DOI:
10.1039/D0SC03606D
(Edge Article)
Chem. Sci., 2020,
11, 7807-7812
Synthesis of unsymmetrical sulfamides and polysulfamides via SuFEx click chemistry†
Received
1st July 2020
, Accepted 10th July 2020
First published on 13th July 2020
Abstract
As hydrogen-bond donors and acceptors, N,N′-disubstituted sulfamides have been used in a range of applications from medicinal chemistry to anion-binding catalysis. However, compared to ureas or thioureas, the utilization of this unique moiety remains marginal, in part because of a lack of general synthetic methods to access unsymmetrical sulfamides. Specifically, polysulfamides are a virtually unknown type of polymer despite their potential utility in non-covalent dynamic networks, an intense area of research in materials science. We report herein a practical and efficient process to prepare unsymmetrical sulfamides via Sulfur(VI)-Fluoride Exchange (SuFEx) click chemistry. This process was then applied to synthesize polysulfamides. Thermal analysis showed that this family of polymers possess high thermal stability and tunable glass transition temperatures. Finally, hydrolysis studies indicated that aromatic polysulfamides could be recycled back to their constituting monomers at the end of their life cycle.
Introduction
Condensation polymerization is at the core of many industrial processes producing high-commodity polymers, such as polyesters, polyamides, polycarbonates, and polyurethanes. The syntheses of these polymers largely rely on robust and high-yielding condensation reactions at activated carbonyl groups and have been optimized over decades of chemical research. The recent development of the Sulfur(VI)-Fluoride Exchange (SuFEx) click chemistry1–3 has enabled the synthesis of unique classes of polymers wherein the conventional carbonyl group is replaced by the more esoteric –SO2– linker. For example, polysulfates4–7 and polysulfonates8,9 have been accessed and their properties compared to the analogous polycarbonates and polyesters. Among the family of hexavalent sulfur moieties, sulfamides are especially intriguing. As an SO2 analog of ureas, sulfamides can engage in hydrogen bonding as hydrogen-bond donors and acceptors,10,11 a powerful feature for materials science and organic chemistry alike. For example, aliphatic N,N′-disubstituted sulfamides have been used as gelators to trigger the assembly of 3D networks and vesicles.12–14 In a medicinal chemistry setting, sulfamides can be used as bioisosteres for amides, ureas, and carbamates, and have become more common in the medicinal chemist's arsenal.15 For example, the broad-spectrum antibiotic doripenem contains a monosubstituted sulfamide pharmacophore.16 Hydrogen-bonding organocatalysis could become a natural avenue of research for these compounds, but only a handful of useful transformations have been reported with sulfamides thus far.17–21 A lack of attention towards this family of molecules stems from the challenging preparation of unsymmetrical sulfamides. Similarly, while polyureas are used as a commodity polymer (e.g., spandex), only a few syntheses of polysulfamides have been reported, rendering their properties underexplored.22 Early work in the 1960s demonstrated that condensation between aliphatic bis(amine) 1a and sulfamide (2a) at high temperatures produced insoluble macromolecules that were not fully characterized (Fig. 1a).23–25 More recently, Rudkevich and coworkers synthesized oligomer 3b (Mn = 1.7 kg mol−1) using 1,4-phenylenediamine (1b) in combination with SO2, I2, and pyridine or triethylamine (Fig. 1a).22 The authors postulated the generation of a variety of hexavalent species including SO2I2.26
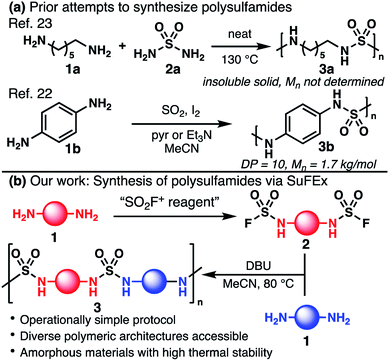 |
| Fig. 1 (a) Early efforts toward polysulfamides led to oligomers or insoluble solids. (b) Synthesis of fully characterizable polysulfamides via SuFEx click chemistry. | |
Considering the Carothers equation,27 we hypothesized that in order to obtain polysulfamides with higher degrees of polymerization (DP), we should identify conditions that (1) afford control over the stoichiometry of AA/BB type monomers, and (2) avoid potential deleterious oxidative side-reactions that diminish the overall yield. We report herein the development of conditions based on SuFEx click chemistry for the high-yielding preparation of isolable sulfamoyl fluorides, unsymmetrical sulfamides, and polysulfamides (Fig. 1b).
Results and discussion
While several methods producing sulfamides have been reported, they generally suffer from narrow substrate scope and variable yields.28–37 Moreover, the iterative addition of two different amines to an electrophilic sulfur(VI) reagent is often complicated by either the high instability of the intermediate or, on the contrary, insufficient reactivity of this species. SuFEx is attractive due to its mildness, efficiency, atom-economy, and stability of the fluoride-containing intermediate. For example, Sharpless disclosed an efficient synthesis of an unsymmetrical tetraalkylated sulfamide using SO2F2 and two secondary amines. Importantly, the sulfamoyl fluoride intermediate could be isolated and purified.38 However, these conditions are known to be unsuitable for primary amines.1,39,40 Indeed, treating benzylamine (4a) with SO2F2 (commercial or ex situ generated)41 led to the formation of undesired symmetrical sulfamide 7a as the major product, likely through the generation of azasulfene 6a (Fig. 2a, S1 and S2†).42 We instead turned our attention to SO2F2 alternatives. Sulfamides have been synthesized using thionyl tetrafluoride (SOF4), but this gas is not commercially available and its preparation is hazardous.43 Dong and Sharpless demonstrated that imidazolium derivative 8, a shelf-stable crystalline solid analog of SO2F2, allows for the preparation of monosubstituted sulfamoyl fluoride in high yield (Fig. 2b).44 We were pleased to find that this reagent afforded a variety of bis(sulfamoyl fluoride) monomers (2b–e) when used in combination with simple primary bis(amine)s in DCM (Fig. 2c). Notably, no precautions were taken to remove water and oxygen from the reaction, and both alkyl and aryl monomers can be synthesized in good yields. These monomers are shelf-stable for several days, but should be stored at 0 °C for extended periods of time to prevent slow decomposition. While decomposition is rapid in basic media, these monomers, apart from 2b, exhibit high tolerance to acidic conditions and can be purified through column chromatography. Yet, most impurities can be removed through aqueous workup, which is a testament to the practicality of the sulfamoylation protocol. Finally, it was found that [4-(acetylamino)phenyl]imidodisulfuryl difluoride (AISF, 9),45 another crystalline solid analog of SO2F2, can also be used to functionalize primary alkyl amines (Fig. 2b). Yields were found to be lower and these conditions are not applicable to aromatic amines, but SO2F2 is not required for the preparation of AISF—by contrast to that of 8—which can be advantageous for laboratories not equipped with this reagent.
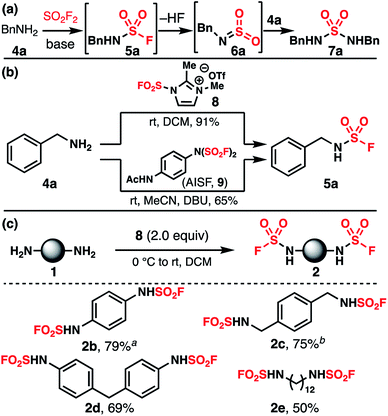 |
| Fig. 2 (a) Undesired formation of symmetrical sulfamide 7a from 4a using SO2F2. (b) Sulfamoylation of primary amines with reagents 8 or 9. (c) Synthesis of bis(sulfamoyl fluoride) monomers 2 from primary bis(amine)s 1. Deviations from standard conditions: a1.8 equiv. of 8; bMeCN as solvent. | |
With sulfamoyl fluoride monomers in hand, we focused on the optimization of the second step of the SuFEx coupling. Treatment of 5a with benzylamine (4a) in MeCN afforded dibenzylsulfamide (7a) in 70% yield after 2 h at 80 °C (Table 1, entry 1). Addition of a catalytic amount of DBU increased the yield to 78%, while with a stoichiometric amount of DBU, the yield improved to 97% (entries 2 and 3). Using a less basic aromatic amine as the coupling partner resulted in lower yield in the absence of additional base (Tables S1 and S2†). With our goal of developing a robust and universal protocol independent of the innate basicity of the amine partner, the amount of added base was kept to at least one equivalent, which also allows for trapping the resulting fluoride as a base-H+–F− salt. Triethylamine and potassium carbonate were found to afford similar yields to DBU (entries 4 and 5). Pyridine (entry 6) did not provide a noticeable improvement as compared to entry 1 but was found to perform best for aryl sulfamoyl fluorides (Fig. 3). A variety of solvents were screened as well (Table S1†). Interestingly, a nonpolar aromatic solvent such as PhMe delivered 7a in a similar yield as with MeCN, while DMF showed a decrease in yield (entries 7 and 8). Finally, quantitative yield could be achieved by running the reaction in MeCN at 50 °C for 4 h (entry 9).46 Again, all reactions were performed without removal of water or oxygen, which renders the overall SuFEx process operationally very simple. The optimized conditions were then used to synthesize a variety of sulfamides (Fig. 3). Monosubstituted alkyl sulfamoyl fluoride 5a was coupled with benzylamine (4a) and aniline (4b) in high yields (products 7a and 7c) using DBU. Monosubstituted aryl sulfamoyl fluoride 5b afforded similar yields with the same coupling partners (products 7b and 7c) using pyridine instead. Secondary amines such as N-benzylmethylamine (4c) can also be used in the second step to deliver trisubstituted sulfamides 7d and 7e. The lack of reactivity of dialkylated sulfamoyl fluoride such as S4 (Fig. S3†), as well as 19F NMR analysis of a solution of 5a and base (Fig. S4†), strongly suggest the generation of azasulfene intermediates in this process.42
Table 1 Optimization of the sulfamide synthesis

|
Entrya |
Solvent |
Temp. (°C) |
Base (equiv.) |
Time (h) |
Yieldb (%) |
Reactions were run on 0.1 mmol scale.
NMR yields using an internal standard.
|
1 |
MeCN |
80 |
— |
2 |
70 |
2 |
MeCN |
80 |
DBU (0.1) |
2 |
78 |
3 |
MeCN |
80 |
DBU (1.0) |
2 |
97 |
4 |
MeCN |
80 |
Et3N (1.0) |
2 |
95 |
5 |
MeCN |
80 |
K2CO3 (1.0) |
2 |
94 |
6 |
MeCN |
80 |
Pyr. (1.0) |
2 |
71 |
7 |
PhMe |
80 |
DBU (1.0) |
2 |
89 |
8 |
DMF |
80 |
DBU (1.0) |
2 |
77 |
9 |
MeCN |
50 |
DBU (1.0) |
4 |
99 |
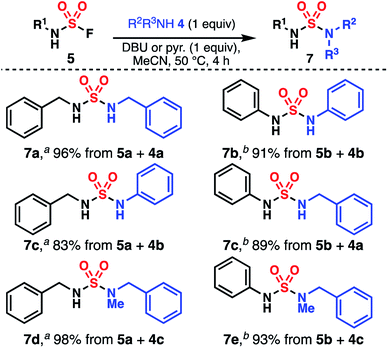 |
| Fig. 3 Synthesis of a variety of sulfamides via SuFEx with isolated yields (0.5–0.6 mmol scale). aDBU; bpyridine. | |
These reaction conditions were then applied to the synthesis of polysulfamides using bis(sulfamoyl fluoride) monomers 2 and commercially available bis(amine)s 1 (Fig. 4). Although premature precipitation of the growing polymers in MeCN was observed initially, this was overcome by increasing the temperature of the reaction to 80 °C and adding an excess of DBU (5 equiv.). A broad array of polymers was obtained with diverse architectures and Mn's between 3 and 9 kg mol−1. Notably, polymer 3b showed a DP of 39 (Mn = 6.7 kg mol−1) using this protocol, which compares favorably to the prior synthesis of 3b (DP = 10, Mn = 1.7 kg mol−1, Fig. 1a).22 Attempts to directly polymerize bis(amine) monomer 1b with SO2F2 failed. Additionally, aryl and alkyl sulfamides can be incorporated in the polymer backbone with similar ease. All synthesized polymers were soluble in DMSO and most in DMAc or DMF, which allowed for full characterization using NMR and size-exclusion chromatography (SEC) (ESI†). Thermal properties of this family of polymers were explored via thermogravimetric analysis (TGA) and differential scanning calorimetry (DSC). All polymers exhibited high thermal stability with decomposition temperatures ranging from 198 °C for 3b to 265 °C for 3n. Although polymers 3b–3n all contain hydrogen-bond donors and acceptors in their repeating units, an impressive range of glass-transition temperatures were measured. Tg's as low as 46 °C and 49 °C were determined for 3j and 3l with aliphatic segments in the backbone, while Tg's above 170 °C were observed for 3f, 3k, and 3n, all three polymers characterized by stiffer aromatic and cyclic repeating motifs. Copolymers 3o and 3p were synthesized using bis(sulfamoyl fluoride) 2c and bis(amine)s 1b and 1g in different ratios. The Tg's of 3o and 3p, 62 °C and 88 °C respectively, are in between that of homopolymers 3j (46 °C) and 3f (170 °C). Interestingly, the Tg of the copolymers differs from the value predicted using the Fox equation (Table S6†).47 These discrepancies are likely the result of intermolecular interactions such as hydrogen bonding. Overall, Tg increases with an increasing amount of monomer 1b, which highlights the facile tunability of Tg as a function of the molecular structure of the monomers. Strikingly, DSC analysis revealed an absence of crystallization and melting temperatures for all these materials. This thermal response contrasts with the melting temperatures measured in low-molecular-weight sulfamide films13 and the high-crystallinity observed with these small molecules.10–14 Powder X-ray diffraction performed on polysulfamides 3b–3n provided a more precise depiction of their structural order (ESI†). Polysulfamides exhibited varying degrees of crystallinity, from amorphous for 3i, 3k, 3m, and 3n to semicrystalline for 3c, 3e, 3f, 3j, and 3l. This behavior is reminiscent of work by Aida and coworkers,48 which demonstrated that low Mn polythioureas may be amorphous due to a zig-zag arrangement of the hydrogen-bond network. As a result, these polythioureas can be processed into materials mechanically robust and self-healable. Polyureas, on the other hand, are generally characterized by a linear array of hydrogen-bond networks,49,50 rendering them semicrystalline and brittle. IR spectroscopy was used to probe the hydrogen-bond interactions in 3b–3n in the solid state. Strong
NH peaks at approximately 3290 cm−1 were found in both 3c and N,N′-dibenzylsulfamide (7a) (Fig. S6†) and are consistent with N–H bonds engaged in hydrogen-bonding based on prior studies.12–14 By contrast, the IR spectrum of 3b displayed much broader
NH peaks, suggesting that the structure of the backbone strongly affects the hydrogen bonding ability of the repeating sulfamide groups. In-depth characterization of the hydrogen-bonding architecture in various polysulfamides will therefore be necessary to shed light on their specific thermal and mechanical properties.
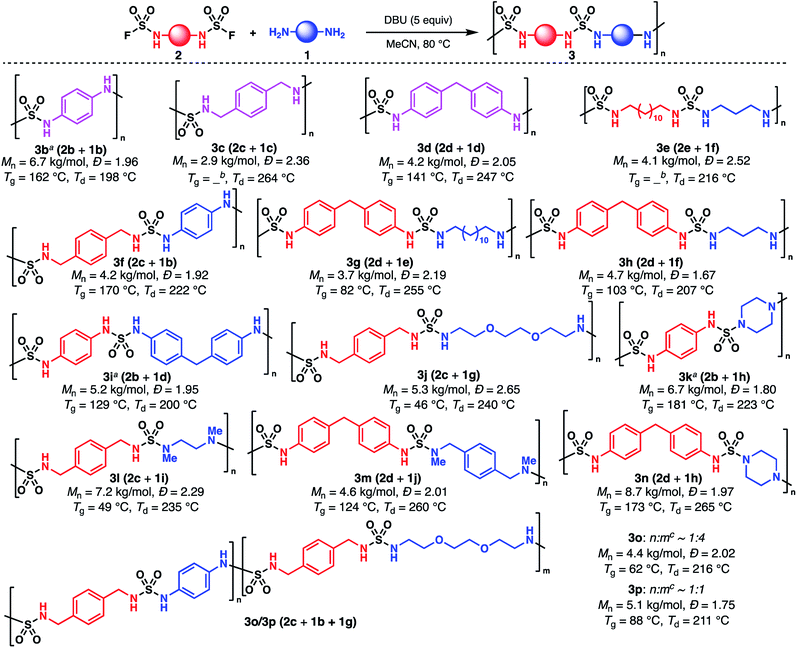 |
| Fig. 4 Synthesized polysulfamides via SuFEx chemistry. Mn's and Đ's were determined by SEC (DMAc + 5% LiCl) using poly(methyl methacrylate) standards. Td = 5% weight loss temperature. aPyridine was used instead of DBU. bNo clear Tg was observed by DSC. cDetermined by 1H NMR. | |
The synthesized polysulfamides showcased high thermal stability, a desirable feature for many applications. However, the global accumulation of plastic waste has created a dire environmental crisis that must be addressed by the development of recyclable polymers. Knowing the hydrolytic stability of polyureas,51 the depolymerization of the synthesized polysulfamides was investigated. While alkyl polysulfamide 3l displayed remarkable stability in acidic or basic media at elevated temperatures (ESI†), aryl polysulfamide 3b could be hydrolyzed in a variety of aqueous conditions.52,533b was typically suspended in an aqueous solution and heated for 40 h. The resulting aqueous solution was then extracted at pH ∼ 14 with EtOAc and the amount of bis(amine) monomer 1b was quantified (Table 2). After treatment with a basic solution containing NaOH or NH4OH for 40 h at 80 °C or 125 °C, up to 53% of pure 1b was isolated, while in HCl at 125 °C for the same amount of time, the recovery was improved to 74%. This initial study will serve as a blueprint to investigate the recyclability potential of aromatic polysulfamides.
Table 2 Hydrolysis of aryl polysulfamides
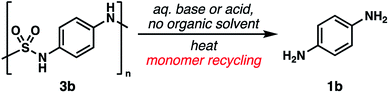
|
Entrya |
Aq. base/acid |
Temp. (°C) |
Monomer recoveryb (%) |
Hydrolysis reactions were conducted for 40 h.
Based on isolated 1b.
Some polymer remained in suspension.
|
1 |
NaOH (4 M) |
80 |
32 |
2 |
NaOH (4 M) |
125 |
42 |
3 |
NH4OH (18 M) |
80 |
53 |
4 |
HCl (4 M) |
80 |
63c |
5 |
HCl (4 M) |
125 |
74 |
Conclusions
In summary, we have developed an efficient, practical, and general synthesis of sulfamides and polysulfamides based on SuFEx click chemistry. We anticipate that this robust and mild method will impact the fields of medicinal chemistry and hydrogen-bond catalysis, areas in which sulfamides have demonstrated exciting promise. This process was adapted to produce and evaluate polysulfamides, a virtually unknown class of polymers. Characterization of these polymers revealed a high thermal stability as well as modular Tg's depending on the monomer structure. IR spectroscopy and power X-ray diffraction revealed that hydrogen-bonding interactions and the degree of crystallinity are strongly influenced by the structure of the polymer backbone. Finally, aromatic polysulfamide 3b was depolymerized upon heating in basic or acidic aqueous solution, which suggests that these materials could be efficiently recycled after use.
Conflicts of interest
There are no conflicts to declare.
Acknowledgements
This work was supported by Texas A&M University, made use of the NMR, X-ray Diffraction, and Mass Spectrometry facilities in the Department of Chemistry and the Soft Matter Facility (TAMU). The authors acknowledge the Welch Foundation (A-2004-20190330) for financial support. Dr David Truong (Soft Matter Facility), PolyAnalytik Inc., Dr Joseph Reibenspies, Dr Nattamai Bhuvanesh, and Katelynn Edgehouse are acknowledged for technical assistance with polymer characterization.
Notes and references
- J. Dong, L. Krasnova, M. G. Finn and K. B. Sharpless, Angew. Chem., Int. Ed., 2014, 53, 9430–9448 CrossRef CAS PubMed.
- T. Abdul Fattah, A. Saeed and F. Albericio, J. Fluorine Chem., 2018, 213, 87–112 CrossRef CAS.
- A. S. Barrow, C. J. Smedley, Q. Zheng, S. Li, J. Dong and J. E. Moses, Chem. Soc. Rev., 2019, 48, 4731–4758 RSC.
- J. Dong, K. B. Sharpless, L. Kwisnek, J. S. Oakdale and V. V. Fokin, Angew. Chem., Int. Ed., 2014, 53, 9466–9470 CrossRef CAS PubMed.
- C. Yang, J. P. Flynn and J. Niu, Angew. Chem., Int. Ed., 2018, 57, 16194–16199 CrossRef CAS PubMed.
- H. Wan, S. Zhou, P. Gu, F. Zhou, D. Lyu, Q. Xu, A. Wang, H. Shi, Q. Xu and J. Lu, Polym. Chem., 2020, 11, 1033–1042 RSC.
- H. Fan, Y. Ji, Q. Xu, F. Zhou, B. Wu, L. Wang, Y. Li and J. Lu, ChemPlusChem, 2018, 83, 407–413 CrossRef CAS PubMed.
- H. Wang, F. Zhou, G. Ren, Q. Zheng, H. Chen, B. Gao, L. Klivansky, Y. Liu, B. Wu, Q. Xu, J. Lu, K. B. Sharpless and P. Wu, Angew. Chem., Int. Ed., 2017, 56, 11203–11208 CrossRef CAS PubMed.
- B. Gao, L. Zhang, Q. Zheng, F. Zhou, L. M. Klivansky, J. Lu, Y. Liu, J. Dong, P. Wu and K. B. Sharpless, Nat. Chem., 2017, 9, 1083–1088 CrossRef CAS PubMed.
- B. Gong, C. Zheng, E. Skrzypczak-Jankun, Y. Yan and J. Zhang, J. Am. Chem. Soc., 1998, 120, 11194–11195 CrossRef CAS.
- B. Gong, C. Zheng, E. Skrzypczak-Jankun and J. Zhu, Org. Lett., 2000, 2, 3273–3275 CrossRef CAS PubMed.
- N. Maeda, K. Masuda, J. Li, S.-i. Kabashima, I. Yoshikawa and K. Araki, Soft Matter, 2010, 6, 5305–5307 RSC.
- S.-i. Kabashima, S. Tanaka, M. Kageyama, I. Yoshikawa and K. Araki, Langmuir, 2011, 27, 8950–8955 CrossRef CAS PubMed.
- S.-i. Kabashima, M. Kageyama, T. Okano, I. Yoshikawa and K. Araki, J. Colloid Interface Sci., 2013, 408, 107–112 CrossRef CAS PubMed.
- A. B. Reitz, G. R. Smith and M. H. Parker, Expert Opin. Ther. Pat., 2009, 19, 1449–1453 CrossRef CAS PubMed.
- T. Mazzei, J. Chemother., 2010, 22, 219–225 CrossRef CAS PubMed.
- K. Han Ahn, D. Jin Yoo and J. Sook Kim, Tetrahedron Lett., 1992, 33, 6661–6664 CrossRef.
- A. A. Rodriguez, H. Yoo, J. W. Ziller and K. J. Shea, Tetrahedron Lett., 2009, 50, 6830–6833 CrossRef CAS PubMed.
- X.-j. Zhang, S.-p. Liu, X.-m. Li, M. Yan and A. S. C. Chan, Chem. Commun., 2009, 833–835 RSC.
- F. Deng and H.-Y. Liu, Synth. Commun., 2012, 42, 767–774 CrossRef CAS.
- P. B. Cranwell, J. R. Hiscock, C. J. E. Haynes, M. E. Light, N. J. Wells and P. A. Gale, Chem. Commun., 2013, 49, 874–876 RSC.
- A. V. Leontiev, H. V. Rasika Dias and D. M. Rudkevich, Chem. Commun., 2006, 2887–2889 RSC.
- A. Vandi, T. Moeller and L. F. Audrieth, J. Org. Chem., 1961, 26, 3478–3480 CrossRef CAS.
- H. Q. Smith and F. L. Scott, J. Polym. Sci., Part A: Gen. Pap., 1964, 2, 481–487 CrossRef CAS.
- R. A. Florentine, G. Barth-Wehrenalp, I. Mockrin, I. Popoff and R. Riordan, J. Polym. Sci., Part A: Gen. Pap., 1964, 2, 489–502 CrossRef CAS.
- These conditions were recently used to produce two porous polymers: P. Zhang, D. Chen, N. Chen, K. Huang, D. Tao, M. Li and S. Dai, ChemSusChem, 2018, 11, 1751–1755 CrossRef CAS PubMed.
-
G. Odian, Principles of Polymerization, Wiley, Hoboken, NJ, 4th edn, 2004 Search PubMed.
- For a general review on sulfamic acid derivatives including sulfamides and their synthesis, see W. Spillane and J.-B. Malaubier, Chem. Rev., 2014, 114, 2507–2586 CrossRef CAS PubMed . Below are selected examples of sulfamide syntheses based on electrophilic SO2-containing reagent.
- For an early example of the sequential addition of two amines to sulfuryl chloride, see A. Vandi, T. Moeller and L. F. Audrieth, J. Org. Chem., 1961, 26, 1136–1138 CrossRef CAS.
- For an example of the use of chlorosulfonyl isocyanate, see Y. Iso, T. Irie, T. Iwaki, M. Ku, Y. Sendo, K. Motokawa and Y. Nishitani, J. Antibiot., 1996, 49, 478–484 CrossRef CAS PubMed.
- For the seminal study of phenolate as leaving group, see G. E. DuBois, J. Org. Chem., 1980, 45, 5373–5375 CrossRef CAS.
- For a recent example involving 4-nitrophenolate as leaving group, see K. J. Fettes, N. Howard, D. T. Hickman, S. Adah, M. R. Player, P. F. Torrence and J. Micklefield, J. Chem. Soc., Perkin Trans. 1, 2002, 485–495 RSC.
- For a recent example based on sulfuryldiimidazole electrophiles, see S. Beaudoin, K. E. Kinsey and J. F. Burns, J. Org. Chem., 2003, 68, 115–119 CrossRef CAS PubMed.
- For an example involving a Lossen-like rearrangement of L. Pantaine, see F. Richard, J. Marrot, X. Moreau, V. Coeffard and C. Greck, Adv. Synth. Catal., 2016, 358, 2012–2016 CrossRef.
- For a recent example involving 2-oxazolidone as leaving group, see T. Duhamel, M. D. Martínez, I. K. Sideri and K. Muñiz, ACS Catal., 2019, 9, 7741–7745 CrossRef CAS.
- For a recent example involving 2,6-difluorophenolate as leaving group, see M. M. Pompeo, J. H. Cheah and M. Movassaghi, J. Am. Chem. Soc., 2019, 141, 14411–14420 CrossRef CAS PubMed.
- For a recent example based on sulfamic acid salts activation, see M. F. Shehata, M. A. Short, M. A. Sanders and J. L. Roizen, Tetrahedron, 2019, 75, 3186–3194 CrossRef CAS.
-
J. Dong and K. B. Sharpless, Sulfur(VI) fluoride compounds and methods for the preparation of thereof, Patent WO2015188120A1, December 10, 2015.
- D. K. Padma, V. Subrahmanya Bhat and A. R. Vasudeva Murthy, J. Fluorine Chem., 1982, 20, 425–437 CrossRef CAS.
- While this manuscript was under review, a synthesis of tri- and tetrasubsituted sulfamides using SuFEx was published by S. Mahapatra, C. P. Woroch, T. W. Butler, S. N. Carneiro, S. C. Kwan, S. R. Khasnavis, J. Gu, J. K. Dutra, B. C. Vetelino, J. Bellenger, C. W. am Ende and N. D. Ball, Org. Lett., 2020, 22, 4389–4394 CrossRef CAS PubMed.
- C. Veryser, J. Demaerel, V. Bieliūnas, P. Gilles and W. M. De Borggraeve, Org. Lett., 2017, 19, 5244–5247 CrossRef CAS PubMed.
- W. J. Spillane, F. A. McHugh and P. O. Burke, J. Chem. Soc., Perkin Trans. 2, 1998, 13–18 RSC.
- F. Liu, H. Wang, S. Li, G. A. L. Bare, X. Chen, C. Wang, J. E. Moses, P. Wu and K. B. Sharpless, Angew. Chem., Int. Ed., 2019, 58, 8029–8033 CrossRef CAS PubMed.
- T. Guo, G. Meng, X. Zhan, Q. Yang, T. Ma, L. Xu, K. B. Sharpless and J. Dong, Angew. Chem., Int. Ed., 2018, 57, 2605–2610 CrossRef CAS PubMed . A single example of (trisubstituted) sulfamide synthesis was reported in this communication as well.
- H. Zhou, P. Mukherjee, R. Liu, E. Evrard, D. Wang, J. M. Humphrey, T. W. Butler, L. R. Hoth, J. B. Sperry, S. K. Sakata, C. J. Helal and C. W. am Ende, Org. Lett., 2018, 20, 812–815 CrossRef CAS PubMed.
- At room temperature, the reaction still proceeded to high yields but failed to reach full conversion even after 24 h (Tables S3 and S4†).
- T. G. Fox, Bull. Am. Phys. Soc., 1956, 1, 123 CAS.
- Y. Yanagisawa, Y. Nan, K. Okuro and T. Aida, Science, 2018, 359, 72–76 CrossRef CAS PubMed.
- R. Custelcean, Chem. Commun., 2008, 295–307 RSC.
- C. Stefaniu, P.-L. Zaffalon, A. Carmine, Q. Verolet, S. Fernandez, T. A. Wesolowski, G. Brezesinski and A. Zumbuehl, Langmuir, 2015, 31, 1296–1302 CrossRef CAS PubMed.
- V. Sendijarevic, A. Sendijarevic, I. Sendijarevic, R. E. Bailey, D. Pemberton and K. A. Reimann, Environ. Sci. Technol., 2004, 38, 1066–1072 CrossRef CAS PubMed.
- R. G. Cornwall, B. Zhao and Y. Shi, Org. Lett., 2013, 15, 796–799 CrossRef CAS PubMed.
- Y. Bekdemir, A. Gediz Erturk and H. Kutuk, J. Phys. Org. Chem., 2014, 27, 94–98 CrossRef CAS.
Footnotes |
† Electronic supplementary information (ESI) available. See DOI: 10.1039/d0sc03606d |
‡ R. W. K. and J. W. W. contributed equally to this study. |
|
This journal is © The Royal Society of Chemistry 2020 |