DOI:
10.1039/D0SC03215H
(Edge Article)
Chem. Sci., 2020,
11, 10159-10166
Extension of the Simmons–Smith reaction to metal-carbynes: efficient synthesis of metallacyclopropenes with σ-aromaticity†
Received
9th June 2020
, Accepted 31st August 2020
First published on 3rd September 2020
Abstract
The Simmons–Smith reaction offers a direct route for conversion of an alkene into a cyclopropane with a zinc carbenoid as the active intermediate. Zinc carbenoids, however, have never delivered a methylene unit to substrates with metal–carbon multiple bonds. Herein, we describe this type of reaction and the construction of three-membered rings has now been applied in organometallic systems by combining classical zinc carbenoid reagents with a range of structurally and electronically diverse metal carbynes. A variety of metallacyclopropene derivatives prepared in this way represent rare examples with σ-aromaticity in an unsaturated three-membered ring. The structures of such products are supported by experimental observations and theoretical calculations.
Introduction
Molecules incorporating three-membered rings have fascinated chemists for decades, and synthetic chemists have developed elegant strategies for the construction of these three-membered ring systems. Popular strategies include [2 + 1]-type cycloadditions between carbon–carbon multiple bonds and reactive reagents, such as carbene equivalents.1–7 The Simmons–Smith reaction (Scheme 1) using carbene equivalents generated from zinc–copper couples and dihalomethanes was first reported over half a century ago but today remains one of the most powerful methods for converting an alkene into a cyclopropane.8,9 Several advances have addressed the limitations of the initial Simmons–Smith protocol.10–16 For example, Furukawa et al. used ZnEt2 to generate the active carbenoid reagent more reliably and quantitatively.17,18 Acidic additives, such as CF3CO2H, were found by Shi et al. to accelerate the cyclopropanation of challenging substrates.19 Significant research efforts have been devoted toward Zn carbenoid chemistry, but metal–carbon multiple bonds have never been successfully employed in the Simmons–Smith reaction.
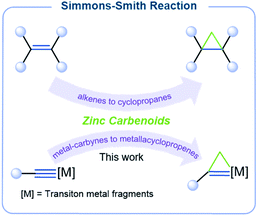 |
| Scheme 1 Extension of the Simmons–Smith reaction. | |
A different approach to the synthesis of such compounds is based on the introduction of metal–carbon multiple bonds, which would form strained three-membered metallacycles, such as metallacyclopropanes20 or metallacyclopropenes.21 Strained three-membered metallacycles are reactive moieties and can be diversified into other frameworks through ring–opening reactions which relieve strain in the three-membered ring.22 For example, highly strained metallacyclopropenes are extremely attractive because they can serve as precursors for efficient synthesis of highly functionalized organic molecules and heterocyclic compounds.23 The Simmons–Smith reaction is potentially well-suited to prepare these structural motifs; however, it is known that it is inapplicable to alkynes, negating this synthetic strategy towards the corresponding cyclopropenes. In our exploration of unique metallacyclic systems, the chemistry of metallapentalynes was investigated. In such reactions, the metal–carbon triple bonds in a five-membered ring exhibit unique reactivity and can lead to the formation of novel three-membered metallacycles, such as halogenium ions within an unsaturated three-membered ring,24 metallacyclopropenimine intermediates,25 and metallaazirines.26 As such, we predicted that metallapentalynes could also serve as suitable substrates with which to extend the classical Simmons–Smith reaction.
For the first time, we applied the Simmons–Smith reaction to metal-carbyne systems and found that the cyclopropenation reactions of metal-carbynes involve the “butterfly-type” transition states (TS) shown in Fig. 1. We found that reaction of a ruthenium-carbyne derivative with Shi's reagent afforded ruthenacyclopropene, which presumably occurred through a metal–carbon triple bond shift. Density functional theory (DFT) calculations demonstrate that the resulting metallacyclopropenes are rare examples of σ-aromaticity in an unsaturated three-membered ring.
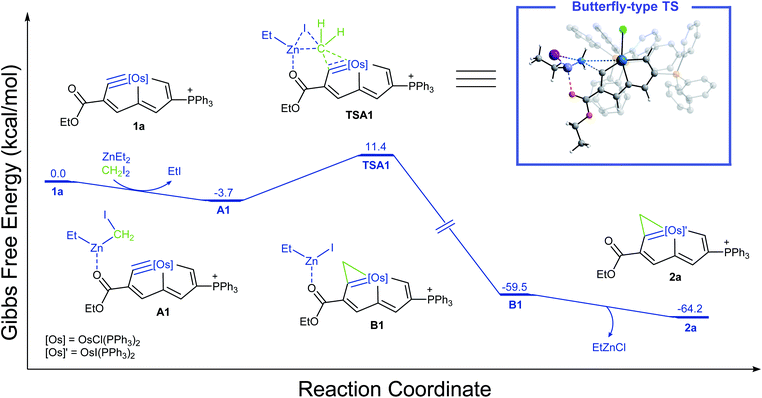 |
| Fig. 1 Gibbs free-energy pathway for the DFT-calculated formation mechanism of complex 2a at 298 K (the energies are given in kcal mol−1). The ωB97X-D level of DFT with a def2-TZVP basis set was used. | |
DFT computational methods
Structure 7′ was optimized at the B3LYP/6-311++G** level of DFT27 with an SDD basis set28 describing P, Cl, Ru, I and Os atoms, whereas the other structures were optimized at the B3LYP/6-31G* level with an SDD basis set describing P, Cl, Zn, Ru, I and Os atoms; our single-point energy calculations were performed on the mechanism at the ωB97X-D/def2-TZVP level29,30 with the Solvation Model based on Density (SMD)31 in dichloromethane. Frequency calculations were performed to identify all the stationary points as minima (zero imaginary frequency). The energies are given in kcal mol−1 and include the zero-point energy corrections. NBO calculations were carried out at the B3LYP/6-31G* level with an SDD basis set to describe P, Cl, Zn, Ru, I and Os atoms with the NBO 6.0 program.32 Nucleus-independent chemical shift (NICS)33–35 values were calculated at the B3LYP/6-311++G** level of theory. All the above calculations were performed with the Gaussian 09 software package.36 Calculations of the anisotropy of the induced current density (AICD)37,38 were carried out with the AICD program.37
Results and discussion
Simmons–Smith reaction of cyclic osmium-carbynes with Furukawa's reagent
Previous reports of mechanistic studies on Simmons–Smith cyclopropanations were consistent with a concerted [2 + 1] methylene transfer process leading to a transition state corresponding to a three-centered structure.39–45 In an effort to optimize the conversion of the metal-carbyne bond of metallapentalyne (1) to metallacyclopropene-fused metallapentalene (2) and gain insights into the reaction mechanism, we first used quantum mechanical calculations. Osmapentalyne (1a), in which the ethoxycarbonyl group is located adjacent to the osmium-carbyne, was selected as a starting material for the model (Fig. 1). Initially, reaction of 1a with ZnEt2 and CH2I2 could form the active complex A1, in which the ethoxycarbonyl group is coordinated with the zinc atom. From the intermediate (A1), the corresponding cyclopropenation was achieved via the “butterfly-type” transition state (TSA1) with an activation free energy of 15.1 kcal mol−1, producing the intermediate B1. Elimination of a zinc complex could then produce the desired fused-metallacyclopropene product. The computed free-energy profile of the reaction also indicates that the stability of the resulting fused-metallaaromatics is crucial in the overall reaction, which could be classified as aromaticity-driven. Reaction of the cyclic osmium-carbyne (1a) with excess ZnEt2/CH2I2 (Furukawa's reagent)17,18 at room temperature (RT) in dichloromethane resulted in consumption of the starting material within 6 h (Scheme 2) and production of compound 2a, which was isolated as a yellow powder in 78% yield. Additionally, osmapentalyne 1 is unreactive to CH2I2 in the absence of ZnEt2 (Fig. S44 and S45†). The 1H NMR spectrum of 2a displays three characteristic signals at 14.82, 9.14, and 8.67 ppm, which are downfield from those of the starting material 1a (13.32, 8.99, and 8.10 ppm).46 The high-field chemical shifts of H8 and C8 in 2a suggest that C8 is a newly formed sp3-hybridized carbon: the signal at δ = 3.21 ppm in the 1H NMR spectrum is associated with that at δ = 18.8 ppm in the 13C{1H} NMR spectrum, as indicated in the 1H–13C HSQC NMR spectrum, which contains peaks similar to those observed for osmacyclopropene fused with osmapentalene (δ = 3.03 and 21.8 ppm47 and δ = 3.03 and 23.1 ppm,48 respectively).
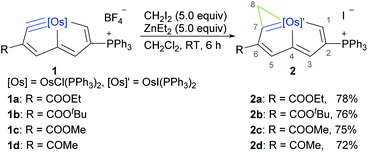 |
| Scheme 2 Synthesis of complex 2via the Simmons–Smith reaction with Furukawa's reagent. | |
Single crystals of 2a (Fig. 2) suitable for an X-ray diffraction study were grown by slow vapor diffusion of hexane in a saturated CHCl3 solution. The X-ray data confirmed that the newly formed metallacyclopropene unit is fused to the original bicyclic system with Os1–C7, Os1–C8 and C7–C8 bond lengths of 1.991(4), 2.288(4), and 1.361(6) Å, respectively. The nine atoms (Os1 and C1–C8) in 2a are approximately coplanar, the mean deviation from the least–squares plane being 0.022 Å. The sum of the angles in the fused five-membered rings (540.0° of both) are in good agreement with the ideal values of 540°. The bond lengths within the osmacyclopropene ring in complex 2a are all similar to those reported for fused osmacyclopropenes (1.927–2.015 Å for Os
C and 2.196–2.272 Å for Os–C, respectively).47–49 The original Cl ligand of the metal center has been replaced by an I ligand. This might be attributed to the presence of excess Furukawa's reagent (ZnEt2/CH2I2) in the reaction.
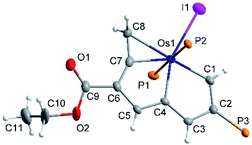 |
| Fig. 2 X-ray molecular structure for the cation of complex 2a drawn at the 50% probability level. The phenyl groups are omitted for clarity. Selected bond lengths (Å) and angles (°): Os1–C1 2.034(4), Os1–C4 2.089(4), Os1–C7 1.991(4), Os1–C8 2.288(4), C1–C2 1.387(6), C2–C3 1.416(5), C3–C4 1.380(5), C4–C5 1.401(5), C5–C6 1.388(6), C6–C7 1.381(6), C7–C8 1.361(6); Os1–C1–C2 119.8(3), C1–C2–C3 113.5(3), C2–C3–C4 113.3(3), C3–C4–Os1 118.3(3), C4–Os1–C1 75.12(15), Os1–C4–C5 119.5(3), C4–C5–C6 112.5(3), C5–C6–C7 110.5(3), C6–C7–Os1 125.3(3), C7–Os1–C4 72.24(16), Os1–C7–C8 83.9(3), C7–C8–Os1 59.9(2), C7–Os1–C8 36.24(16). | |
Using this method, the reaction of Furukawa's reagent with a series of cyclic osmium-carbyne substrates containing tert-butoxycarbonyl, methoxycarbonyl, and acetyl was found to afford the expected cyclopropene products (2b–2d) in good isolated yields (72–76%). The results showed compatibility and efficiency of extending the Simmons–Smith reaction to the osmium-carbyne system. These annulation products (2) exhibit good thermal stability both in solution and in the solid state. For example, the solid sample of 2a can survive for several months when exposed to air at RT or even when heated at 100 °C in air for 3 h.
Simmons–Smith reaction of cyclic osmium-carbyne with Shi's reagent
On the basis of DFT calculations, the “butterfly-type” TS of the cyclopropenation reaction has the zinc bonded to the carbonyl oxygen atom. Thus, we were curious to see if cyclic osmium-carbynes with different groups, for example hydroxyalkyl, would react with zinc carbenes. The cyclic osmium carbyne complex with hydroxymethyl (3a) or hydroxy(phenyl)methyl (3b) can be obtained by reacting osmium complex S1 (ref. 50) with propargyl alcohol or 1-phenylprop-2-yn-1-ol, respectively, and excess PPh3 at RT for 3 h (see the ESI† for details). The cyclopropenylation of the osmapentalynes (3) was first attempted with Furukawa's reagent (CH2I2/ZnEt2). Monitored in situ by NMR, the reaction proceeded with some decomposition of the starting material, but without any desired cyclopropenation product. Reaction of 3 with CF3COOH/CH2I2/ZnEt2 (Shi's reagent),19 however, led to complete conversion, as determined by 1H NMR spectroscopy, furnishing 4 with the help of the more reactive zinc carbenoid precursor, CF3COOZnCH2I. Compounds 4a and 4b were isolated in 70% and 73% yield, respectively (Scheme 3). However, the cyclic osmium carbyne complex 5 was unreactive to either Furukawa's reagent or Shi's reagent, which might be attributed to the electronic effect.
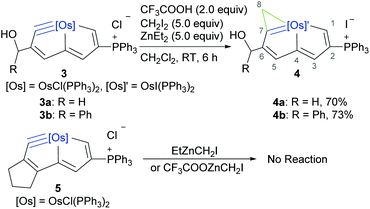 |
| Scheme 3 Synthesis of complex 4 via the Simmons–Smith reaction with Shi's reagent. | |
Complexes 4a and 4b were characterized spectroscopically and analytically. The osmacyclopropene rings were shown by a sharp singlet appearing in the 1H NMR spectra (3.04 ppm for 4a; 3.32 ppm for 4b) and 13C NMR spectra (232.9 ppm (C7) and 19.4 ppm (C8) for 4a; 229.1 ppm (C7) and 27.2 ppm (C8) for 4b). The 1H NMR spectra of 4 also display diagnostic broad peaks in the hydroxyl region at 4.13 (4a) and 3.91 (4b) ppm, with integration showing one proton each.
Simmons–Smith reaction of cyclic ruthenium-carbyne
Building on the aforementioned cyclopropenation of osmium-carbynes, subsequent efforts were directed towards extension of the Simmons–Smith reaction to other metal-carbyne complexes. The application of Furukawa's reagent to ruthenium-carbyne (6) gave no detectable cyclopropenation product under the same reaction conditions used for osmium-carbyne (1), as monitored by in situ NMR. When Shi's reagent was allowed to react with ruthenium-carbyne (6) at 0 °C, the expected cyclopropenation occurs. The addition of an excess of the chlorine anion species, Bu4NCl, increased the yield of the cyclopropenation product, which may retain the chlorine ligand of the metal center. The formation of complex 7 may involve a stepwise pathway, since the gradual addition of Shi's reagent can increase the yield, affording the complex (7) which was isolated as a brown solid in 55% yield (Scheme 4).
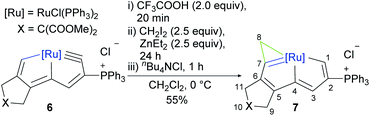 |
| Scheme 4 Synthesis of the metallacyclopropene complex (7) via the Simmons–Smith reaction with Shi's reagent. | |
Complex 7 was fully characterized spectroscopically and by X-ray diffraction studies. In the 1H NMR spectrum of complex 7, the doublet signal at 13.91 ppm was attributed to the proton at C1, and the other proton at C3 of the fused five-member metallacycle (H3) was observed at 7.72 ppm. The proton signal of the newly formed metallacyclopropene ring was observed at 3.67 ppm, which is comparable to 4.06 ppm in the only reported ruthenacyclopropene.51 In the 13C NMR spectrum of 7, the characteristic signals of the tricyclic framework were observed at 269.4 (C1), 250.6 (C7), 202.4 (C4), 194.4 (C5), 153.7 (C6), 150.0 (C3), 129.9 (C2), and 45.8 (C8) ppm. The NMR spectra confirmed the similarity of 7 to the osmacyclopropene-fused complexes 2 and 4.
After several days at RT, a concentrated chloroform solution of 7 gave rise to brown crystals adequate for X-ray analysis. X-ray crystallography confirmed the structure of 7 as a cycloproparuthenapentalene (Fig. 3). One of the five-membered metallapentalene rings and the three-membered metallacyclopropene ring are linked together by the Ru atom which is nearly coplanar with C1–C8; the mean deviation from the least–squares plane of Ru1 and C1–C8 is only 0.026 Å. The sum of the interior angles of the fused five-membered rings (540.0° of both) is exactly the same as the ideal values of 540°. The bond lengths of Ru1–C7 (2.030(4) Å) are much shorter than those of Ru1–C8 (2.308(4) Å). These bond lengths are quite similar to those in the ruthenabenzene-fused ruthenacyclopropene ring (2.043(8) and 2.219(9) Å), while the C7–C8–Ru1 angle (60.9(2)o) is smaller than the C–C–Ru angle (64.2(5)o) in the ruthenabenzene-fused ruthenacyclopropene ring.51 It is noteworthy that compound 7 is the first example of a ruthenacyclopropene with the coplanar fused-metallaaromatic ring system. It is different from the only reported ruthenacyclopropene example, in which the ruthenacyclopropene ring deviates considerably from the fused ruthenabenzene ring. The cycloproparuthenapentalene 6 possesses a coplanar tricyclic ring system.
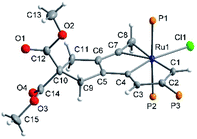 |
| Fig. 3 X-ray molecular structure for the cation of complex 7 drawn at the 50% probability level. The phenyl groups are omitted for clarity. Selected bond lengths (Å) and angles (o): Ru1–C1 2.012(4), Ru1–C4 2.067(4), Ru1–C7 2.027(4), Ru1–C8 2.315(4), C1–C2 1.385(5), C2–C3 1.412(5), C3–C4 1.375(5), C4–C5 1.411(5), C5–C6 1.379(6), C6–C7 1.377(6), C7–C8 1.356(6); Ru1–C1–C2 119.5(3), C1–C2–C3 113.6(3), C2–C3–C4 113.0(4), C3–C4–Ru1 118.2(3), C4–Ru1–C1 75.72(15), Ru1–C4–C5 117.9(3), C4–C5–C6 113.7(4), C5–C6–C7 112.6(3), C6–C7–Ru1 121.4(3), C7–Ru1–C4 74.40(16), Ru1–C7–C8 83.9(3), C7–C8–Ru1 60.5(2), C7–Ru1–C8 35.62(16). | |
X-ray diffraction studies of 7 clearly reveal that the cyclopropenation does not occur at the original metal-carbyne position, but rather in the five-membered ring with the oxygen-containing group. For a better understanding of the mechanistic pathway of the Simmons–Smith reaction of cyclic ruthenium-carbyne 6, DFT calculations were performed at the SMD-ωB97X-D/Def2-TZVP (Fig. 4). Initially, in the presence of acid, the Ru–C triple bond of 6 could shift from the original ring to the other fused ring via the ruthenapentalene intermediate (A2), which could be observed by in situ NMR experiments (Fig. S42 and S43†).
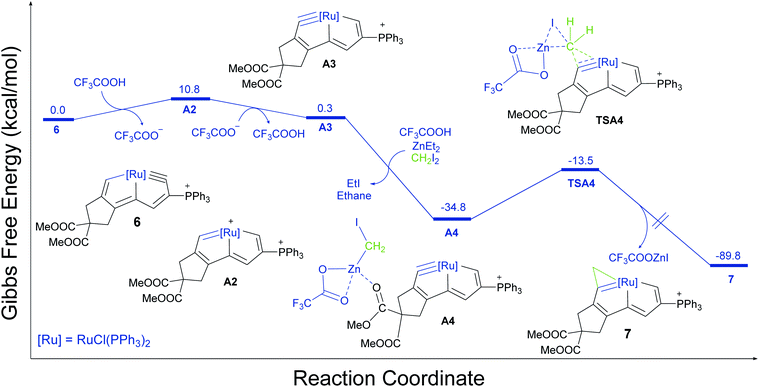 |
| Fig. 4 Gibbs free-energy pathway for the DFT-calculated formation mechanism of complex 7 at 273 K (the energies are given in kcal mol−1). The ωB97X-D level of DFT with a def2-TZVP basis set was used. | |
This type of extraordinary metal carbyne bond shift reaction has been observed and isotopic-labeling experiments suggest that the hydrogen atom at the original carbyne carbon position in the product originates from the acid proton.52 Subsequent coordination of oxygen with zinc affords an intermediate (A4, −34.8 kcal mol−1). A transition state (TSA4) with “butterfly-type” conformation has been identified with an activation barrier of 21.3 kcal mol−1, leading to the formation of the cyclopropenation product (7, −89.8 kcal mol−1). Notably, acidic additives such as CF3CO2H in this observed ring expansion pathway act not only as the auxiliary reagent activating carbenoid reagent and accelerating the cyclopropanation, but also facilitate the metal carbyne bond shift to produce the ruthenium-carbyne with the adjacent oxygen-containing group. In addition, the natural bond orbital (NBO) charge at the metal centres decreases from Os(1) to Ru(6), but the NBO charge at the related carbyne atoms increases (Fig. S7†). Thus, our combined experimental and computational studies demonstrated that the electronic properties of the metal-carbynes are very important for the formation of the metallacyclopropenes.
Evaluation of the σ-aromaticity of fused ruthenacyclopropenes by DFT calculations
Metallacycles with σ-aromaticity arising from the delocalization of σ-bonds are uncommon, and only a handful of osmacyclopropenes fused with osmapentalene,46,47 osmafuran,53 and osmanaphthelene48 have been reported. Since a new class of metallacyclopropenes, fused ruthenacyclopropene, has been obtained, we performed DFT calculations to elucidate the nature of the aromaticity in the ruthenacyclopropene ring. For the model compound (7′) we calculated the nucleus independent chemical shifts (NICS)33–35 in the ring and along the z axis at 1 Å above the ring critical point [NICS(1)zz] (the average value was used when the environments above and below the ring centers were not equivalent). These data were simplified by replacing the PPh3 groups with PH3 groups (Fig. 5A). The NICS(0) (a: −2.5 ppm; b: −0.3 ppm; c: −40.5 ppm) and NICS(1)zz (a: −14.0 ppm; b: −5.6 ppm; c: −26.0 ppm) values unambiguously support the aromatic nature of the three fused metallacycles (GIAO-B3LYP/6-311++g**, with an SDD basis set to describe P, Cl, Ru, and I atoms). The computed NICS value for the three-membered ring in 7′ was found to be much more negative than those of the five-membered rings, and the NICS values of the three metallacycles were in good agreement with those of previously reported fused osmacyclopropenes.46–48 Indeed, canonical molecular orbital (CMO) nucleus-independent chemical shift (NICS) calculations23–35 show that the contribution of the NICS(0) value for the ruthenacyclopropene ring of 7′ from the five occupied π-molecular orbitals (HOMO-2, HOMO-4, HOMO-5, HOMO-12 and HOMO-14; see Fig. 5) is +7.8 ppm, whereas the contribution from all the σ orbitals is much more negative (−48.3 ppm), indicating the σ-aromaticity in the ruthenacyclopropene ring.
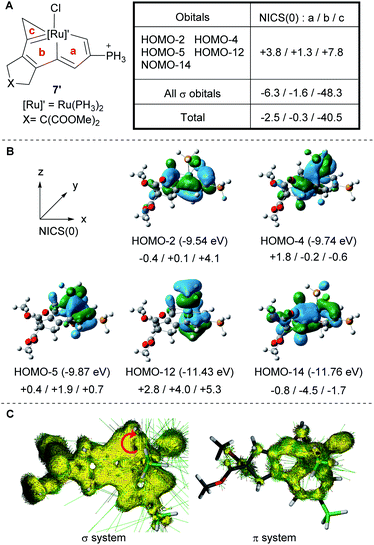 |
| Fig. 5 Aromaticity evaluation. (A) NICS(0) values for the model complex 7′; (B) key occupied π MOs and their energies together with contributions to NICS(0): the eigenvalues of the MOs are given within parentheses in the first line and the NICS(0) values of rings a, b, and c are given in the second line; (C) AICD isosurfaces of 7′ separated into the σ and π contribution. Current density vectors are plotted on the AICD isosurface of 0.025 to indicate diatropic and paratropic ring currents. The magnetic field vector is orthogonal with respect to the ring plane and is directed upwards (clockwise currents are diatropic). | |
In addition, the σ-aromaticity in the ruthenacyclopropene ring of 7′ is further supported by the anisotropy of the induced current density (AICD) analysis. As shown in Fig. 5C, the current density vectors plotted on the AICD isosurface of 7′ indicated a diatropic ring current (clockwise vectors) and are only observed in the σ system of the ruthenacyclopropene ring. Thus, complex 7 can be regarded as the first example of a σ-aromatic metallacyclopropene complex with a second-row transition metal center. It is interesting that a great number of metalla-aromatic species contain third-row transition metals, whereas only a few contain first- and second-row transition metal centers. Considering that the strength of transition metal–carbon bonds decreases when ascending the column in the periodic table, it is usual to see the relative lability of the first- and second-row organometallic compounds in comparison with their third-row analogs. Ruthenacyclopropene (7) represents an important complement to the metalla-aromatic family and distinguishes itself by appearing as a ruthenacyclopropene skeleton in a fused aromatic ring system.
Conclusions
In summary, we developed some [2 + 1] cycloaddition reactions of metal–carbon multiple bonds that involve the use of classical zinc carbenoid reagents for the preparation of three-membered metallacycles in a one-pot reaction under mild conditions. These reagents can be used to react on the gram scale with a series of osmium-carbyne complexes and a ruthenium-carbyne complex, affording fused metallacyclopropenes with σ-aromaticity. Our theoretical and experimental studies have revealed that the electronic properties of the metal-carbynes have substantial roles in the high efficiency and robust characteristics of these extended Simmons–Smith reactions. Such a strategy utilizing classical zinc carbenoid reagents could gain widespread use as a promising complement to existing techniques for the preparation of polycyclic organometallic complexes and will promote the discovery of new, challenging and complex aromatic molecular architectures.
Conflicts of interest
The authors declare no conflict of interest.
Acknowledgements
This research was supported by the National Natural Science Foundation of China (U1705254, 21871225 and 21931002) and the Fundamental Research Funds for the Central Universities (20720190042).
Notes and references
- M. Lautens, W. Klute and W. Tam, Chem. Rev., 1996, 96, 49–92 CrossRef CAS.
- H. Lebel, J.-F. Marcoux, C. Molinaro and A. B. Charette, Chem. Rev., 2003, 103, 977–1050 CrossRef CAS.
- J. M. Concellon, H. Rodriguez-Solla, C. Concellon and V. del Amo, Chem. Soc. Rev., 2010, 39, 4103–4113 RSC.
- R. G. Cornwall, O. A. Wong, H. Du, T. A. Ramirez and Y. Shi, Org. Biomol. Chem., 2012, 10, 5498–5513 RSC.
- A. Archambeau, F. Miege, C. Meyer and J. Cossy, Acc. Chem. Res., 2015, 48, 1021–1031 CrossRef CAS.
- D. Qian and J. Zhang, Chem. Soc. Rev., 2015, 44, 677–698 RSC.
- W. Wu, Z. Lin and H. Jiang, Org. Biomol. Chem., 2018, 16, 7315–7329 RSC.
- H. E. Simmons and R. D. Smith, J. Am. Chem. Soc., 1958, 80, 5323–5324 CrossRef CAS.
- H. E. Simmons and R. D. Smith, J. Am. Chem. Soc., 1959, 81, 4256–4264 CrossRef CAS.
- S. E. Denmark and S. P. O'Connor, J. Org. Chem., 1997, 62, 3390–3401 CrossRef CAS.
- J. Long, Y. Yuan and Y. Shi, J. Am. Chem. Soc., 2003, 125, 13623–13633 Search PubMed.
- L. E. Zimmer and A. B. Charette, J. Am. Chem. Soc., 2009, 131, 15624–15626 CrossRef CAS.
- L.-P. B. Beaulieu, L. E. Zimmer, A. Gagnon and A. B. Charette, Chem.–Eur. J., 2012, 18, 14784–14791 CrossRef CAS.
- L.-P. B. Beaulieu, J. F. Schneider and A. B. Charette, J. Am. Chem. Soc., 2013, 135, 7819–7822 CrossRef CAS.
- S. Taillemaud, N. Diercxsens, A. Gagnon and A. B. Charette, Angew. Chem., Int. Ed., 2015, 54, 14108–14112 CrossRef CAS.
- G. Benoit and A. B. Charette, J. Am. Chem. Soc., 2017, 139, 1364–1367 CrossRef CAS.
- J. Furukawa, N. Kawabata and J. Nishimura, Tetrahedron, 1968, 24, 53–58 CrossRef CAS.
- J. Nishimura, J. Furukawa, N. Kawabata and M. Kitayama, Tetrahedron, 1970, 27, 1799–1806 CrossRef.
- Z. Yang, J. C. Lorenz and Y. Shi, Tetrahedron Lett., 1998, 39, 8621–8624 CrossRef CAS.
- Selected examples of metallacyclopropane:
(a) O. J. Curnow, R. P. Hughes, E. N. Mairs and A. L. Rheingold, J. Am. Chem. Soc., 1991, 114, 33153–33155 Search PubMed;
(b) B. R. Bender, J. R. Norton, M. M. Miller, O. P. Anderson and A. K. Rappé, Organometallics, 1992, 11, 3427–3434 CrossRef CAS;
(c) O. J. Curnow, R. P. Hughes, E. N. Mairs and A. L. Rheingold, Organometallics, 1993, 12, 3102–3108 CrossRef CAS;
(d) E. Mizushima, N. Chatani and F. Kakiuchi, J. Organomet. Chem., 2006, 691, 5739–5745 CrossRef CAS.
- Selected examples of metallacyclopropene:
(a) M. L. Buil, O. Eisenstein, M. A. Esteruelas, C. García-Yebra, E. Gutiérrez-Puebla, M. Oliván, E. Oñate, N. Ruiz and M. A. Tajada, Organometallics, 1999, 18, 4949–4959 CrossRef CAS;
(b) C. Cui, S. Köpke, R. Herbst-Irmer, H. W. Roesky, M. Noltemeyer, H.-G. Schmidt and B. Wrackmeyer, J. Am. Chem. Soc., 2001, 123, 9091–9098 CrossRef CAS;
(c) P. Barrio, M. A. Esteruelas and E. Oñate, Organometallics, 2003, 22, 2472–2485 CrossRef CAS;
(d) K. C. Stone, G. M. Jamison, P. S. White and J. L. Templeton, Organometallics, 2003, 22, 3093–3095 CrossRef;
(e) J. Chen, C. Shi, H. H.-Y. Sung, I. D. Williams, Z. Lin and G. Jia, Organometallics, 2012, 31, 1817–1824 CrossRef CAS;
(f) D. R. Williams, A. A. Shah, S. Mazumder and M.-H. Baik, Chem. Sci., 2013, 4, 238–247 RSC.
- Selected examples of ring-opening reactions of strained three-membered metallacycles:
(a) H. Yamazaki, K. Aoki, Y. Yamamoto and Y. Wakatsuki, J. Am. Chem. Soc., 1975, 97, 3546–3548 CrossRef CAS;
(b) S. L. Buchwald, R. T. Lum and J. C. Dewan, J. Am. Chem. Soc., 1986, 108, 7441–7442 CrossRef CAS;
(c) S. L. Buchwald and B. T. Watson, J. Am. Chem. Soc., 1986, 108, 7411–7413 CrossRef CAS;
(d) G. A. Vaughan, C. D. Sofield and G. L. Hillhouse, J. Am. Chem. Soc., 1989, 111, 5491–5493 CrossRef CAS;
(e) J. K. F. Buijink, K. R. Kloetstra, A. Meetsma and J. H. Teuben, Organometallics, 1996, 15, 2523–2533 CrossRef CAS;
(f) S. Mansel, D. Thomas, C. Lefeber, D. Heller, R. Kempe, W. Baumann and U. Rosenthal, Organometallics, 1997, 16, 2886–2890 CrossRef CAS;
(g) L. W. M. Lee, W. E. Piers, M. Parvez, S. J. Rettig and V. G. Young, Organometallics, 1999, 18, 3904–3912 CrossRef CAS;
(h) K. Tsuchiya, H. Kondo and H. Nagashima, Organometallics, 2007, 26, 1044–1051 CrossRef CAS;
(i) T. Beweries, C. Fischer, S. Peitz, V. V. Burlakov, P. Arndt, W. Baumann, A. Spannenberg, D. Heller and U. Rosenthal, J. Am. Chem. Soc., 2009, 131, 4463–4469 CrossRef CAS;
(j) S. Zhang, W.-X. Zhang, J. Zhao and Z. Xi, J. Am. Chem. Soc., 2010, 132, 14042–14045 CrossRef CAS;
(k) Z. J. Lv, Z. Huang, J. Shen, W. X. Zhang and Z. Xi, J. Am. Chem. Soc., 2019, 141, 20547–20555 CrossRef CAS.
- Selected examples of conversion of metallacyclopropenes:
(a) S. L. Buchwald, E. A. Lucas and J. C. Dewan, J. Am. Chem. Soc., 1987, 109, 4396–4397 CrossRef CAS;
(b) J. F. Hartwig, R. A. Andersen and R. G. Bergman, J. Am. Chem. Soc., 1988, 111, 2717–2719 CrossRef;
(c) S. L. Buchwald, E. A. Lucas and W. M. Davis, J. Am. Chem. Soc., 1989, 111, 397–398 CrossRef CAS;
(d) M. A. Bennett and E. Wenger, Organometallics, 1995, 14, 1267–1277 CrossRef CAS;
(e) S. Saito, K. Tando, C. Kabuto and Y. Yamamoto, Organometallics, 2000, 19, 3740–3743 CrossRef CAS;
(f) K. R. Deaton and M. S. Gin, Org. Lett., 2003, 5, 2477–2480 CrossRef CAS;
(g) J. Zhang, S. Xing, J. Ren, S. Jiang and Z. Wang, Org. Lett., 2015, 17, 218–221 CrossRef CAS;
(h) Y. Sumida, T. Sumida, D. Hashizume and T. Hosoya, Org. Lett., 2016, 18, 5600–5603 CrossRef CAS;
(i) G. R. Kiel, S. C. Patel, P. W. Smith, D. S. Levine and T. D. Tilley, J. Am. Chem. Soc., 2017, 139, 18456–18459 CrossRef CAS;
(j) G. R. Kiel, M. S. Ziegler and T. D. Tilley, Angew. Chem., Int. Ed., 2017, 56, 4839–4844 CrossRef CAS;
(k) J. M. Kieser, R. J. Gilliard Jr, A. L. Rheingold, H. Grutzmacher and J. D. Protasiewicz, Chem. Commun., 2017, 53, 5110–5112 RSC;
(l) G. R. Kiel, A. E. Samkian, A. Nicolay, R. J. Witzke and T. D. Tilley, J. Am. Chem. Soc., 2018, 140, 2450–2454 CrossRef CAS;
(m) M. Terada, Y. Nishii and M. Miura, Chem. Commun., 2018, 54, 2918–2921 RSC.
- M. Luo, C. Zhu, L. Chen, H. Zhang and H. Xia, Chem. Sci., 2016, 7, 1815–1818 RSC.
- M. Luo, L. Long, H. Zhang, Y. Yang, Y. Hua, G. Liu, Z. Lin and H. Xia, J. Am. Chem. Soc., 2017, 139, 1822–1825 CrossRef CAS.
- M. Luo, Y. Hua, K. Zhuo, L. Long, X. Lin, Z. Deng, Z. Lin, H. Zhang, D. Chen and H. Xia, CCS Chem., 2020, 2, 758–763 CrossRef.
- P. J. Stephens and F. J. Devlin, J. Phys. Chem., 1994, 98, 11623–11627 CrossRef CAS.
- C. Peng, P. Y. Ayala and H. B. Schlegel, J. Comput. Chem., 1996, 17, 49–56 CrossRef CAS.
- J.-D. Chai and M. Head-Gordon, Phys. Chem. Chem. Phys., 2008, 10, 6615–6620 RSC.
- F. Weigend and R. Ahlrichs, Phys. Chem. Chem. Phys., 2005, 7, 3297–3305 RSC.
- A. V. Marenich, C. J. Cramer and D. G. Truhlar, J. Phys. Chem. B, 2009, 113, 6378–6396 CrossRef CAS.
-
E. D. Glendening, J. K. Badenhoop, A. E. Reed, J. E. Carpenter, J. A. Bohmann, C. M. Morales, C. R. Landis, and F. Weinhold. NBO 6.0, Theoretical Chemistry Institute, University of Wisconsin, Madison, WI, 2013 Search PubMed.
- P. v. R. Schleyer, C. Maerker, A. Dransfeld, H. Jiao and N. J. R. v. E. Hommes, J. Am. Chem. Soc., 1996, 118, 6317–6318 CrossRef CAS.
- Z. Chen, C. S. Wannere, C. Corminboeuf, R. Puchta and P. v. R. Schleyer, Chem. Rev., 2005, 105, 3842–3888 CrossRef CAS.
- H. Fallah-Bagher-Shaidaei, C. S. Wannere, C. Corminboeuf, R. Puchta and P. v. R. Schleyer, Org. Lett., 2006, 8, 863–866 CrossRef CAS.
-
M. J. Frisch, et al., Gaussian 09, revision E.01, Gaussian, Inc., Wallingford CT, 2013 Search PubMed.
- R. Herges and D. Geuenich, J. Phys. Chem. A, 2001, 105, 3214–3220 CrossRef CAS.
- D. Geuenich, K. Hess, F. Köhler and R. Herges, Chem. Rev., 2005, 105, 3758–3772 CrossRef CAS.
- F. Bernardi, A. Bottoni and G. P. Miscione, J. Am. Chem. Soc., 1997, 119, 12300–12305 CrossRef CAS.
- A. Hirai, M. Nakamura and E. Nakamura, Chem. Lett., 1998, 27, 927–928 CrossRef.
- W.-H. Fang, D. L. Phillips, D. Wang and Y.-L. Li, J. Org. Chem., 2002, 67, 154–160 CrossRef CAS.
- D. Wang, D. L. Phillips and W.-H. Fang, Organometallics, 2002, 21, 5901–5910 CrossRef CAS.
- C. Zhao, D. Wang and D. L. Phillips, J. Am. Chem. Soc., 2002, 124, 12903–12914 CrossRef CAS.
- M. Nakamura, A. Hirai and E. Nakamura, J. Am. Chem. Soc., 2003, 125, 2341–2350 CrossRef CAS.
- T. Wang, Y. Liang and Z.-X. Yu, J. Am. Chem. Soc., 2011, 133, 9343–9353 CrossRef CAS.
- C. Zhu, S. Li, Z. Cao, X. Lu, T. Wen, M. Luo, X. Zhou, Z. Xie, Y. Niu, M. Lin, P. v. R. Schleyer, J. Zhu and H. Xia, Nat. Chem., 2013, 5, 698–703 CrossRef CAS.
- C. Zhu, Y. Yang, M. Luo, C. Yang, J. Wu, L. Chen, G. Liu, T. Wen, J. Zhu and H. Xia, Angew. Chem., Int. Ed., 2015, 127, 6279–6283 CrossRef.
- Q. Zhuo, J. Lin, Y. Hua, X. Zhou, Y. Shao, S. Chen, Z. Chen, J. Zhu, H. Zhang and H. Xia, Nat. Commun., 2017, 8, 1912–1918 CrossRef.
- Z. Chu, G. He, X. Cheng, Z. Deng and J. Chen, Angew. Chem., Int. Ed., 2019, 58, 9174–9178 CrossRef CAS.
- H. Xia, G. He, H. Zhang, T. B. Wen, H. H. Y. Sung, I. D. Williams and G. Jia, J. Am. Chem. Soc., 2004, 126, 6862–6863 CrossRef CAS.
- S. H. Liu, W. S. Ng, H. S. Chu, T. B. Wen, H. Xia, Z. Y. Zhou, C. P. Lau and G. Jia, Angew. Chem., Int. Ed., 2002, 41, 1589–1591 CrossRef CAS.
- Q. Zhuo, H. Zhang, Y. Hua, H. Kang, X. Zhou, X. Lin, Z. Chen, J. Lin, K. Zhuo and H. Xia, Sci. Adv., 2018, 4, eaat0336 CrossRef.
- M. Batuecas, R. Castro-Rodrigo, M. A. Esteruelas, C. García-Yebra, A. M. López and E. Oñate, Angew. Chem., Int. Ed., 2016, 55, 13749–13753 CrossRef CAS.
Footnote |
† Electronic supplementary information (ESI) available: Characterization details of new compounds, computational details, crystallographic details, NMR spectra. CCDC 2005482 (1d), 2005483 (2a), 2005484 (2b), 2005479 (3a), 2005480 (3b), and 2005481 (6). For ESI and crystallographic data in CIF or other electronic format see DOI: 10.1039/d0sc03215h |
|
This journal is © The Royal Society of Chemistry 2020 |
Click here to see how this site uses Cookies. View our privacy policy here.