DOI:
10.1039/D0SC04888G
(Edge Article)
Chem. Sci., 2020,
11, 11989-11997
Fluorescent probes for in vitro and in vivo quantification of hydrogen peroxide†
Received
4th September 2020
, Accepted 27th September 2020
First published on 27th October 2020
Abstract
Hydrogen peroxide (H2O2) plays essential roles in redox signaling and oxidative stress, and its dynamic concentration is critical to human health and diseases. Here we report the design, syntheses, and biological applications of HKPerox-Red and HKPerox-Ratio for quantitative measurement of H2O2. Both probes were successfully applied to detect endogenous H2O2 fluxes in living cells or zebrafish, and biological effects of multiple stress inducers including rotenone, arsenic trioxide, and starvation were investigated. As H2O2 is a common by-product for oxidase oxidation, a general assay was developed for ultrasensitive detection of various metabolites (glucose, uric acid, and sarcosine). Moreover, cellular H2O2 measurements were achieved for the first time by combining flow cytometry with live cell calibration. This study provides a pair of unique molecular tools for advanced H2O2 bio-imaging and assay development.
Introduction
Oxygen fuels energy production processes in aerobic organisms through respiration, while partial reduction of oxygen produces reactive oxygen species (ROS) as by-products of oxygen metabolism.1 Among all ROS, hydrogen peroxide (H2O2) stands out as the most significant messenger molecule for redox signaling due to its relatively long half-life and diffusion range.2 Cellular H2O2 is constantly produced with a steady-state concentration (∼0.1 μM) to signal cell growth and proliferation, whereas higher levels of H2O2 (e.g. ∼100 μM) trigger cell growth arrest or apoptosis.2b,3 Quantitative analysis of H2O2 in biological content is key to understanding its multiple roles in redox signaling and oxidative stress. However, there is no method currently available for non-invasive, sensitive, and precise measurement of H2O2 in intact cells, and previous H2O2 quantifications relied heavily on electrochemical analysis or peroxidase-based H2O2 assay, where only extracellular H2O2 secreted from cells could be measured accurately.4
A powerful tool to investigate cellular H2O2 fluxes is fluorimetry (e.g. confocal imaging and flow cytometry) due to its high sensitivity, non-invasiveness and spatio-temporal resolution.5,6 While several genetically encoded H2O2 sensors have been developed for H2O2 detection with unparalleled dynamic subcellular precision, they may suffer from pH sensitivity, poor brightness, limited responses and selectivity toward H2O2.7 Nearly all chemosensors for H2O2 detection are based on small-molecule dyes, including traditional dichloro-dihydro-fluorescein diacetate (DCFH-DA, a commonly used unselective ROS probe) and selective probes, especially activity-based sensing,5c including boronate oxidation,5a,8 Baeyer–Villiger reaction,5b,9 tandem Payne/Dakin reaction,10 and very recently Mislow–Evans rearrangement.11 Those intensity-based probes are very sensitive, but they are prone to signal fluctuations arising from variations in probe uptake, distribution, or even optical inputs. Therefore, ratiometric sensing with an internal standard for calibration is more desirable for quantitative measurement of H2O2,12 however, most of current ratiomeric probes (including both biosensors and chemosensors) still suffer from low selectivity, low sensitivity, as well as slow response, thereby limited biological applications.7,13
To address those challenges, we herein report a pair of new fluorescent probes, HKPerox-Red and HKPerox-Ratio (Scheme 1). Both probes feature excellent selectivity and sensitivity for unambiguous H2O2 detection and quantification in aqueous solution. Moreover, the red emissive HKPerox-Red allows imaging endogenous H2O2 fluxes in living cells and zebrafish embryos, by virtue of its outstanding permeability. HKPerox-Red was further utilized to develop a general in vitro assay for ultrasensitive detection of various biomarkers and respective oxidases (glucose/glucose oxidase, uric acid/urate oxidase, sarcosine/sarcosine oxidase), since H2O2 is quantatitively produced during those enzymatically catalyzed oxidation reactions. As a result of its nanomolar sensitivity, HKPerox-Red could be used for accurate measurement of glucose with 1000 times diluted serum (1 μL to 1 mL), which provides a new method for non-invasive glucose detection with high precision. On the other hand, our novel ratiometric probe HKPerox-Ratio has been successfully applied to detect H2O2 burst induced by arsenic trioxide (As2O3) in multiple leukaemia cell lines. For the first time, intracellular H2O2 in response to starvation is visualized and quantified with HKPerox-Ratio by using both confocal microscopy and flow cytometry, establishing a useful protocol for H2O2 ratiometric analysis with spatio-temporal precision. Our new data confirm the value of HKPerox series for advanced bio-imaging analysis (in vivo imaging, ratiometric imaging, and flow cytometry analysis) as well as the development of ultrasensitive chemical tools to interrogate H2O2-related physiology and pathology.
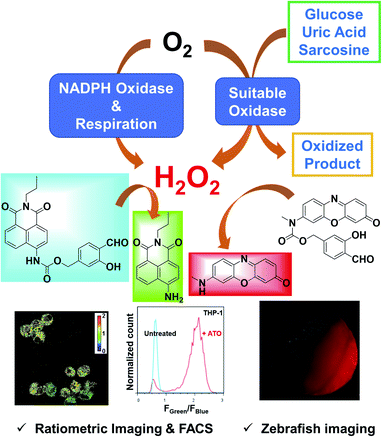 |
| Scheme 1
HKPerox-Red and HKPerox-Ratio for H2O2 bio-imaging and in vitro assay development. | |
Results and discussion
Design and synthesis of HKPerox-Red and HKPerox-ratio
Our general strategy on developing red emissive and ratiometric H2O2 probes is based on modulation of internal charge transfer (ICT) of fluorophores that could be deprotected upon H2O2 mediated Payne/Dakin reaction (activity-based sensing). As shown in Schemes 1 and 2a, an electron-withdrawing carbamate sensing moiety could efficiently interrupt the ICT process in the resorufin derivative, making HKPerox-Red essentially non-fluorescent. However, after H2O2-mediated deprotection, the remaining electron-donating 7-methylamino group could restore the push–pull conjugating system in 3H-phenoxazin-3-one scaffold, releasing a bright red emission. Similarly, HKPerox-Ratio was designed by modulating ICT on a biocompatible 4-amino-1,8-naphthalimide platform (see Scheme 2b):12a,14 before the treatment of H2O2, the electron-withdrawing carbamate linker on the 4-position of 1,8-naphthalimide disrupts the ICT process to produce a blue emission; upon H2O2 mediated deprotection, the carbamate linker is efficiently cleaved to release the electron-donating amine, and the ICT process is restored to provide a bright green emission.
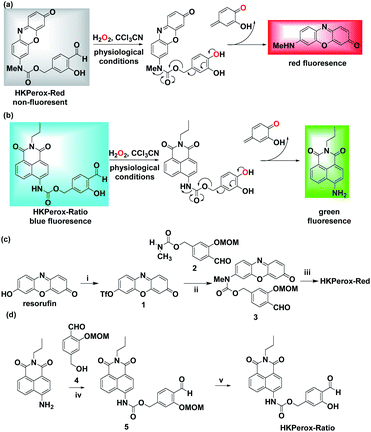 |
| Scheme 2 Design and synthesis of HKPerox-Red and HKPerox-Ratio. Rational design of (a) HKPerox-Red and (b) HKPerox-Ratio for H2O2 sensing based on tandem Payne/Dakin reaction. Synthetic schemes for (c) HKPerox-Red and (d) HKPerox-Ratio. Reagents and conditions: (i) DMF, NaH, PhNTf2, 0 °C, 8 h, 74%; (ii) Pd2(dba)3, xantphos, Cs2CO3, dioxane, 100 °C, 24 h, 57%; (iii) TFA/DCM (v/v = 1 : 1), rt, 2 h, 95%; (iv) DIPEA, triphosgene, toluene, reflux, 1 h; 1, DCM, rt, 3 h, 38%; (v) TFA/DCM (v/v = 1 : 1), rt, 2 h, 92%. | |
Two general synthetic routes were envisioned to prepare HKPerox-Red and HKPerox-Ratio: (1) a general coupling reaction between halogen- or triflate-substituted fluorophores and the carbamate sensing moiety; (2) a general addition reaction between isocyanate-substituted fluorophores and the benzyl alcohol sensing moiety. Specifically, HKPerox-Red was synthesized from commercially available resorufin in three steps: triflation, a cross-coupling between triflate 1 and carbamate 2, and deprotection. HKPerox-Ratio was synthesized according to another approach (Scheme 2d) for amine-containing fluorophore: 4-amino group of 1,8-naphthalimide fluorophore was first converted to an isocyanate by treatment with triphosgene, and the in situ generated isocyanate readily reacted with the benzyl alcohol sensing moiety 4 to form carbamate 5, which was deprotected to afford HKPerox-Ratio.
Reactivity and selectivity of HKPerox-Red and HKPerox-Ratio toward H2O2
With both probes in hand, we first investigated the spectroscopic properties of HKPerox-Red and HKPerox-Ratio in potassium phosphate buffer (0.1 M, pH 7.4, 100 μM CCl3CN). Due to its excellent water-solubility, both probes could form a homogenous solution in the buffer without additional co-solvent. As shown in Fig. 1a, an obvious absorption peak of HKPerox-Red was observed at 580 nm after H2O2 treatment. At the same time, a bright red emission at 602 nm appeared with increasing amounts of H2O2 in a dose-dependent manner (0–100 μM). The resulting fluorescent product was detected as a resorufin derivative by UPLC-MS analysis (Scheme 2a and ESI†), which confirms that tandem Payne/Dakin reaction was successfully extended to this scaffold. As shown in Fig. 1c, the fluorescence intensity at 602 nm was linearly correlated with H2O2 concentration ranging from 0 to 30 μM. The detection limit was estimated to be as low as 4.8 nM (3σ/k) with this standard calibration curve, which could be used as an ultrasensitive assay for H2O2 quantification. The major challenges for H2O2 sensors are actually sensitivity and selectivity: the reactivity of H2O2 is milder than those highly reactive species, thus competing responses from other ROS seem to be inevitable with previously reported strategies. However, HKPerox-Red is highly sensitive toward H2O2, and a >150-fold enhancement in fluorescence intensity was observed upon treatment with H2O2, while other potential competing ROS/RNS including 1O2, ROO˙, TBHP, NO, O2˙−, ·OH, ONOO−, and HOCl, only triggered negligible changes (Fig. 1d).
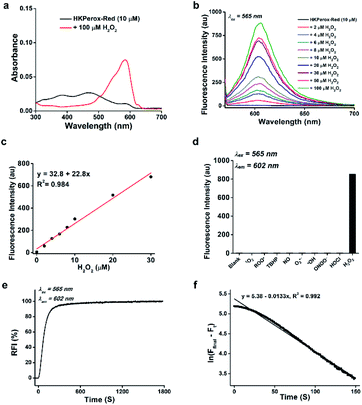 |
| Fig. 1 Chemical characterization of HKPerox-Red (10 μM) in 0.1 M potassium phosphate buffer (pH 7.4, 0.5% DMF, 100 μM CCl3CN). (a) Absorbance spectra of HKPerox-Red before and after treatment with H2O2 (100 μM). (b) Fluorescence emission spectra of HKPerox-Red upon treatment with different amounts of H2O2 (0–100 μM). (c) Fluorescence intensity of HKPerox-Red at 602 nm as a function of H2O2 (0–30 μM). (d) Fluorescence responses of HKPerox-Red toward various reactive oxygen/nitrogen species (ROS/RNS; 100 μM). (e) Time course of HKPerox-Red (1 μM) in buffer treated with 1 mM H2O2. (f) Rate calculation based on pseudo first order kinetics. kobs = 1.3 × 10−2 s−1. | |
In addition, reaction kinetics of HKPerox-Red were investigated. Time courses of fluorescence intensities of HKPerox-Red (10 μM) at 602 nm upon treatment with 100 μM H2O2 were recorded. The reaction was almost completed within 10 min (Fig. S1, ESI†), meaning that a short period of incubation is enough to trigger a significant fluorescence response. At pH 8.0, this reaction could be further accelerated, which is consistent with the mechanism of tandem Payne/Dakin reaction. The reaction rate constant was calculated to be 1.3 × 10−2 s−1, based on a pseudo first order model by mixing 1 μM HKPerox-Red with 1 mM H2O2 (Fig. 1e and f).
As shown in Fig. 2a, the absorption peak at 375 nm of HKPerox-Ratio was shifted to 425 nm upon H2O2 oxidation, which is consistent with restoration of ICT process in this ratiometric probe.12a,14 To our delight, subsequent fluoresence measurments revealed an isosbestic point on the fluorescence spectra of HKPerox-Ratio (Fig. 2b), indicating its clean conversion. The green fluorescent compound was also confirmed by UPLC-MS analysis to be the cleavage product 4-amino-1,8-naphthalimide (Scheme 2b and ESI†). The ratios of fluorescence emission intensities at 540 nm and 475 nm (F540/F475) were proportional to H2O2 concentrations ranging from 0 to 100 μM. The selectivity of HKPerox-Ratio was also tested, which exhibited a 12.7-fold increase in F540/F475 toward H2O2, while other ROS/RNS gave negligible ratio changes (Fig. 2d). This reaction also proceeded rapidly to complete in 30 min (Fig. S2, ESI†). Collectively, these results demonstrate excellent water-solubility, sensitivity, selectivity of HKPerox-Ratio toward H2O2.
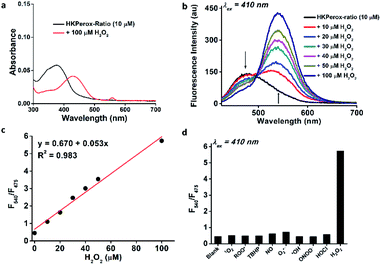 |
| Fig. 2 Chemical characterization of HKPerox-Ratio (10 μM) in 0.1 M potassium phosphate buffer (pH 7.4, 0.1% DMF, 100 μM CCl3CN). (a) Absorbance spectra of HKPerox-Ratio before and after treatment with H2O2 (100 μM). (b) Fluorescence emission spectra of HKPerox-Ratio upon treatment with different amounts of H2O2 (0–100 μM). (c) Ratios of the emission intensities (F540/F475) of HKPerox-Ratio as a function of H2O2 (0–100 μM). (d) Fluorescence responses of HKPerox-Ratio toward ROS/RNS (100 μM). | |
Encouraged by their excellent performances toward H2O2 in chemical system, we further performed biological evaluations of HKPerox-Red and HKPerox-Ratio in living cells. The cytotoxicities of HKPerox-Red and HKPerox-Ratio were assessed in RAW264.7 macrophages, and no obvious toxicity was observed up to 20 μM after 24 h incubation (Fig. S3 and S4, ESI†).
Molecular imaging of endogenous H2O2 production in live cells and in vivo using HKPerox-Red
We went on to apply HKPerox-Red in live cell imaging to detect endogenous H2O2 production with confocal microscopy. RAW264.7 macrophages were co-incubated with HKPerox-Red (4 μM) in Hank's Balanced Salt Solution (HBSS) for 30 min before imaging. As expected, the fluorescence signal in PMA treated cells was significantly stronger than that of untreated cells (Fig. 3a). This PMA induced H2O2 burst could be effectively inhibited by the addition of NADPH oxidase (NOX) inhibitor DPI, and quantifications based on imaging showed a clear 7-fold enhancement of fluorescence intensities (Fig. 3b). Endogenous H2O2 bursts can be robustly visualized in stimulated macrophages with HKPerox-Red in a selective manner.
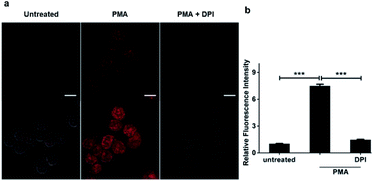 |
| Fig. 3 Molecular imaging of endogenous hydrogen peroxide in living cells. (a) Representative confocal images for RAW264.7 macrophages co-incubated with HKPerox-Red (4 μM) and CCl3CN (100 μM) in the absence or presence of PMA (200 ng mL−1) or PMA plus DPI (100 nM). (b) Relative mean fluorescence intensities of cells incubated with HKPerox-Red were quantified. Scale bars represent 10 μm. Data are mean ± SEM, n = 30–34 cells. Statistical significance was determined as ***p < 0.001 by Student's t test. PMA: phorbol 12-myristate 13-acetate; DPI: diphenyleneiodonium. | |
We next explored the applications of HKPerox-Red to in vivo imaging of zebrafish. To perform molecular imaging of H2O2 produced in living zebrafish, the desirable probe should be both lipophilic and water-soluble to penetrate through fish skin by simple co-incubation. HKPerox-Red, our resorufin-derived H2O2 probe, featuring small-molecular weight, good lipophilicity, excellent water-solubility, and most importantly, ultrasensitivity, is very promising for such study.
In our initial study, zebrafish embryos 6 hours post fertilization (hpf) were acquired to co-incubate with HKPerox-Red (including 100 μM CCl3CN in 1 mL E3 buffer) or HKPerox-2, our previous rhodol-base probe,10a at room temperature for 30 min to monitor zebrafish development process. Endogenous H2O2 produced during cell proliferation was clearly visualized by HKPerox-Red since the red fluorescence was detected inside zebrafish embryo (Fig. 4), while there was no fluorescence signal in the yolk part of the fertilized egg or outside of the embryo. In contrast, HKPerox-2 could not penetrate through the embryo membrane via co-incubation, and the resulting fluorescence signals were only found outside the embryo. Thus, HKPerox-Red could efficiently stain zebrafish embryo by simple co-incubation, and H2O2 production during cell proliferation in early stage of embryo development was visualized.
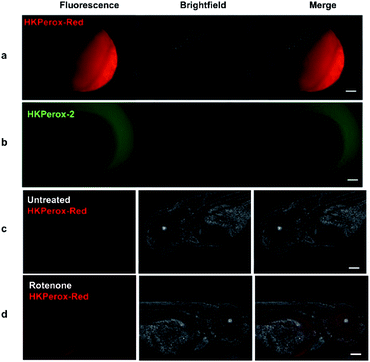 |
| Fig. 4 Molecular imaging of endogenous H2O2 in 6 hpf zebrafish embryos with (a) HKPerox-Red (20 μM) and (b) HKPerox-2 (20 μM). Molecular imaging of (c) untreated or (d) rotenone induced H2O2 in 72 hpf zebrafish embryos with HKPerox-Red (20 μM). Scale bars represent 100 μm. | |
Rotenone is a widely used pesticide that interferes with the electron transport chain during mitochondrial respiration. Previous study reveals that long term exposure to rotenone is associated with higher risk of Parkinson's disease in farm workers,15 while their models for rotenone challenging mainly focused on isolated mitochondria or cell lines,16 and the imaging reagents used to quantify ROS burst were traditional unselective probes. Thus, we applied HKPerox-Red to evaluate the effect of rotenone on zebrafish embryos. 72 hpf zebrafish embryos were incubated with HKPerox-Red (20 μM) for 20 min at room temperature, then challenged with or without rotenone (50 μM) in E3 buffer supplemented with 100 μM CCl3CN for 15 min. Basal H2O2 production and distribution could be visualized as a weak fluorescence signal (Fig. 4c). However, this signal was significantly stronger in rotenone treated zebrafish, which indicates acute H2O2 burst in living embryos. Distributions of H2O2 burst could be found in the brain and gut regions of zebrafish embryos, which may further contribute to oxidative damage of proteins and the initiation of neurodegenerative diseases.
New assay development based on HKPerox-Red
As H2O2 is a biologically important oxidizing reagent, its production and scavenging profiles are of great interest in health products, nutrition, and food industry.17 Moreover, H2O2 is one of the most common products of enzyme catalyzed oxidations, thus a series of metabolites including glucose, cholesterol, uric acid, and sarcosine could be converted to H2O2 quantitively with corresponding oxidases or enzyme mimetics.18
For example, in Amplex Red Glucose/Glucose Oxidase assay, glucose could be oxidized to glucuronolactone in the presence of glucose oxidase and oxygen, and one equivalent of H2O2 is produced and quantified by Amplex Red in this process. However, this resulting fluorescence could be bleached by extra amounts of H2O2 in presence of horseradish peroxidase (HRP), and Amplex Red is also vulnerable to photo oxidation.6f,19
Similar to Amplex Red in both excitation and emission wavelengths, HKPerox-Red can be a novel molecular tool compatible with current assays using Amplex Red, yet it is more stable, sensitive, and HRP independent. In HKPerox-Red glucose assay, various amounts of glucose (0–40 μM, final concentrations) were added into potassium phosphate buffer, and then 1 U mL−1 glucose oxidase and HKPerox-Red (10 μM; with 100 μM CCl3CN) were added subsequently into the solution. Resulting solutions were incubated for 30 min at 37 °C before fluorescence measurement. As shown in Fig. 5a, H2O2 produced by glucose oxidation could be sensitively detected with HKPerox-Red, and a linear relationship between glucose concentrations and fluorescence emissions at 602 nm was observed. The detection limit of glucose was calculated to be as low as 34 nM (3σ/k) according to the calibration curve.
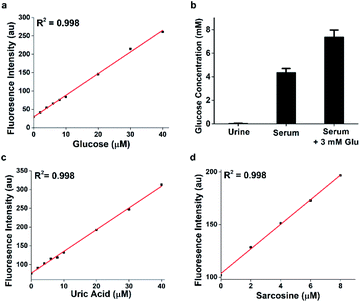 |
| Fig. 5 (a) Ultrasensitive detection of glucose with HKPerox-Red (10 μM) in aqueous buffer (pH 7.4, 0.5% DMF, 1 U mL−1 glucose oxidase, 100 μM CCl3CN). Excitation was provided at 565 nm; fluorescence emission was obtained at 602 nm. (b) Glucose quantifications of urine and serum samples using HKPerox-Red glucose assay. Data are mean ± SEM, n = 3. (c) Detection of uric acid with HKPerox-Red (10 μM, with 1 U mL−1 urate oxidase) in aqueous buffer. (d) Detection of sarcosine with HKPerox-Red (10 μM, with 1 U mL−1 sarcosine oxidase) in aqueous buffer. | |
To prove the practical applications of this novel assay, non-diabetic urine and serum samples were analyzed by HKPerox-Red glucose assay, and only 1 μL serum is needed due to the ultra-sensitivity. In this experiment, 1 μL deproteinized serum was diluted with 1 mL potassium phosphate buffer (pH 7.4, 0.5% DMF) containing 1 U mL−1 glucose oxidase, 10 μM HKPerox-Red and 100 μM CCl3CN. Quantifications were based on serum samples spiked with 3 mM glucose as internal standard, and the glucose concentration in serum sample was quantified as 4.38 ± 0.32 mM. Therefore, with tiny amount of sample, HKPerox-Red holds great potential to measure serum glucose in a fast, accurate, and high-throughput assay. The ultrasensitivity also makes it possible to conduct non-invasive glucose evaluation with samples like sweat, saliva or tears. More importantly, HKPerox-Red enables a general assay for biologically important metabolites detection with suitable oxidases. As a proof of concept, uric acid/urate oxidase assay and sarcosine/sarcosine oxidase assay were developed by using HKPerox-Red (Fig. 5c and d), which provide practical methods for disease diagnosis based on metabolite screening. A clinical study with HKPerox-Red is on-going to use urinary sarcosine as potential biomarker for prostate cancer diagnosis.
Ratiometric detection of H2O2 burst in As2O3 treated leukaemia cells by using flow cytometry
Next, we sought to explore ratiometric H2O2 sensing with our HKPerox-Ratio. Although some H2O2 ratiometric fluorescent probes have been developed for this purpose, very few of them are sensitive enough to be used in flow cytometry, and none of them is suitable for quantifying endogenous H2O2 in living cells.13 We firstly applied HKPerox-Ratio in flow cytometry to detect H2O2 fluxes in leukemia cells challenged with As2O3, a very effective approach to treat acute promyelocytic leukemia.20 As leukemia cells are suspension cells, they are not suitable for confocal imaging; however, flow cytometry enables quantitative analysis of a large population of suspension cells rapidly. Moreover, with a suitable ratiometric probe, multiple fluoresence emissions could be detected simultaneously by flow cytometry,21 and the green to blue emission ratio (Fgreen/Fblue) reported by HKPerox-Ratio is expected to be a more reliable ratiometric indicator of cellular H2O2.
For this purpose, U-937, NB4 and THP-1 leukemia cells were selected for the treatment with As2O3 for 24 h, and then untreated cells and As2O3 challenged cells were co-incubated with HKPerox-Ratio (10 μM) for 30 min before flow cytometry analysis. Over 10
000 cells were analysed, and Fgreen/Fblue in each individual cell was calculated by a FlowJo software. As shown in Fig. 6a–c, As2O3-treated leukemia cells could be clearly distinguished from those untreated cells by Fgreen/Fblue ratio (x-axis), which correlates with H2O2 levels. Further quantifications in Fig. 6d showed a significant enhancement in Fgreen/Fblue ratio in all three cell lines (1.9-fold for U-937, 1.7-fold for NB4, and 2.8-fold for THP-1), indicating a robust cellular H2O2 flux. This As2O3-challenged H2O2 burst may be responsible for differentiation or apoptosis of leukemia cells.22 To conclude, HKPerox-Ratio is a promising probe to detect H2O2 fluxes via flow cytometry in a ratiometric manner, which provides an ideal platform to screen novel apoptosis inducer for leukemia therapy.
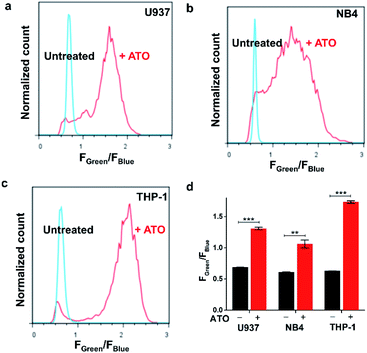 |
| Fig. 6 Detection of arsenic trioxide induced H2O2 in leukemia cells with flow cytometry. (a) U937, (b) NB4, and (c) THP-1 cells were treated with or without 20 μM As2O3 for 24 h, and then cells were incubated with HKPerox-Ratio (10 μM) and CCl3CN (100 μM) for 30 min before flow cytometry analysis. The y-axis is the normalized cell count; the x-axis is the Fgreen/Fblue ratio in each cell. (d) Fluorescence intensities ratios Fgreen/Fblue in untreated cells and As2O3 treated cells were compared. Data are mean ± SEM, n = 3 independent experiments. Statistical significance was determined as **p < 0.01, ***p < 0.001 by Student's t test. | |
Ratiometric fluorescence imaging of starvation induced H2O2 in living cells
As H2O2 is believed to be an important mediator during autophagy in response to nutrient shortage,23 we would like to identify and quantify endogenous H2O2 production during this process with ratiometric probe HKPerox-Ratio. RAW264.7 macrophages were co-incubated with HKPerox-Ratio (5 μM) in HBSS supplemented with 100 μM CCl3CN for 30 min at 37 °C with 5% CO2 before confocal imaging. The fluorescence signals in blue region (440–490 nm) and green region (540–650 nm) were collected simultaneously with an excitation at 405 nm (Fig. 7a and b). As expected, the blue emission was significantly stronger than the green emission in untreated cells, and the merged Fig. 7c showed the predominant blue emission. In Fig. 7d, a heatmap of green-to-blue emission ratio (Fgreen/Fblue) was generated by a Ratio Plus plugin in ImageJ software. Basal H2O2 concentrations and distributions were reported as a small value of Fgreen/Fblue (∼0.2), which is consistent with low H2O2 levels in resting cells. To mimic starvation induced stress, RAW264.7 cells were incubated HBSS (protein free) for 24 h, and then confocal images were taken under the same optical settings. Upon starvation challenge, blue emission in cells decreased significantly, while green emission increased due to H2O2 burst (Fig. 7e and f). The merged image in Fig. 7g showed a predominant green emission, and the ratiometric heatmap (Fig. 7h) confirmed a significant increase of Fgreen/Fblue (∼1.0) inside the cells.
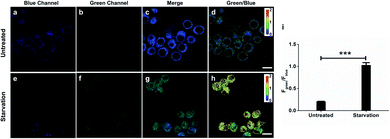 |
| Fig. 7 Confocal images of H2O2 production with HKPerox-Ratio (5 μM) in untreated or nutrient-depleted (HBSS treatment) RAW264.7 macrophages. (a) and (e) Representative fluorescence images in the blue channel (440–490 nm). (b) and (f) Representative fluorescence images in the green channel (540–650 nm). (c) and (g) Merged images of blue, green and brightfield channels. (d) and (h) Pseudo-color heatmap of green/blue emission ratio (Fgreen/Fblue). (i) Fgreen/Fblue of RAW 264.7 macrophages under homeostasis or starvation was quantified. Data are mean ± SEM, n = 19–29 cells. Statistical significance was determined as ***p < 0.001 by Student's t test. Scale bars represent 10 μm. | |
Quantification based on confocal images was performed to show a clear 5-fold overall increase of Fgreen/Fblue in starvation-challenged cells compared to resting cells (Fig. 7i), which demonstrated the excellent sensitivity of HKPerox-Ratio in cell imaging. The green signal and blue signal overlapped well with an overlap coefficient of 0.85 (Fig. S5, ESI†), which shows a good co-localization of HKPerox-Ratio and its cleavage product in cells. Collectively, those data demonstrate that HKPerox-Ratio can be robustly applied in ratiometric imaging of endogenous H2O2 fluxes with spatio-temporal precision.
Quantitative measurement of cellular H2O2 by flow cytometry with live cell calibration
Calibration curves of previous ratiometric probes were largely established in a cell-free solvent/buffer mixture, which may not be applicable for precise quantification of cellular H2O2.13 Therefore, we further applied HKPerox-Ratio in flow cytometry for quantitative measurement of H2O2 fluxes during starvation. As shown in Fig. 8a and b, a calibration curve with excellent linearity (R2 = 0.993) was obtained by adding known amounts of H2O2 (0, 10, 20, 40, and 80 μM H2O2) into the probe solution and then co-incubating with cells for 30 min before flow cytometry measurement. The green to blue emission ratio (Fgreen/Fblue) in each group of cells was analysed, and those cell populations could be distinguished in Fig. 8a. The detection limit of HKPerox-Ratio by flow cytometry measurement was estimated to be as low as 1.8 μM (3σ/k) in this cellular assay. A standard curve (y = 0.372 + 0.010x) with live cell calibration was thus established for RAW264.7 cells, enabling the direct measurement of intracellular H2O2 concentrations.
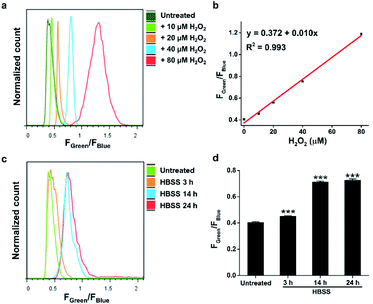 |
| Fig. 8 Measurements of H2O2 concentrations in nutrient-depleted (HBSS treatment) RAW264.7 macrophages by flow cytometry analysis. (a) Histogram of the Fgreen/Fblue ratio in RAW264.7 cells co-incubated with HKPerox-Ratio (10 μM) and CCl3CN (100 μM) pretreated with 0, 10, 20, 40, and 80 μM H2O2. (b) Calibration curve of Fgreen/Fblue in RAW264.7 cells as a function of H2O2 concentrations. (c) Histogram of the Fgreen/Fblue ratio in untreated or starvation challenged RAW264.7 cells incubated with HKPerox-Ratio (10 μM) and CCl3CN (100 μM). (d) Comparison of Fgreen/Fblue in untreated or starvation challenged cells. Data are mean ± SEM, n = 3 independent experiments. Statistical significance was determined as ***p < 0.001 by Student's t test. | |
As shown in Fig. 8c and d, starvation induced H2O2 was detected and quantified with HKPerox-Ratio. Our data showed that 3 h starvation already induced a discernible increase in Fgreen/Fblue ratio, corresponding to 7.9 μM H2O2 according to the calibration curve. This rapidly elevated cellular H2O2 levels during starvation suggest that treatment of cells in protein-free buffers (such as HBSS and phosphate-buffered saline) may stimulate unexpected ROS burst, thus longtime incubation in those buffers should be avoided.
Moreover, H2O2 fluxes in living RAW264.7 cells reached its maximum concentration (34 μM) after 14 h starvation, and longer period of starvation (e.g. 24 h) did not induce higher levels of H2O2. This endogenous H2O2 burst is sufficient to signal subsequent pathological processes, such as growth arrest or apoptosis.24
Due to variations of cellular environment, independent calibration curves should be obtained in different cell types before ratiometric H2O2 quantification. We further applied this protocol to HeLa cells, and a new calibration curve with excellent linearity (y = 0.606 + 0.013x, R2 = 0.988) was obtained with HKPerox-Ratio by using flow cytometry (Fig. S6, ESI†). Note that the calibration curve obtained by using a fluorospectrometer in chemical system (Fig. 2c, y = 0.670 + 0.053x) is very different from those obtained by using flow cytometry with live cell calibration. This result indicates that calibration curves established in a cell-free solvent/buffer mixture are not applicable to cellular H2O2 measurement; however, with a suitable ratiometric probe, flow cytometry provides an ideal high-throughput platform for quantitative analysis. One potential risk from live cell calibration may arise from different cell permeabilities of HKPerox-Ratio and its cleavage product. A control experiment is recommended to co-stain the cells with both compounds to confirm their similar permeability (Fig. S7, ESI†). To the best of our knowledge, HKPerox-Ratio is the first ratiometric probe that can be used to quantify cellular H2O2 level by flow cytometry, which makes it an invaluable tool for not only qualitative detection, but also quantification in redox biology.
Conclusions
During past decades, great efforts have been made to develop novel H2O2 sensors, and diverse roles of H2O2 in mediating various biological processes have been uncovered. However, unambiguous quantitative analysis of H2O2in vitro and in vivo still represents a substantial challenge even to date. In this work, we have developed a pair of highly selective and sensitive fluorescent probes for precise measurement of H2O2. Our unique strategy relies on tandem Payne/Dakin reaction, which provides a general and biocompatible strategy to distinguish H2O2 from other potential competing ROS. HKPerox-Red and HKPerox-Ratio have been successfully applied to evaluate the effects of PMA, As2O3, rotenone, and starvation in various models.
In particular, HKPerox-Red with outstanding permeability could be used in zebrafish imaging by simple co-incubation, and rotenone induced oxidative stress was also successfully visualized in vivo. Moreover, the outstanding performance of HKPerox-Red has been exploited to develop new assays for H2O2 scavenging capacity or H2O2-based quantification for metabolites including glucose, uric acid and sarcosine, which are regarded as diagnostic biomarkers for diabetes, gout, and prostate cancer, respectively. The intrinsic sensitivity of our novel strategy ensures its robust application for in vitro quantification of H2O2 and related metabolites.
Even more challenging in the redox biology field is to measure intracellular H2O2 accurately. In this study, we have developed HKPerox-Ratio, a small-molecule ratiometric probe, that allows quantitative detection of H2O2 by measuring the ratio of the blue and green emission. HKPerox-Ratio could be used in various cell lines with simple co-incubation, and the significant signal changes triggered by intracellular H2O2 were robustly captured by both flow cytometry and confocal imaging. Therefore, As2O3-induced H2O2 fluxes in multiple leukemia cells were readily detected. HKPerox-Ratio further enables the first quantitative detection of endogenously generated H2O2 in living cells by using flow cytometry and live cell calibration curve.
We anticipate that both probes provide important molecular tools to study H2O2 physiology and pathology, and more efforts are on-going to apply HKPerox series probes and tandem Payne/Dakin reaction in advanced bio-imaging, drug screening, and disease diagnostics.
Conflicts of interest
S. Y., J. J. H., and D. Y. have filed a patent application for the reported probes.
Acknowledgements
The study using zebrafish embryos up to 72 hpf was strictly conducted according to the approved protocols provided by Zebrafish Core Facility at HKU and Committee on the Use of Live Animals and Teaching and Research (CULATR) at HKU (Hong Kong, China). We thank the HKU Li Ka Shing Faculty of Medicine Faculty Core Facility for support in confocal imaging, flow cytometry analysis, and zebrafish imaging. This work was supported by The University of Hong Kong, Morningside Foundation, Hong Kong Research Grants Council Area of Excellence Scheme (AoE/P-705/16) and National Natural Science Foundation of China (21961142011).
Notes and references
-
(a) K. Apel and H. Hirt, Annu. Rev. Plant Biol., 2004, 55, 373–399 CrossRef CAS
;
(b) M. P. Murphy, Biochem. J., 2009, 417, 1–13 CrossRef CAS
.
-
(a) M. Reth, Nat. Immunol., 2002, 3, 1129–1134 CrossRef CAS
;
(b) M. Giorgio, M. Trinei, E. Migliaccio and P. G. Pelicci, Nat. Rev. Mol. Cell Biol., 2007, 8, 722–728 CrossRef CAS
;
(c) E. A. Veal, A. M. Day and B. A. Morgan, Mol. Cell, 2007, 26, 1–14 CrossRef CAS
.
- G. Filomeni, D. De Zio and F. Cecconi, Cell Death Differ., 2015, 22, 377–388 CrossRef CAS
.
-
(a) P. C. Panus, R. Radi, P. H. Chumley, R. H. Lillard and B. A. Freeman, Free Radical Biol. Med., 1993, 14, 217–223 CrossRef CAS
;
(b) X. Liu and J. L. Zweier, Free Radical Biol. Med., 2001, 31, 894–901 CrossRef CAS
;
(c) L. C. Seaver and J. A. Imlay, J. Bacteriol., 2001, 183, 7182–7189 CrossRef CAS
;
(d) E. Werner, Sci. Signaling, 2003, 2003, l3 CrossRef
;
(e) X. Li, Y. Liu, A. Zhu, Y. Luo, Z. Deng and Y. Tian, Anal. Chem., 2010, 82, 6512–6518 CrossRef CAS
;
(f) N. Zhang, W. Ma, P.-G. He and Y.-T. Long, J. Electroanal. Chem., 2015, 739, 197–201 CrossRef CAS
.
-
(a) M. C. Chang, A. Pralle, E. Y. Isacoff and C. J. Chang, J. Am. Chem. Soc., 2004, 126, 15392–15393 CrossRef CAS
;
(b) M. Abo, Y. Urano, K. Hanaoka, T. Terai, T. Komatsu and T. Nagano, J. Am. Chem. Soc., 2011, 133, 10629–10637 CrossRef CAS
;
(c) P. Gao, W. Pan, N. Li and B. Tang, Chem. Sci., 2019, 10, 6035–6071 RSC
;
(d) K. J. Bruemmer, S. W. M. Crossley and C. J. Chang, Angew. Chem., Int. Ed., 2020, 59, 13734–13762 CrossRef CAS
.
-
(a) B. C. Dickinson and C. J. Chang, Nat. Chem. Biol., 2011, 7, 504–511 CrossRef CAS
;
(b) X. H. Li, X. H. Gao, W. Shi and H. M. Ma, Chem. Rev., 2014, 114, 590–659 CrossRef CAS
;
(c) Z. Guo, S. Park, J. Yoon and I. Shin, Chem. Soc. Rev., 2014, 43, 16–29 RSC
;
(d) F. Rezende, R. P. Brandes and K. Schröder, Antioxid. Redox Signaling, 2017, 29, 585–602 CrossRef
;
(e) J. Huang, J. Li, Y. Lyu, Q. Miao and K. Pu, Nat. Mater., 2019, 18, 1133–1143 CrossRef CAS
;
(f) X. Bai, K. K.-H. Ng, J. J. Hu, S. Ye and D. Yang, Annu. Rev. Biochem., 2019, 88, 605–633 CrossRef CAS
.
-
(a) V. V. Belousov, A. F. Fradkov, K. A. Lukyanov, D. B. Staroverov, K. S. Shakhbazov, A. V. Terskikh and S. Lukyanov, Nat. Methods, 2006, 3, 281–286 CrossRef CAS
;
(b) D. S. Bilan, L. Pase, L. Joosen, A. Y. Gorokhovatsky, Y. G. Ermakova, T. W. J. Gadella, C. Grabher, C. Schultz, S. Lukyanov and V. V. Belousov, ACS Chem. Biol., 2013, 8, 535–542 CrossRef CAS
;
(c) B. Morgan, K. Van Laer, T. N. E. Owusu, D. Ezeriņa, D. Pastor-Flores, P. S. Amponsah, A. Tursch and T. P. Dick, Nat. Chem. Biol., 2016, 12, 437–443 CrossRef CAS
;
(d) T. F. Langford, B. K. Huang, J. B. Lim, S. J. Moon and H. D. Sikes, Nat. Commun., 2018, 9, 3145 CrossRef
;
(e) V. V. Pak, D. Ezeriņa, O. G. Lyublinskaya, B. Pedre, P. A. Tyurin-Kuzmin, N. M. Mishina, M. Thauvin, D. Young, K. Wahni, S. A. Martínez Gache, A. D. Demidovich, Y. G. Ermakova, Y. D. Maslova, A. G. Shokhina, E. Eroglu, D. S. Bilan, I. Bogeski, T. Michel, S. Vriz, J. Messens and V. V. Belousov, Cell Metab., 2020, 31, 642–653 CrossRef CAS
.
-
(a) E. W. Miller, A. E. Albers, A. Pralle, E. Y. Isacoff and C. J. Chang, J. Am. Chem. Soc., 2005, 127, 16652–16659 CrossRef CAS
;
(b) B. C. Dickinson and C. J. Chang, J. Am. Chem. Soc., 2008, 130, 9638–9639 CrossRef CAS
;
(c) A. R. Lippert, G. C. V. De Bittner and C. J. Chang, Acc. Chem. Res., 2011, 44, 793–804 CrossRef CAS
;
(d) L. Yuan, W. Lin, Y. Xie, B. Chen and S. Zhu, J. Am. Chem. Soc., 2012, 134, 1305–1315 CrossRef CAS
;
(e) X. Sun, S.-Y. Xu, S. E. Flower, J. S. Fossey, X. Qian and T. D. James, Chem. Commun., 2013, 49, 8311–8313 RSC
;
(f) J. Wang, W. Zhu, G. Niu, G. Jiang, Q. Chen, P. Gao, Y. Li, G. Zhang, X. Fan and B. Z. Tang, Chem. Commun., 2018, 54, 13957–13960 RSC
;
(g) Y. Zhang, C. Yan, C. Wang, Z. Guo, X. Liu and W.-H. Zhu, Angew. Chem., Int. Ed., 2020, 59, 9059–9066 CrossRef CAS
.
- X. Xie, X. e. Yang, T. Wu, Y. Li, M. Li, Q. Tan, X. Wang and B. Tang, Anal. Chem., 2016, 88, 8019–8025 CrossRef CAS
.
-
(a) S. Ye, J. J. Hu and D. Yang, Angew. Chem., Int. Ed., 2018, 57, 10173–10177 CrossRef CAS
;
(b) S. Ye, N. Hananya, O. Green, H. Chen, A. Q. Zhao, J. Shen, D. Shabat and D. Yang, Angew. Chem., Int. Ed., 2020, 59, 14326–14330 CrossRef CAS
.
- D. Pham, U. Basu, I. Pohorilets, C. S. Croix, S. C. Watkins and K. Koide, Angew. Chem., Int. Ed., 2020, 59, 17435–17441 CrossRef CAS
.
-
(a) D. Srikun, E. W. Miller, D. W. Domaille and C. J. Chang, J. Am. Chem. Soc., 2008, 130, 4596–4597 CrossRef CAS
;
(b) C. Chung, D. Srikun, C. S. Lim, C. J. Chang and B. R. Cho, Chem. Commun., 2011, 47, 9618–9620 RSC
;
(c) Y. Wen, K. Liu, H. Yang, Y. Li, H. Lan, Y. Liu, X. Zhang and T. Yi, Anal. Chem., 2014, 86, 9970–9976 CrossRef CAS
;
(d) B. Dong, X. Song, X. Kong, C. Wang, Y. Tang, Y. Liu and W. Lin, Adv. Mater., 2016, 28, 8755–8759 CrossRef CAS
;
(e) W. Zhang, T. Liu, F. Huo, P. Ning, X. Meng and C. Yin, Anal. Chem., 2017, 89, 8079–8083 CrossRef CAS
;
(f) H. Wang, Z. He, Y. Yang, J. Zhang, W. Zhang, W. Zhang, P. Li and B. Tang, Chem. Sci., 2019, 10, 10876–10880 RSC
;
(g) Z. Wang, X. Ai, Z. Zhang, Y. Wang, X. Wu, R. Haindl, E. K. L. Yeow, W. Drexler, M. Gao and B. Xing, Chem. Sci., 2020, 11, 803–811 RSC
.
- D. Andina, J.-C. Leroux and P. Luciani, Chem.–Eur. J., 2017, 23, 13549–13573 CrossRef CAS
.
-
(a) Z. Xu, J. Yoon and D. R. Spring, Chem. Commun., 2010, 46, 2563–2565 RSC
;
(b) Z.-Z. Li, C.-G. Niu, G.-M. Zeng, Y.-G. Liu, P.-F. Gao, G.-H. Huang and Y.-A. Mao, Sens. Actuators, B, 2006, 114, 308–315 CrossRef CAS
;
(c) V. S. Lin, W. Chen, M. Xian and C. J. Chang, Chem. Soc. Rev., 2015, 44, 4596–4618 RSC
;
(d) Y. Hu, X. Li, Y. Fang, W. Shi, X. Li, W. Chen, M. Xian and H. Ma, Chem. Sci., 2019, 10, 7690–7694 RSC
.
- M. T. Caroline, F. Kamel, G. W. Ross, A. H. Jane, M. G. Samuel, M. Korell, C. Marras, S. B. Grace, M. Kasten, R. C. Anabel, K. Comyns, B. R. Marie, C. Meng, B. Priestley, H. F. Hubert, F. Cambi, M. U. David, A. Blair, P. S. Dale and J. W. Langston, Environ. Health Perspect., 2011, 119, 866–872 CrossRef
.
-
(a) S. C. Sousa, E. N. Maciel, A. E. Vercesi and R. F. Castilho, FEBS Lett., 2003, 543, 179–183 CrossRef CAS
;
(b) C. M. Testa, T. B. Sherer and J. T. Greenamyre, Mol. Brain Res., 2005, 134, 109–118 CrossRef CAS
;
(c) N. Yadava and D. G. Nicholls, J. Neurosci., 2007, 27, 7310–7317 CrossRef CAS
.
-
(a) M. Özyürek, B. Bektaşoğlu, K. Güçlü, N. Güngör and R. Apak, J. Food Compos. Anal., 2010, 23, 689–698 CrossRef
;
(b) N. Higashi, H. Yokota, S. Hiraki and Y. Ozaki, Anal. Chem., 2005, 77, 2272–2277 CrossRef CAS
.
- M. E. Hafez, H. Ma, W. Ma and Y.-T. Long, Angew. Chem., Int. Ed., 2019, 58, 6327–6332 CrossRef CAS
.
-
(a) K. J. Reszka, B. A. Wagner, C. P. Burns and B. E. Britigan, Anal. Biochem., 2005, 342, 327–337 CrossRef CAS
;
(b) B. Zhao, F. A. Summers and R. P. Mason, Free Radical Biol. Med., 2012, 53, 1080–1087 CrossRef CAS
.
-
(a) G. Q. Chen, J. Zhu, X. G. Shi, J. H. Ni, H. J. Zhong, G. Y. Si, X. L. Jin, W. Tang, X. S. Li, S. M. Xong, Z. X. Shen, G. L. Sun, J. Ma, P. Zhang, T. D. Zhang, C. Gazin, T. Naoe, S. J. Chen, Z. Y. Wang and Z. Chen, Blood, 1996, 88, 1052–1061 CrossRef CAS
;
(b) G. Q. Chen, X. G. Shi, W. Tang, S. M. Xiong, J. Zhu, X. Cai, Z. G. Han, J. H. Ni, G. Y. Shi, P. M. Jia, M. M. Liu, K. L. He, C. Niu, J. Ma, P. Zhang, T. D. Zhang, P. Paul, T. Naoe, K. Kitamura, W. Miller, S. Waxman, Z. Y. Wang, H. de The, S. J. Chen and Z. Chen, Blood, 1997, 89, 3345–3353 CrossRef CAS
.
-
(a) A. Kaur, M. A. Haghighatbin, C. F. Hogan and E. J. New, Chem. Commun., 2015, 51, 10510–10513 RSC
;
(b) Y. Chen, C. Zhu, J. Cen, Y. Bai, W. He and Z. Guo, Chem. Sci., 2015, 6, 3187–3194 RSC
.
-
(a) K. Kinjo, M. Kizaki, A. Muto, Y. Fukuchi, A. Umezawa, K. Yamato, T. Nishihara, J. Hata, M. Ito, Y. Ueyama and Y. Ikeda, Leukemia, 2000, 14, 431–438 CrossRef CAS
;
(b) E.-K. Noh, H. Kim, M. J. Park, J. H. Baek, J.-H. Park, S. J. Cha, J.-H. Won and Y. J. Min, Leuk. Res., 2010, 34, 1501–1505 CrossRef CAS
.
-
(a) Y. Chen, E. McMillan-Ward, J. Kong, S. J. Israels and S. B. Gibson, Cell Death Differ., 2008, 15, 171–182 CrossRef CAS
;
(b) M. Dewaele, H. Maes and P. Agostinis, Autophagy, 2010, 6, 838–854 CrossRef CAS
;
(c) J. Lee, S. Giordano and J. H. Zhang, Biochem. J., 2012, 441, 523–540 CrossRef CAS
.
-
(a) F. Antunes and E. Cadenas, Free Radical Biol. Med., 2001, 30, 1008–1018 CrossRef CAS
;
(b) Q. M. Chen, J. Liu and J. B. Merrett, Biochem. J., 2000, 347, 543–551 CrossRef CAS
.
Footnote |
† Electronic supplementary information (ESI) available: Experiment details, including general methods, synthetic details, kinetic study, toxicity study and supplemental figures. See DOI: 10.1039/d0sc04888g |
|
This journal is © The Royal Society of Chemistry 2020 |
Click here to see how this site uses Cookies. View our privacy policy here.