DOI:
10.1039/D0SC03759A
(Edge Article)
Chem. Sci., 2020,
11, 8961-8965
Asymmetric synthesis of γ-chiral borylalkanes via sequential reduction/hydroboration using a single copper catalyst†
Received
9th July 2020
, Accepted 6th August 2020
First published on 6th August 2020
Abstract
The synthesis of γ-chiral borylalkanes through copper-catalyzed enantioselective SN2′-reduction of γ,γ-disubstituted allylic substrates and subsequent hydroboration was reported. A copper–DTBM-Segphos catalyst produced a range of γ-chiral alkylboronates from easily accessible allylic acetate or benzoate with high enantioselectivities up to 99% ee. Furthermore, selective organic transformations of the resulting γ-chiral alkylboronates generated the corresponding γ-chiral alcohol, arene and amine compounds.
Introduction
Efficient synthesis of enantiopure molecules with a stereogenic center remote from a functional group is of great interest in synthetic and medicinal chemistry, despite the difficulty of introducing such stereogenic centers.1 Especially, functionalized γ-chiral compounds represent important structural motifs in a diverse range of biologically active natural products and pharmaceutical drugs such as a marine natural product (curcuphenol) having inhibitory H,K-ATPase activity, an antimycobacterial agent (erogorgiaene) and a sleep agent (Ramelteon) (Fig. 1).2 In this context, γ-chiral organoboron compounds are valuable building blocks for the synthesis of functionalized chiral molecules due to efficient conversion of the carbon–boron bond to a range of carbon–carbon and carbon–heteroatom bonds.3 A typical approach towards γ-chiral organoborons is Matteson's homologation of enantioenriched β-chiral organoboranes with stoichiometric organolithium reagents (Scheme 1a).4 Despite the importance of these molecules, the direct preparation of γ-chiral organoboron compounds from easily accessible prochiral substrates remains unexplored in comparison with well-established methods for constructing α- and β-chiral organoboron compounds.5
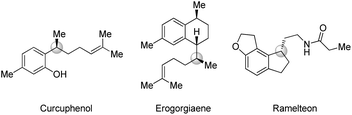 |
| Fig. 1 Representative functionalized γ-chiral compounds. | |
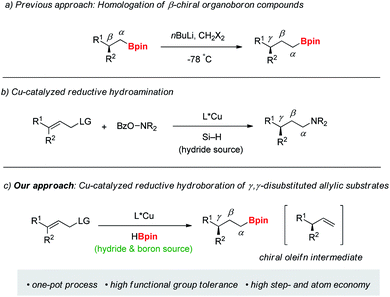 |
| Scheme 1 Approaches to γ-chiral organoboron compounds. | |
Transition-metal catalyzed allylation is one of the most efficient and reliable tools for the synthesis of functionalized chiral molecules owing to facile construction of new stereogenic centers with simultaneous introduction of a versatile olefin fragment.6 Among the various methods, copper-catalyzed allylations have been widely explored with a range of organometallic nucleophiles such as Grignard, organolithium, organoboron, and organozirconium reagents.7 More recently, organocopper nucleophiles, catalytically in situ generated from unsaturated substrates, have been utilized in copper-catalyzed C–C bond formation reactions.8 Despite these significant advances, use of a hydride nucleophile is still rare in the allylation. Only two examples of copper-catalyzed enantioselective allylic reduction with hydrosilane (Si–H) as the stoichiometric hydride source have been recently reported.9 One of them reported highly enantioselective SN2′-reduction/hydroamination in a one-pot sequence (Scheme 1b).9b
Recently, we reported copper-catalyzed enantioselective hydroborations of various olefins with pinacolborane (HBpin).10,11 While pinacolborane displayed higher efficiency for addition reactions to multiple bonds such as alkenes, alkynes, and carbonyl derivatives10–12 than hydrosilane in the presence of a copper catalyst, its reactivity toward substitution reactions is unknown to date. Moreover, in our previous study on the copper-catalyzed hydroboration, the reaction of allylic acetate with pinacolborane-derived copper-hydride catalyst gave only hydroboration product,10b indicating high tendency of pinacolborane for hydroboration of alkenes.
Our ongoing interest in copper-catalyzed synthesis of chiral organoboranes13 led us to explore preparation of γ-chiral organoboron compounds. Based on a possible dual role of pinacolborane to serve both as reducing and borating reagent, we envisioned that chiral copper-hydride species generated from HBpin could catalyze enantioselective SN2′-reduction of γ,γ-disubstituted allylic substrate, and hydroboration of the chiral intermediate olefins could afford γ-chiral organoboron compounds in a single operation (Scheme 1c). Herein, we report a general route for synthesis of γ-chiral organoboranes through reductive hydroboration strategy.
Results and discussion
In initial investigations, a series of chiral bisphosphine ligands (Fig. 2) were examined for reductive hydroboration of γ,γ-disubstituted allylic substrates (1a) derived from geraniol using pinacolborane (HBpin) (Table 1). Alkyl-tethered bisphosphine ligand L1 and ferrocene-based bisphosphine ligand L2 gave no desired product (entries 1 and 2). C2-symmetric tol-BINAP ligand L3 showed no reactivity, but L4 afforded the product in promising yield and with excellent enantioselectivity (entries 3 and 4). Although the Segphos (L5) did not provide the product, changing the ligand to DTBM-Segphos (L6) with its bulky aryl groups on the phosphine increased yield and enantioselectivity (entries 5 and 6).10b–d,11a Next, we screened a range of leaving groups (LG) of 1a for their effectiveness. Although use of allylic benzoate and carbonate afforded products in decreased yields, excellent enantioselectivities were conserved (entries 7 and 8). Allylic phosphate resulted in product in 60% yield and with 87% ee (entry 9), but allylic benzyl ether and bromide were inefficient (entries 10 and 11). Therefore, we chose acetate as the optimal leaving group, because it can be conveniently prepared from inexpensive acetic anhydride. Finally, prolongation of the reaction time to 24 h provided the product in 90% yield with retention of the high ee value (entry 12).
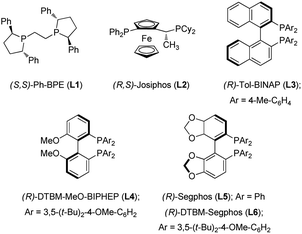 |
| Fig. 2 Structures of the chiral ligands. | |
Table 1 Optimization of reaction conditionsa
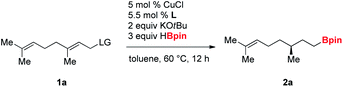
|
Entry |
Ligand |
LG |
Yieldb (%) |
eec |
Reactions were conducted on 0.5 mmol scale of 1a.
Isolated yield.
Determined by HPLC analysis on a chiral stationary phase.
The reaction was carried out for 24 h.
|
1 |
L1
|
OAc |
0 |
— |
2 |
L2
|
OAc |
0 |
— |
3 |
L3
|
OAc |
0 |
— |
4 |
L4
|
OAc |
63 |
98 |
5 |
L5
|
OAc |
0 |
— |
6 |
L6
|
OAc |
80 |
99 |
7 |
L6
|
OBz |
75 |
97 |
8 |
L6
|
OCO2Me |
59 |
99 |
9 |
L6
|
OP(O)(OEt)2 |
60 |
87 |
10 |
L6
|
OBn |
0 |
— |
11 |
L6
|
Br |
0 |
— |
12d |
L6
|
OAc |
90 |
99 |
With the optimized reaction conditions, the hydroboration of a range of γ,γ-disubstituted allylic substrates was investigated (Table 2). Allylic acetate derived from Nerol bearing a (Z)-olefin moiety was converted into 2b, the enantiomeric product opposite to 2a in high yield and enantioselectivity. Various functional groups were tolerated well, including chloro (2d), benzyl ether (2e), silyl ether (2f), and acetal group (2g) under the reaction conditions. While allylic acetate bearing a methyl and ethyl substituent on the γ-position underwent the reaction to afford highly enantioenriched alkylboronate (2h), the compounds (1i) bearing an ethyl and n-hexyl substituent resulted in drastically diminished yield and enantioselectivity. Bulky cyclohexyl (1j) and tert-butyl (1k) substituted allylic acetates were compatible and formed products in good yields and with excellent enantioselectivity. Similarly, silyl-substituted allylic acetate was converted into the γ-chiral silylalkylboronate (2l).
Table 2 Substrate scope in asymmetric reductive hydroborationa
Reactions were conducted on 0.5 mmol scale of 1. ee values of 2 were determined by HPLC analysis on a chiral stationary phase.
|
|
Aryl-substituted allyl benzoates (1m–1r) efficiently underwent the hydroboration.14 Substrates bearing phenyl, 4-fluoro-phenyl, 4-tolyl, 4-methoxy-phenyl, and 2-naphthyl group were suitable for the reaction. However, allylic benzoate (1r) with a phenyl and ethyl substituent at the γ-position provided the desired product in diminished yield and enantioselectivity, possibly due to increased steric bulkiness at the reaction site. In addition, we found that allylic benzoates with a substituent at the Cα or Cβ position were not efficient in yielding the desired products, probably due to enhanced steric hindrance around the olefin.15
To examine the mechanism of the reductive hydroboration, we performed the reaction of 1q with 1 equiv. of pinacolborane to observe the reaction intermediate (Scheme 2a). The reaction resulted in the formation of chiral olefin 1q′ in 64% yield without formation of further hydroboration product 2q, indicating that this cascade reaction proceeds via rate-determining SN2′-reduction step followed by hydroboration. Moreover, DFT calculations of transition state for hydrocupration step of the allylic substrate 1m revealed that the hydrocupration barrier for the major enantiomer is lower than that of the minor enantiomer by 4.6 kcal mol−1 (Scheme 2b).16 This energy difference of the transition states stems from steric repulsion between the phenyl substituent of 1m and the bulky P substituents of the ligand L6 (grey area l in the quadrant diagrams).
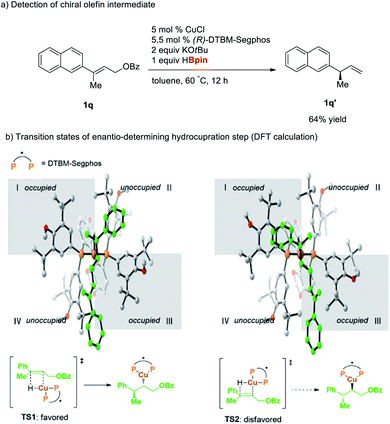 |
| Scheme 2 Mechanistic studies. | |
Based on the mechanistic studies, we propose a catalytic cycle for the reductive hydroboration (Fig. 3). Copper–H addition to the allylic substrate would generate a chiral alkylcopper species l, which rapidly undergoes β-LG elimination to afford the chiral olefin intermediate ll and L*Cu–LG.5a,17 Subsequent addition of copper-hydride species, regenerated from the reaction of L*Cu–LG with pinacolborane and alkoxide base to ll would produce terminal alkylcopper intermediate lll. Finally, transmetalation of lll with pinacolborane would result in the formation of the desired product, releasing the copper-hydride species.
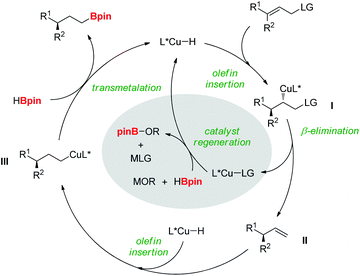 |
| Fig. 3 Proposed mechanism of copper-catalyzed reductive hydroboration. | |
Next, we examined applications of the resulting γ-chiral alkylboron compounds (Scheme 3). First, oxidation of 2a with sodium perborate yielded (−)-citronellol 3. Suzuki–Miyaura cross-coupling reaction of 2a with an aryl bromide afforded the arylated product 4.18 Furthermore, 2m was transformed into the Boc-protected amine 5 through an amination and Boc protection.19
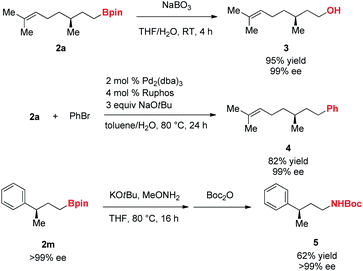 |
| Scheme 3 Application of γ-chiral alkylboron compounds. | |
Conclusion
In summary, we have described an efficient catalytic method for the synthesis of γ-chiral alkylboronates via SN2′-reduction and hydroboration. The DTBM-Segphos–copper complex successfully catalyzed the enantioselective allylic reduction of γ,γ-disubstituted allylic acetate (or benzoate) and subsequent hydroboration to produce γ-chiral alkylboronates in a one-pot cascade manner. This process provides a modular and general approach towards synthesis of γ-chiral organoboron compounds. Efforts to utilize a copper-hydride catalyst derived from pinacolborane in asymmetric synthesis are in progress.
Conflicts of interest
There are no conflicts to declare.
Acknowledgements
This study was supported by National Research Foundation of Korea (NRF) grants (2019R1A2C2005706 and 2019R1A4A2001440), funded by the Korean government (MEST). The authors thank Dr J. H. Moon for helpful discussions.
Notes and references
-
(a) E. W. Werner, T.-S. Mei, A. J. Burckle and M. S. Sigman, Science, 2012, 338, 1455 CrossRef CAS PubMed;
(b) T.-S. Mei, H. H. Patel and M. S. Sigman, Nature, 2014, 508, 340 CrossRef CAS PubMed;
(c) W.-B. Liu, N. Okamoto, E. J. Alexy, A. Y. Hong, K. Tran and B. M. Stoltz, J. Am. Chem. Soc., 2016, 138, 5234 CrossRef CAS PubMed;
(d) Z.-X. Wang, X.-Y. Bai, H.-C. Yao and B.-J. Li, J. Am. Chem. Soc., 2016, 138, 14872 CrossRef CAS PubMed;
(e) J. Liu, Q. Yuan, F. D. Toste and M. S. Sigman, Nat. Chem., 2019, 11, 710 CrossRef CAS PubMed.
-
(a) A. E. Wright, S. A. Pomponi, O. J. McConnell, S. Kohmoto and P. J. McCarthy, J. Nat. Prod., 1987, 50, 976 CrossRef CAS;
(b) A. D. Rodriguez and C. Ramirez, J. Nat. Prod., 2001, 64, 100 CrossRef CAS PubMed;
(c) S. Hegde and M. Schmidt, Annu. Rep. Med. Chem., 2006, 41, 439 CAS.
- For reviews, see:
(a) H. K. Scott and V. K. Aggarwal, Chem.–Eur. J., 2011, 17, 13124 CrossRef CAS PubMed;
(b) C. Sandford and V. K. Aggarwal, Chem. Commun., 2017, 53, 5481 RSC.
-
(a) D. S. Matteson, Chem. Rev., 1989, 89, 1535 CrossRef CAS;
(b)
D. S. Matteson, Stereodirected Synthesis with Organoboranes, Springer, New York, 1995 CrossRef;
(c) D. S. Matteson, J. Org. Chem., 2013, 78, 10009 CrossRef CAS PubMed.
-
(a) H. Ito, S. Ito, Y. Sasaki, K. Matsuura and M. Sawamura, J. Am. Chem. Soc., 2007, 129, 14856 CrossRef CAS PubMed;
(b) H. Ito, Y. Kosaka, K. Nonoyama, Y. Sasaki and M. Sawamura, Angew. Chem., Int. Ed., 2008, 47, 7424 CrossRef CAS PubMed;
(c) Y. Lee and A. H. Hoveyda, J. Am. Chem. Soc., 2009, 131, 3160 CrossRef CAS PubMed;
(d) N. Matsuda, K. Hirano, T. Satoh and M. Miura, J. Am. Chem. Soc., 2013, 135, 4934 CrossRef CAS PubMed;
(e) F. Meng, K. P. McGrath and A. H. Hoveyda, Nature, 2014, 513, 367 CrossRef CAS PubMed;
(f) K. Kubota, E. Yamamoto and H. Ito, J. Am. Chem. Soc., 2015, 137, 420 CrossRef CAS PubMed;
(g) T. Jia, P. Cao, B. Wang, Y. Lou, X. Yin, M. Wang and J. Liao, J. Am. Chem. Soc., 2015, 137, 13760 CrossRef CAS PubMed;
(h) D. Nishikawa, K. Hirano and M. Miura, J. Am. Chem. Soc., 2015, 137, 15620 CrossRef CAS PubMed;
(i) F. Meng, X. Li, S. Torker, Y. Shi, X. Shen and A. H. Hoveyda, Nature, 2016, 537, 387 CrossRef CAS PubMed;
(j) K. Yeung, R. E. Ruscoe, J. Rae, A. P. Pulis and D. J. Procter, Angew. Chem., Int. Ed., 2016, 55, 11912 CrossRef CAS PubMed;
(k) H. Jang, F. Romiti, S. Torker and A. H. Hoveyda, Nat. Chem., 2017, 9, 1269 CrossRef CAS PubMed;
(l) K. M. Logan and M. K. Brown, Angew. Chem., Int. Ed., 2017, 56, 851 CrossRef CAS PubMed;
(m) Y. Huang, K. B. Smith and M. K. Brown, Angew. Chem., Int. Ed., 2017, 56, 13314 CrossRef CAS PubMed;
(n) J. Lee, S. Radomkit, S. Torker, J. del Pozo and A. H. Hoveyda, Nat. Chem., 2018, 10, 99 CrossRef CAS PubMed;
(o) T. Itoh, Y. Kanzaki, Y. Shimizu and M. Kanai, Angew. Chem., Int. Ed., 2018, 57, 8265 CrossRef CAS PubMed.
- For reviews, see:
(a) H. Miyabe and Y. Takemoto, Synlett, 2005, 1641 CAS;
(b) J. T. Mohr and B. M. Stoltz, Chem.–Asian J., 2007, 2, 1476 CrossRef CAS PubMed;
(c) J. F. Hartwig and L. M. Stanley, Acc. Chem. Res., 2010, 43, 1461 CrossRef CAS PubMed;
(d) J. F. Hartwig and M. J. Pouy, Top. Organomet. Chem., 2011, 34, 169 CrossRef CAS;
(e) W.-B. Liu, J.-B. Xia and S.-L. You, Top. Organomet. Chem., 2012, 38, 155 CrossRef;
(f) Q. Cheng, H.-F. Tu, C. Zheng, J.-P. Qu, G. Helmchen and S.-L. You, Chem. Rev., 2019, 119, 1855 CrossRef CAS PubMed.
- For reviews, see:
(a) S. R. Harutyunyan, T. den Hartog, K. Geurts, A. J. Minnaard and B. L. Feringa, Chem. Rev., 2008, 108, 2824 CrossRef CAS PubMed;
(b) A. Alexakis, J. E. Bäckvall, N. Krause, O. Pàmies and M. Diéguez, Chem. Rev., 2008, 108, 2796 CrossRef CAS PubMed;
(c) C. A. Falciola and A. Alexakis, Eur. J. Org. Chem., 2008, 3765 CrossRef CAS;
(d) J.-B. Langlois and A. Alexakis, Top. Organomet. Chem., 2012, 38, 235 CrossRef;
(e) V. Hornillos, J.-B. Gualtierotti and B. L. Feringa, Top. Organomet. Chem., 2016, 58, 1 CrossRef CAS;
(f) R. Shintani, Synthesis, 2016, 48, 1087 CrossRef CAS;
(g) H. You, E. Rideau, M. Sidera and S. P. Fletcher, Nature, 2015, 517, 351 CrossRef CAS PubMed.
- For a review on copper-catalyzed hydrofunctionalization of alkenes:
(a) H. Wang and S. L. Buchwald, Org. React., 2019, 100, 121 Search PubMedFor reviews on sequential hydrofunctionalization:
(b) X. Zeng, Chem. Rev., 2013, 113, 6864 CrossRef CAS PubMed;
(c) Z. Cheng, J. Guo and Z. Lu, Chem. Commun., 2020, 56, 2229 RSCFor a review on earth-abundant transition metal-catalyzed hydrofunctionalization of alkenes, see:
(d) J. Chen, J. Guo and Z. Lu, Chin. J. Chem., 2018, 36, 1075 CrossRef CAS;
(e) J. Chen and Z. Lu, Org. Chem. Front., 2018, 5, 260 RSC.
-
(a) T. N. T. Nguyen, N. O. Thiel and J. F. Teichert, Chem. Commun., 2017, 53, 11686 RSC;
(b) S. Zhu, N. Niljianskul and S. L. Buchwald, Nat. Chem., 2016, 8, 144 CrossRef CAS PubMed.
- Our reports on copper-catalyzed enantioselective hydroboration:
(a) D. Noh, H. Chea, J. Ju and J. Yun, Angew. Chem., Int. Ed., 2009, 48, 6062 CrossRef CAS PubMed;
(b) D. Noh, S. K. Yoon, J. Won, J. Y. Lee and J. Yun, Chem.–Asian J., 2011, 6, 1967 CrossRef CAS PubMed;
(c) X. Feng, H. Jeon and J. Yun, Angew. Chem., Int. Ed., 2013, 52, 3989 CrossRef CAS PubMed;
(d) W. J. Jang, S. M. Song, J. H. Moon, J. Y. Lee and J. Yun, J. Am. Chem. Soc., 2017, 139, 13660 CrossRef CAS PubMed;
(e) W. J. Jang, S. M. Song, Y. Park and J. Yun, J. Org. Chem., 2019, 84, 4429 CrossRef CAS PubMed.
- Other group's reports on copper-catalyzed enantioselective hydroboration:
(a) Y. Xi and J. F. Hartwig, J. Am. Chem. Soc., 2016, 138, 6703 CrossRef CAS PubMed;
(b) Y. Huang, J. del Pozo, S. Torker and A. H. Hoveyda, J. Am. Chem. Soc., 2018, 140, 2643 CrossRef CAS PubMed;
(c) D.-W. Gao, Y. Xia, M. Liu, Z. Liu, M. K. Karunananda, J. S. Chen and K. M. Engle, ACS Catal., 2018, 8, 3650 CrossRef CAS PubMed;
(d) H. L. Sang, S. Yu and S. Ge, Org. Chem. Front., 2018, 5, 1284 RSC.
-
(a) B. H. Lipshutz, Ž. V. Bošković and D. H. Aue, Angew. Chem., Int. Ed., 2008, 47, 10183 CrossRef CAS PubMed;
(b) K. Semba, T. Fujihara, J. Terao and Y. Tsuji, Chem.–Eur. J., 2012, 18, 4179 CrossRef CAS PubMed;
(c) S. Lee, D. Li and J. Yun, Chem.–Asian J., 2014, 9, 2440 CrossRef CAS PubMed;
(d) A. Nagy, L. Collard, K. Indukuri, T. Leyssens and O. Riant, Chem.–Eur. J., 2019, 25, 8705 CAS;
(e) X. Liu, W. Ming, Y. Zhang, A. Friedrich and T. B. Marder, Angew. Chem., Int. Ed., 2019, 58, 18923 CrossRef CAS PubMed.
-
(a) J.-E. Lee and J. Yun, Angew. Chem., Int. Ed., 2008, 47, 145 CrossRef CAS PubMed;
(b) X. Feng and J. Yun, Chem.–Eur. J., 2010, 16, 13609 CrossRef CAS PubMed;
(c) H. Lee, B. Y. Lee and J. Yun, Org. Lett., 2015, 17, 764 CrossRef CAS PubMed;
(d) J. T. Han, W. J. Jang, N. Kim and J. Yun, J. Am. Chem. Soc., 2016, 138, 15146 CrossRef CAS PubMed;
(e) W. J. Jang and J. Yun, Angew. Chem., Int. Ed., 2018, 57, 12116 CrossRef CAS PubMed;
(f) W. J. Jang and J. Yun, Angew. Chem., Int. Ed., 2019, 58, 18131 CrossRef CAS;
(g) H. Lee, S. Lee and J. Yun, ACS Catal., 2020, 10, 2069 CrossRef CAS.
- The reaction of allylic acetate (1m-OAc) having a phenyl substituent under the standard reaction conditions produced the corresponding hydrolyzed alcohol product instead of 2m. The benzoate provided better stability toward base catalyzed hydrolysis and used for the reductive hydroboration. See Scheme S1 in the ESI.†.
- See Scheme S2 in the ESI† for details.
- For details, see Fig. S1 and S2 in the ESI.†.
-
(a) H. Ito, T. Okura, K. Matsuura and M. Sawamura, Angew. Chem., Int. Ed., 2010, 122, 570 CrossRef;
(b) H. Ohmiya, U. Yokobori, Y. Makida and M. Sawamura, J. Am. Chem. Soc., 2010, 132, 2895 CrossRef CAS PubMed;
(c) K. Nagao, U. Yokobori, Y. Makida, H. Ohmiya and M. Sawamura, J. Am. Chem. Soc., 2012, 134, 8982 CrossRef CAS PubMed.
- C.-T. Yang, Z.-Q. Zhang, H. Tajuddin, C.-C. Wu, J. Liang, J.-H. Liu, Y. Fu, M. Czyzewska, P. G. Steel, T. B. Marder and L. Liu, Angew. Chem., Int. Ed., 2012, 51, 528 CrossRef CAS PubMed.
- E. K. Edelstein, A. C. Grote, M. D. Palkowitz and J. P. Morken, Synlett, 2018, 29, 1749 CrossRef CAS PubMed.
Footnote |
† Electronic supplementary information (ESI) available: Optimization tables, experimental procedures, and characterization data. See DOI: 10.1039/d0sc03759a |
|
This journal is © The Royal Society of Chemistry 2020 |