* indicates peaks from the solvent and water); H-atom labeling is shown on the structure of OU. (b) The partial 1H NMR spectra of OU in DMSO-d6 at different concentrations: (i) 0.925 mM, (ii) 3.7 mM, and (iii) 25.0 mM. | |
Self-assembly gelation
OU spontaneously self-assembles in certain solvents (notably dimethyl formamide and dimethylsulfoxide) transforming into a supramolecular gel (Table S1, ESI†). The lowest critical gelation concentration (CGC) of OU is 4% (w/v, 10 mg mL−1 = 1%), and the corresponding gel–sol transition temperature (Tgel) is 85–87 °C. In order to gain an insight into the self-assembly mechanism, 1H NMR, FTIR, XRD and urea addition experiments were conducted. 1H NMR spectra were recorded for different concentrations of OU in DMSO-d6 (Fig. 1b). The Ha and Hf proton signals are shifted ca. 0.04 and 0.03 ppm upfield compared to pure OUG upon adding 25 mM Fe3+. Meanwhile, the signals of protons Hd (the NH groups) shifted slightly downfield ca. 0.01 ppm.44 These results confirmed the H-bonding interactions between amide groups and van der Waals interactions between alkyl chains. Comparing the FTIR data before and after gelation (Fig. S1, ESI†), the N–H stretching absorbance bands of OUG are broader and move to significantly higher wavenumbers (3323 to 3361 cm−1) in the solid state compared to the gel state: these data suggest hydrogen bonds play a critical role in the gelation process.45,46
It is well known that adding urea, which has a high propensity to form hydrogen bonds, can disrupt existing hydrogen bonds in a supramolecular structure.47,48 Accordingly, adding urea (10 equiv.) into OUG and heating the gel, led to the formation of a sol. It was observed that after adding urea, the sol did not revert back to gel, even when the OUG-urea mixture was cooled at 15 °C for several days, indicating that the gelation is driven by hydrogen bonds among OU molecular chains (Fig. 2a). Besides, the X-ray diffraction (XRD) peaks of OUG 2θ = 20.54°, 23.22° corresponding to d-spacings of 4.32 Å and 3.83 Å, respectively, also indicated the presence of π–π stacking interactions (Fig. S11b, ESI†), further promoting the self-assembly behavior. OUG showed weak fluorescence in the sol state, however, after transforming to the gel state, the emission intensity of OUG at 439 nm increased 6 times (Fig. S3, ESI†), indicating that OU is an AIE-active gelator.49
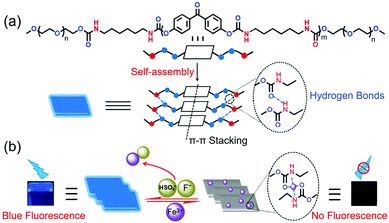 |
| Fig. 2 (a) Structure of OU and its self-assembly gelation process. (b) Schematic diagram for the ion sensing processes of OUG. | |
Stimuli-responsive behaviors
OUG exhibits a high selectivity to Fe3+ over other metal ions. By monitoring the change of fluorescence, we investigated the recognition characteristics of OUG towards metal ions. Using nitrate salts as the cation sources, an aqueous metal ion solution of Na+, Ca2+, Co2+, Cu2+, Mn2+, Ni2+, Cr3+, La3+, Fe3+, Sr2+, Ce3+, Ag+, Al3+, Mg2+, Cd2+, Pb2+ or Fe2+ (c = 0.2 M) was added to the OUG to generate the corresponding metal-gels.50–53 As shown in Fig. 3a and c, initially, the OUG had a strong blue fluorescence emission. When different metal ions were added, only Fe3+ quenched the fluorescence of OUG. Thus, the OUG could effectively and selectively detect Fe3+. To further evaluate the sensitivity of OUG for Fe3+, the fluorescence behavior of OUG was monitored by continuous titrations with Fe3+. As shown in Fig. S5a (ESI†), with the increasing addition of Fe3+ (0–1.1 equiv.), the emission intensity of the corresponding metal-gels (OUFeG) at 439 nm gradually decreased. The limit of detection (LOD) of OUG towards Fe3+ was calculated to be 5.89 × 10−9 M based on the 3δ/S method54 (Fig. S4 and S5a, ESI†), confirming the high selectivity of OUG as a sensor for Fe3+ compared with other reported sensor systems (Table S2, ESI†). The high selectivity of OUG to Fe3+ is attributed to two reasons: firstly, unpaired electrons in Fe3+ cause a paramagnetic effect, prompting energy dissipation of excited states through non-radiative pathways.55 Secondly, the high ionic strength of Fe3+ could easily induce the transfer of π-electrons from the urethane backbone to Fe3+ through cation–π interactions.56 both of these effects will cause the fluorescence quenching of OUG.
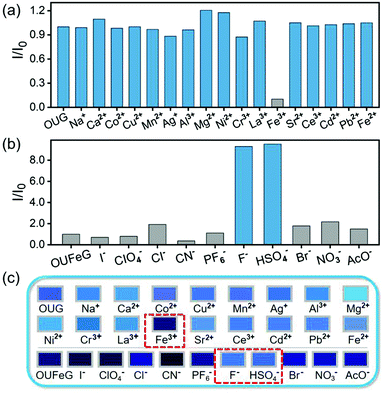 |
| Fig. 3 (a) Fluorescence responses of OUG (in DMF, 10% (w/v)) toward Fe3+ and other metal ions at room temperature. (b) Fluorescence responses of OUG (in DMF, 10% (w/v)) toward F−, HSO4− and other anions at room temperature. All data represent the fluorescence intensity ratio I/I0 (I and I0 are the final fluorescence intensity and initial fluorescence intensity for the gel with each ion tested at 384 nm). (c) Photos of OUG fluorescence responses to various cations and anions. | |
The response of OUFeG towards anions was further investigated, using tetrabutylammonium salts as the anion sources, adding aqueous solutions of anions including HSO4−, ClO4−, I−, CN−, PF6−, F−, Cl−, Br−, NO3−, AcO− (c = 0.2 M) to OUFeG.57–60 As shown in Fig. 3b and c, when HSO4− and F− were added the emission at 439 nm recovered immediately, whereas the other anions did not lead to a comparable fluorescence recovery of OUFeG. These phenomena confirmed the excellent selectivity of OUFeG towards HSO4− and F−. This selectivity is explained by the strong coordinating capacity of HSO4− and F− with Fe3+, in contrast to (metal-free) OUG. These two anions could competitively bind to Fe3+ in OUFeG and induce the re-emission of OUG. Titration experiments evaluated the sensing efficiency of OUFeG to HSO4− and F−. Limit of detection (LOD) values were calculated according to the 3δ/S method to be 8.17 × 10−8 M for F− and 1.16 × 10−8 M for HSO4− (Fig. S5b and Fig. S7–S9, ESI†). Notably the LOD values of OUFeG are lower than many other reported F− and HSO4− fluorescence sensors (Table S2, ESI†). The gel OUG selectively senses Fe3+, and the metal-gel OUFeG selectively responds to F− and HSO4−. This property makes gel OUG and metal-gel OUFeG act as an efficient “on–off–on” fluorescence sensor controlled by Fe3+, HSO4− or F− with excellent reversibility. A simple regeneration treatment verified the recyclability of OUG. An anion solution (F− or HSO4−, 2 × 10−5 mol L−1; 10 mL) was added into metal-gel OUFeG, stirring the mixture for 5 min, centrifuging and recycling OUG for again detecting ions. As shown in Fig. S10 (ESI†), after five consecutive cycles, the intensity of the OUG signal is essentially unchanged, indicating the excellent recyclability and reversibility of the OUG for the detection of Fe3+ and HSO4− or F−.
Mechanism of cation–anion sensor
When adding aqueous solution of Fe3+ into OUG, Fe3+ can diffuse in the solution. Due to the high ionic strength of Fe3+, the π-electrons in the urethane groups of OUG are easily induced to form cation–π interactions quenching the fluorescence. The recognition mechanisms of OUG to Fe3+ were investigated via a combination of 1H NMR, FTIR, XRD and SEM analysis. In the 1H NMR titration experiments, with the increasing amount of Fe3+ added into OU, protons Hd, Hf and Ha all displayed clear upfield shifts (Fig. 4a), indicating that the OUG combined with Fe3+via cation–π interactions between the urethane groups and Fe3+.56,61 As Fig. 4b shows, by adding F− or HSO4− (3.7 mM) into a sample of OU (with 2 equiv. Fe3+), all the protons recovered the chemical shifts as in the original OU: Hd (from 7.85 to 7.93 ppm and 7.09 to 7.19 ppm), Hf (from 3.94 to 4.03 ppm) and Ha (from 3.01 to 3.09 ppm and 2.87 to 2.95 ppm), which indicated the cation–anion interactions between Fe3+ and F− or HSO4− could release the π-electrons of urethane groups, thus recovering the fluorescence of OUG.
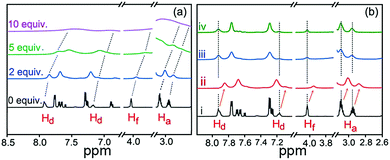 |
| Fig. 4 (a) 1H NMR titration spectra (298 K) of 0.925 mM OU with various equivalents of Fe3+ in DMSO-d6 solution. (b) 1H NMR spectra of (i) OU (ii) OUFeG (iii) OUFeG + HSO4− and (iv) OUFeG + F−. | |
In the FTIR experiments (Fig. S11a, ESI†), when Fe3+ was added into OUG to form OUFeG, the stretching absorbance bands of N–H, C
O and C–O–C shifted from 3361 cm−1, 1708 cm−1 and 1161 cm−1 to 3480 cm−1, 1673 cm−1 and 1158 cm−1 respectively, which further confirmed that Fe3+ interacts with π-electrons of the urethane groups, thus influencing H-bonds between the amide groups.6,57 After the addition of F− or HSO4− into the OUFeG, the C
O, N–H and C–O–C all reverted to their initial positions (Fig. S11a, ESI†). These observations suggested that F− and HSO4− competitively bound to Fe3+ rather than to OUG. Moreover, the XRD peaks of OUG moved with adding Fe3+ into OUG, and recovered when F− or HSO4− was added into OUFeG (Fig. S11b, ESI†).
To get further insight into the mechanism of cation–anion sensing, as shown in Fig. 5, the SEM studies were carried out. Fig. 5a demonstrates that gel OUG shows a lamellar stacking structure with a smooth surface. This structure was converted into a honeycomb structure in the metal-gel OUFeG (Fig. 5b), while in gel OUFeG + HSO4− and OUFeG + F−, the image again showed a smooth lamellar stacking structure (Fig. 5c and d). Such morphological change is attributed to the cation–π interactions between OUG and Fe3+, breaking hydrogen bonding between the OUG chains and modifying the supramolecular structure.57,61 After adding F− or HSO4− into OUFeG, π-electrons of OUG were released, hydrogen bonds are rebuilt and the morphology is recovered. These experimental results indicated that the fluorescence of OUG can be reversibly switched by Fe3+ (off) and then by F− or HSO4− (on), through repeated competition between cation–π and cation–anion interactions (Fig. 2b).
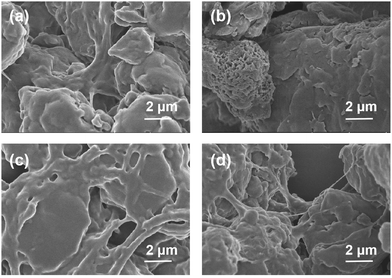 |
| Fig. 5 SEM images of (a) OUG; (b) OUFeG; (c) OUFeG + HSO4−; (d) OUFeG + F−. | |
Application in the rapid removal of Fe3+
The development of new sorbents for the sensing and extraction of metal ions from environmental and biological samples is of current importance.62,63 The performance of OUG to effectively remove Fe3+ from aqueous solution was analyzed by atomic absorption spectrometry (Table S3, ESI†). Specifically, OUG (0.2 g) was added to a dilute aqueous solution of Fe3+ (1 × 10−5 mol L−1 in 10 mL water) and the mixture was stirred for 10 min. The precipitate was separated by centrifuging at 5000 rpm for 5 min and the supernatant was retained, the residual concentration of Fe3+ was about 2.5 × 10−7 mol L−1. Experimental results demonstrated that 97.5% of Fe3+ could be efficiently removed by OUG even from extremely dilute solutions, which indicated the excellent adsorption capacity of OUG towards Fe3+.6,56,57,64 As shown in Fig. S6 (ESI†), adsorption tests of OUG toward Fe3+ in the pH range from 4 to 10 showed that the adsorption of OUG toward Fe3+ showed excellent stability at different pH conditions (in the range of 92.1–97.5%). OUG can selectively detect with high-efficiency and rapidly remove toxic Fe3+, offering potential practical applications in combating heavy metal ion pollution and in environmental remediation.
Application as a writing display material
Based on the above-mentioned “on–off–on” properties, the OUG has a great potential as a rewritable fluorescent display material. As a proof-of-concept a rewritable board was constructed (Fig. 6). The detailed steps are described as follows: (i) OUG sol (10%) was poured onto a clean quartz plate surface and dried under ambient conditions to give a film emitting strong blue fluorescence under ultraviolet radiation (365 nm). (ii) Writing the symbol “Fe” on the film with a brush dipped in aqueous Fe3+ solution (0.3 M), a dark “Fe” image was clearly displayed due to the fluorescence quenching effect of Fe3+ on OUG. (iii) The whole OUG film was transformed into a non-fluorescent display board by brushing with Fe3+ solution. (iv) Two new letters “S” and “F” could be written again with the same brushing method using HSO4− and F− solutions (0.3 M), respectively. Visually, the letters emitted blue fluorescence under a UV lamp. Combining these practically very simple processes with the excellent recyclability of OUG (discussed above; Fig. S10, ESI†) means that OUG has promising applications as a fluorescent writing display material.
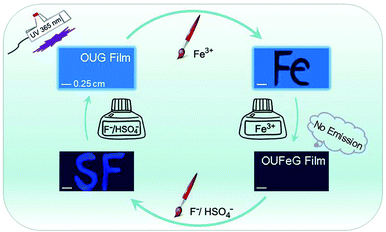 |
| Fig. 6 An erasable writing board of OUG based on its ion-controlled fluorescent switching properties. | |
Conclusion
In conclusion, a novel supramolecular AIE gel, OUG, was designed and synthesized by a straightforward “one-pot” procedure. The dynamic and reversible noncovalent interactions endow OUG with distinct advantages of a reversible and highly sensitive response to Fe3+, HSO4−, and F−, acting as an “on–off–on” fluorescent sensor for these cationic and anionic species. Importantly, OUG can absorb up to 97.5% Fe3+ from a water environment. This rapid, simple, low cost and highly sensitive material has great potential for practical applications in intelligent sensing, handling heavy metal ion pollution and environmental remediation.
Conflicts of interest
There are no conflicts to declare.
Acknowledgements
The work was funded by NSFC (No. 51473028), the key scientific and technological project of Jilin province (20150204011GX, 20160307016GX, 20190701010GH), the Development and Reform Commission of Jilin province (20160058, 2020C035-5), Open Project of State Key Laboratory of Supramolecular Structure and Materials (sklssm202039). M. R. B. thanks EPSRC grant EL/L02621X/1 for funding.
Notes and references
- L.-J. Chen, G.-Z. Zhao, B. Jiang, B. Sun, M. Wang, L. Xu, J. He, Z. Abliz, H. Tan, X. Li and H.-B. Yang, J. Am. Chem. Soc., 2014, 136, 5993–6001 CrossRef CAS PubMed.
- S.-H. Hsiao and S.-h. Hsu, ACS Appl. Mater. Interfaces, 2018, 10, 29273–29287 CrossRef CAS PubMed.
- J. B. Beck and S. J. Rowan, J. Am. Chem. Soc., 2003, 125, 13922–13923 CrossRef CAS PubMed.
- M. Zhang, D. Xu, X. Yan, J. Chen, S. Dong, B. Zheng and F. Huang, Angew. Chem. Int. Ed., 2012, 51, 7011–7015 CrossRef CAS PubMed.
- J. Lai, H. Zhou, Z. Jin, S. Li, H. Liu, X. Jin, C. Luo, A. Ma and W. Chen, ACS Appl. Mater. Interfaces, 2019, 11, 26412–26420 CrossRef CAS PubMed.
- Y.-M. Zhang, W. Zhu, W.-J. Qu, K.-P. Zhong, X.-P. Chen, H. Yao, T.-B. Wei and Q. Lin, Chem. Commun., 2018, 54, 4549–4552 RSC.
- Q. Lin, B. Sun, Q.-P. Yang, Y.-P. Fu, X. Zhu, Y.-M. Zhang and T.-B. Wei, Chem. Commun., 2014, 50, 10669–10671 RSC.
- X. Cheng, Y. Jin, T. Sun, R. Qi, H. Li and W. Fan, Colloids Surf., B, 2016, 141, 44–52 CrossRef CAS PubMed.
- M. Ikeda, T. Tanida, T. Yoshii, K. Kurotani, S. Onogi, K. Urayama and I. Hamachi, Nat. Chem., 2014, 6, 511–518 CrossRef CAS PubMed.
- Z.-Y. Li, Y. Zhang, C.-W. Zhang, L.-J. Chen, C. Wang, H. Tan, Y. Yu, X. Li and H.-B. Yang, J. Am. Chem. Soc., 2014, 136, 8577–8589 CrossRef CAS PubMed.
- K. Manokruang and D. S. Lee, Macromol. Biosci., 2013, 13, 1195–1203 CrossRef CAS PubMed.
- T. Tanaka, Phys. Rev. Lett., 1978, 40, 820–823 CrossRef CAS.
- Y. Chang, J. Fu, K. Yao, B. Li, K. Xu and X. Pang, Dyes Pigm., 2019, 161, 331–340 CrossRef CAS.
- B.-J. Wang, W.-K. Dong, Y. Zhang and S. F. Akogun, Sens. Actuators, B, 2017, 247, 254–264 CrossRef CAS.
- X. Liu, T. Chen, F. Yu, Y. Shang, X. Meng and Z.-R. Chen, Macromolecules, 2020, 53, 1224–1232 CrossRef CAS.
- C.-W. Zhang, B. Ou, S.-T. Jiang, G.-Q. Yin, L.-J. Chen, L. Xu, X. Li and H.-B. Yang, Polym. Chem., 2018, 9, 2021–2030 RSC.
- P. Q. Nhien, W.-L. Chou, T. T. K. Cuc, T. M. Khang, C.-H. Wu, N. Thirumalaivasan, B. T. B. Hue, J. I. Wu, S. P. Wu and H.-C. Lin, ACS Appl. Mater. Interfaces, 2020, 12, 10959–10972 CrossRef PubMed.
- Y. Bao, E. Guégain, V. Nicolas and J. Nicolas, Chem. Commun., 2017, 53, 4489 RSC.
- R. Hu, A. Qin and B. Z. Tang, Prog. Polym. Sci., 2020, 100, 101176 CrossRef CAS.
- N. Jiang, G.-F. Li, B.-H. Zhang, D.-X. Zhu, Z.-M. Su and M. R. Bryce, Macromolecules, 2018, 51, 4178–4184 CrossRef CAS.
- N. Jiang, G. Li, W. Che, D. Zhu, Z. Su and M. R. Bryce, J. Mater. Chem. C, 2018, 6, 11287–11291 RSC.
- N. Jiang, D. Zhu, Z. Su and M. R. Bryce, J. Mater. Chem. C, 2020, 8, 5137–5142 RSC.
- K. P. Carter, A. M. Young and A. E. Plamer, Chem. Rev., 2014, 114, 4564–4601 CrossRef CAS PubMed.
- Y. Fang, J. Tan, H. Choi, S. Lim and D.-H. Kim, Sens. Actuators, B, 2018, 259, 155–161 CrossRef CAS.
- Y. Li, Y. Duan, J. Zheng, J. Li, W. Zhao, S. Yang and R. Yang, Anal. Chem., 2013, 85, 11456–11463 CrossRef CAS PubMed.
- U. Haldar and H.-i. Lee, Polym. Chem., 2018, 9, 4882–4890 RSC.
- L. Li, Y. Ji and X. Tang, Anal. Chem., 2014, 86, 10006–10009 CrossRef CAS PubMed.
- H. J. Kim, S. Bhuniya, R. K. Mahajan, R. Puri, H. Liu, K. C. Ko, J. Y. Lee and J. S. Kim, Chem. Commun., 2009, 7128–7130 RSC.
- M. Türkmen, A. Türkmen, Y. Tepe, Y. Töre and A. Ateş, Food Chem., 2009, 113, 233–237 CrossRef.
- V. Fernández and G. Winkelmann, Biometals, 2005, 18, 53–62 CrossRef PubMed.
- B. Bansod, T. Kumar, R. Thakur, S. Rana and I. Singh, Biosens. Bioelectron., 2017, 94, 443–455 CrossRef CAS PubMed.
- X.-Y. Xu and B. Yan, ACS Appl. Mater. Interfaces, 2015, 7, 721–729 CrossRef CAS PubMed.
- Q. Zhou, B. Cao, C. Zhu, S. Xu, Y. Gong, W. Z. Yuan and Y. Zhang, Small, 2016, 12, 6586–6592 CrossRef CAS PubMed.
- D. Zhang, Y. Zhang, Y. Fan, M.-N. Rager, V. Guérineau, L. Bouteiller, M.-H. Li and C. M. Thomas, Macromolecules, 2019, 52, 2719–2724 CrossRef CAS.
- K. A. Houton, G. M. Burslem and A. J. Wilson, Chem. Sci., 2015, 6, 2382–2388 RSC.
- S. Sami, E. Yildirim, M. Yurtsever, E. Yurtsever, E. Yilgor, I. Yilgor and G. L. Wilkes, Polymer, 2014, 55, 4563–4576 CrossRef CAS.
- D. H. Merino, A. T. Slark, H. M. Colquhoun, W. Hayes and I. W. Hamley, Polym. Chem., 2010, 1, 1263–1271 RSC.
- P. J. Woodward, D. H. Merino, B. W. Greenland, I. W. Hamley, Z. Light, A. T. Slark and W. Hayes, Macromolecules, 2010, 43, 2512–2517 CrossRef CAS.
- D. Hermida-Merino, G. E. Newby, I. W. Hamley, W. Hayes and A. Slark, Soft Matter, 2015, 11, 5799–5803 RSC.
- T. S. Babra, M. Wood, J. S. Godleman, S. Salimi, C. Warriner, N. Bazin, C. R. Siviour, I. W. Hamley, W. Hayes and B. W. Greenland, Eur. Polym. J., 2019, 119, 260–271 CrossRef CAS.
- J. P. Sheth, D. B. Klinedinst, G. L. Wilkes, I. Yilgor and E. Yilgor, Polymer, 2005, 46, 7317–7322 CrossRef CAS.
- T. Mondal, J. Sarkar and S. Ghosh, Chem. – Eur. J., 2016, 22, 10930–10936 CrossRef CAS PubMed.
- Q. Wan, M. Liu, L. Mao, R. Jiang, D. Xu, H. Huang, Y. Dai, F. Deng, X. Zhang and Y. Wei, Mater. Sci. Eng., C, 2017, 72, 352–358 CrossRef CAS PubMed.
- J. A. Sáez, B. Escuder and J. F. Miravet, Chem. Commun., 2010, 46, 7996–7998 RSC.
- P. Maiti, G. Radhakrishnan, P. Aruna and G. Ghosh, Macromol. Symp., 2006, 241, 51–59 CrossRef CAS.
- M.-T. Leiendecker, C. J. Licht, J. Borghs, D. J. Mooney, M. Zimmermann and A. Böker, Macromol. Rapid Commun., 2018, 39, 1700711 CrossRef PubMed.
- M. R. Molla and S. Ghosh, Chem. – Eur. J., 2012, 18, 9860–9869 CrossRef CAS PubMed.
- D. R. Canchi, D. Paschek and A. E. García, J. Am. Chem. Soc., 2010, 132, 2338–2344 CrossRef CAS PubMed.
- X.-M. Jiang, X.-J. Huang, S.-S. Song, X.-Q. Ma, Y.-M. Zhang, H. Yao, T.-B. Wei and Q. Lin, Polym. Chem., 2018, 9, 4625–4630 RSC.
- Q. Lin, X.-M. Jiang, X.-Q. Ma, J. Liu, H. Yao, Y.-M. Zhang and T.-B. Wei, Sens. Actuators, B, 2018, 272, 139–145 CrossRef CAS.
- W. Dong, H. Wu, M. Chen, Y. Shi, J. Sun, A. Qin and B. Z. Tang, Polym. Chem., 2016, 7, 5835–5839 RSC.
- G. Yang, H. Zhang, Y. Wang, X. Liu, Z. Luo and J. Yao, Sens. Actuators, B, 2017, 251, 773–780 CrossRef CAS.
- Q. Lin, B. Sun, Q.-P. Yang, Y.-P. Fu, X. Zhu, T.-B. Wei and Y.-M. Zhang, Chem. – Eur. J., 2014, 20, 11457–11462 CrossRef CAS PubMed.
- D. Dai, Z. Li, J. Yang, C. Wang, J.-R. Wu, Y. Wang, D. Zhang and Y.-W. Yang, J. Am. Chem. Soc., 2019, 141, 4756–4763 CrossRef CAS PubMed.
- S. K. Sahoo, D. Sharma, R. K. Bera, G. Crisponi and J. F. Callan, Chem. Soc. Rev., 2012, 41, 7195–7227 RSC.
- Y.-M. Zhang, W. Zhu, X.-J. Huang, W.-J. Qu, J.-X. He, H. Fang, H. Yao, T.-B. Wei and Q. Lin, ACS Sustainable Chem. Eng., 2018, 6, 16597–16606 CrossRef CAS.
- Y.-M. Zhang, J.-X. He, W. Zhu, Y.-F. Li, H. Fang, H. Yao, T.-B. Wei and Q. Lin, Mater. Sci. Eng., C, 2019, 100, 62–69 CrossRef CAS PubMed.
- H. Xie, F. Zeng, C. Yu and S. Wu, Polym. Chem., 2013, 4, 5416–5424 RSC.
- H. Wan, P. Gu, F. Zhou, H. Wang, J. Jiang, D. Chen, Q. Xu and J. Lu, Polym. Chem., 2018, 9, 3893–3899 RSC.
- G. G. V. Kumar, M. P. Kesavan, G. Sivaraman and J. Rajesh, Sens. Actuators, B, 2018, 255, 3194–3206 CrossRef CAS.
- Q. Lin, P.-P. Mao, Y.-Q. Fan, L. Liu, J. Liu, Y.-M. Zhang, H. Yao and T.-B. Wei, Soft Matter, 2017, 13, 7085–7089 RSC.
- W. A. Wan Ibrahim, L. I. Abd Ali, A. Sulaiman, M. M. Sanagi and H. Y. Aboul-Enein, Crit. Rev. Anal. Chem., 2014, 44, 233–254 CrossRef CAS PubMed.
- M. Kataria, M. Kumar and V. Bhalla, J. Indian Chem. Soc., 2018, 95, 1559–1577 CAS.
- J. Liu, Y.-Q. Fan, S.-S. Song, G.-F. Gong, J. Wang, X.-W. Guan, H. Yao, Y.-M. Zhang, T.-B. Wei and Q. Lin, ACS Sustainable Chem. Eng., 2019, 7, 11999–12007 CAS.
Footnotes |
† Electronic supplementary information (ESI) available: 1H NMR spectra and photophysical properties. See DOI: 10.1039/d0tc02381g |
‡ These authors contributed equally to the preparation of this work. |
|
This journal is © The Royal Society of Chemistry 2020 |