DOI:
10.1039/D0SC02745F
(Edge Article)
Chem. Sci., 2020,
11, 6325-6331
Reversible photo-control over transmembrane anion transport using visible-light responsive supramolecular carriers†
Received
13th May 2020
, Accepted 4th June 2020
First published on 4th June 2020
Abstract
Ion transport across lipid bilayer membranes in biology is controlled by membrane proteins, which in turn are regulated in response to chemical-, physical- and photo-stimuli. The design of synthetic supramolecular ion transporters able to be precisely controlled by external signals, in particular bio-compatible wavelengths of visible light, is key for achieving spatio-temporal control over function. Here we report two-colour responsive molecular photo-switches that act as supramolecular transmembrane anion carriers. Reversible switching of the photo-switch within the lipid bilayer membrane is achieved using biocompatible visible wavelengths of light, such that temporal control over transmembrane anion transport is achieved through alternating irradiation with red and blue light.
Introduction
Ion transport across lipid bilayer membranes in biology is regulated by membrane proteins, which are themselves regulated by external stimuli such as small molecules, membrane potential and light. Protein ion channels and their modified analogues find applications in diverse fields ranging from optogenetics,1 photopharmacology,2 and synthetic biology.3 Supramolecular anionophores that act as mobile ion carriers have also attracted significant interest; driven by potential uses as therapeutics for cancer treatment and diseases arising from mis-regulated ion channels including cystic fibrosis, or as tools for studying ion transport processes.4 Significant effort has been devoted to designing mobile carrier systems with high anion transport activity in vesicles and, more recently, in cells.5
Whilst the activity of natural ion channels and transporters can be precisely controlled by external stimuli, stimuli-responsive supramolecular ion channels6 and mobile ion carriers7 are rare, and achieving high levels of control (to switch between inactive “OFF” states and ion transporting “ON” states) is particularly challenging. Photo-regulated transporters are particularly attractive targets, because of the possibility of achieving both spatial and temporal control over ion transport in a readily adaptable synthetic system. Since the pioneering work of Shinkai and co-workers on photo-controlled cation transport in liquid membranes,8 the large amplitude conformational change afforded by E–Z photo-isomerisation has been a key component of many light-activated molecular machines, receptors and catalysts.9 However, light-activated mobile ion carriers that function in lipid bilayers are extremely rare, and precise, reversible control over activity is particularly challenging to achieve. Furthermore, the photo-responsive ion carriers reported to date employ almost exclusively UV light-triggered transport mechanisms.7e–g UV-light is generally unsuitable for biological applications due to poor tissue penetration and collateral cell damage.2a Because of this limitation, long wavelength visible light photo-switches are attracting significant interest as tools for chemical biology applications.10 Here we report a supramolecular photo-switchable carrier able to regulate transmembrane anion transport using visible light. The system is reversibly controlled by alternate irradiation with red and blue light, with a high level of discrimination between the OFF and ON states.
Results and discussion
Approach
Synthetic mobile ion carriers facilitate ion transport down a concentration gradient by lowering the kinetic barrier to transmembrane transport. This is achieved through binding of the ion within a hydrophobic receptor, which diffuses across the bilayer to release the ion on the opposite side. For the development of reversible photo-controlled anion carriers, we identified two key requirements: (i) excellent discrimination between the inactive “OFF” state (with negligible background transport) and photo-activated “ON” state and (ii) photo-switching with visible light for improved biocompatibility. Efficient anion transporters require sufficient lipophilicity to ensure optimum partitioning into the membrane, excellent anion binding affinity,11 and also benefit from encapsulation of the hydrophilic anion within a hydrophobic receptor.12 Conversely, carriers with weak anion binding affinity, poor encapsulation and extended conformations typically exhibit low activity; the latter attributed to reduced mobility and high reorganization penalties within the membrane.13
We identified red-shifted azobenzenes bearing anion binding groups of the general structure 1 (Fig. 1) as a possible scaffold that fulfils these requirements, where E–Z photo-isomerization of azobenzenes is exploited to enhance anion recognition by cooperative binding between two appended receptors. A greater degree of anion encapsulation and increased mobility of the less extended Z isomer relative to the E isomer enhances the anion transport capability further.
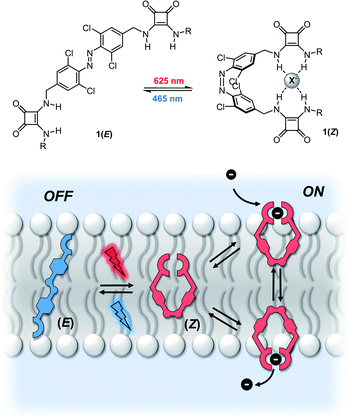 |
| Fig. 1 Schematic representation of two-colour photo-switching of a supramolecular ion carrier 1. | |
Design and synthesis
The E–Z photo-isomerisation of azobenzenes is achieved using UV light and reversed with visible light or by thermal relaxation; the rate of which depends on the substituents but can often be fast (t1/2 milliseconds to minutes).14 To address the key challenge of achieving reversible, visible light mediated control over ion transport, we designed molecular photo-switches incorporating ortho-chloro-substituted azobenzene derivatives. Recent work by a number of groups including those of Hecht and Woolley has demonstrated that heteroatom ortho-substitution of azobenzenes leads to significant red shifting of the n–π* band of the E isomer, separating it from the Z n–π* transition.15 This allows for bi-directional photo-switching with orthogonal wavelengths of visible light, and significantly enhanced Z isomer lifetimes. Remarkably, ortho-chloro-derivatives have been shown to allow photo-switching with red light, which is highly beneficial for biological applications.15d
Direct conjugation of anion binding motifs to the azobenzene core is known to significantly decrease the lifetime of the Z isomer (such as in azobenzene-urea derivatives).16 Molecular mechanics calculations indicated that incorporation of methylene spacers between the photo-switch and the anion binding squaramides allows for cooperative chloride anion binding between both squaramide motifs in the Z isomer (Fig. 2). Squaramides are potent halide ion binders, and highly effective anion transporters.17
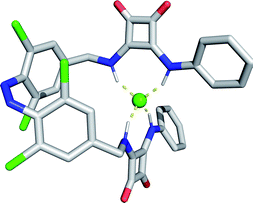 |
| Fig. 2 Calculated structure of the chloride complex 1aZ·Cl (MM+ force field, HyperChem). | |
Red-shifted azobenzene derivatives 1a–i were prepared as shown in Scheme 1. Reduction and immediate protection of 4-cyano-2,6-dichloroaniline 2 afforded 3, which was subjected to oxidative coupling conditions18 to afford red-shifted azobenzene 4 in good yields. Quantitative Boc-deprotection gave rise to 5, which was subsequently reacted with mono-squaramide derivatives (6a–i, prepared from dimethyl squarate and the corresponding amine derivative), to afford the anion carriers 1a–i. For full experimental procedures and characterization see the ESI.†
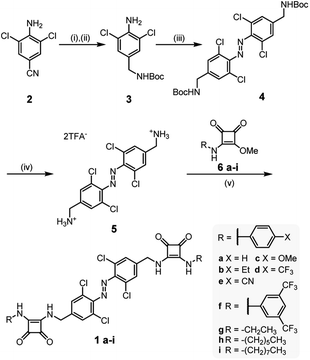 |
| Scheme 1 Synthesis of squaramide-functionalized azobenzene anion carriers. Conditions: (i) LiAlH4, THF, 3 h, rt; (ii) Boc2O, THF, 3 h, rt, 59% over two steps; (iii) NCS, DBU, CH2Cl2, 10 min, −78 °C, 43%; (iv) TFA, CH2Cl2, 4 h, quant.; (v) 6a–i; DIPEA, MeOH, 16 h, 55 °C, 49–83%. | |
We first examined the photo-switching properties of the parent azobenzene 4. Irradiation of 4E with red light from an LED (625 nm, ∼0.9 W) allowed for high conversion to the Z isomer in the photo-stationary state (PSS) (80% 4Z as determined by NMR integration). The UV-vis spectra of 4E and 4Z are shown in Fig. 3. As with similar ortho-chloro-substituted azobenzenes,15d significant red-shifting of the n–π* transition is observed, and separation of the E n–π* from the Z n–π* transitions allows for efficient switching (Fig. 2). Irradiation with blue light (LED, 455 nm, ∼1.1 W) was effective at triggering the reverse Z → E photo-isomerisation (86% Z → E). The Z isomer exhibited excellent thermal stability, with a half-life for the thermally promoted Z → E isomerization of ∼7 days at 298 K (Fig. S78†).
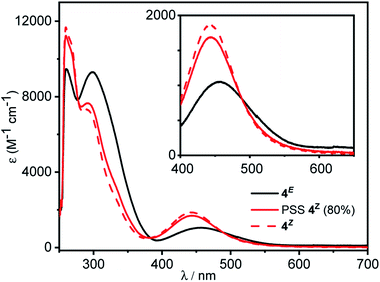 |
| Fig. 3 Absorption spectra of 4 as the E and Z isomer, as well as the PSS mixture in DMSO at 25 °C. Inset: n → π* bands and composition of the PSS mixtures upon irradiation with red light (625 nm, determined by NMR integration). Pure Z-isomer spectrum was calculated from the spectra of pure 4E and the PSS mixture of known composition. | |
NMR anion binding titrations
With the library of squaramide-functionalized bistable switches in hand, we examined their chloride binding capability by conducting 1H NMR titration experiments in d6-DMSO by addition of aliquots of tetrabutylammonium chloride, and monitoring the binding induced chemical shift perturbations. Data were fitted to the appropriate binding isotherms using the Dynafit software package,19 and the association constants are shown in Table 1. KE1 is the 1
:
1 binding constant for chloride recognition by one squaramide motif of the bi-functional E-isomer, weighted by the appropriate statistical factor, and KZ1 is the corresponding 1
:
1 binding constant for chloride recognition by the Z isomer. The latter were determined by repeating the titration with the PSS mixture formed by irradiating the sample in the NMR tube with 625 nm light (77% Z for 1a–i), and accounting for the binding competition to the minor population of E isomer in the analysis (see ESI† for data and binding models). The affinity of 1E for chloride was relatively weak in this competitive polar solvent, and binding of a second equivalent of anion was too weak to be determined, presumably due to unfavourable anion–anion repulsion. The affinity of 1Z derivatives for chloride were higher than the analogous 1E isomers, with a ratio of KZ1/KE1 ∼ 3, indicating cooperative anion binding by both squaramide motifs in the Z isomer, consistent with the calculated structure. Varying the electron donating or withdrawing nature of the squaramide translated into changes in the binding affinity for chloride in both E and Z isomers, and for the aryl substituents this showed good correlation with Hammett constant20 (Fig. 4).
Table 1 Characteristics of photo-switchable anion carriers 1
Entry |
Characteristic |
Carriers |
1a
|
1b
|
1c
|
1d
|
1e
|
1f
|
1g
|
1h
|
1I
|
1 : 1 association constant for chloride binding in d6-DMSO. KE1 is statistically corrected to account for affinity to one squaramide, where KE1 = Kobs/2. Errors at the 95% confidence limit.
Effective concentration to reach 50% of maximal activity in the HPTS assay, in LUVs of POPC (mean diameter 200 nm, lipid concentration 31 μM) loaded with 1 mM HPTS, NaCl (100 mM) and buffered at pH 7.0 with 10 mM HEPES. For compounds of low activity, estimated lower bounds for the EC50 value are given.
For the Z isomer, ECZ,PSS50 indicates apparent value achieved by addition of the 77 : 23 Z/E PSS mixture.
Hill coefficient.
Not determined.
Poor solubility in DMSO prevented full Hill analysis.
|
1 |
K
E
1 (M−1)a |
90 ± 2 |
72 ± 3 |
77 ± 3 |
126 ± 1 |
137 ± 10 |
139 ± 10 |
47 ± 1 |
65 ± 5 |
−e |
2 |
K
Z
1 (M−1)a |
267 ± 3 |
201 ± 3 |
231 ± 12 |
346 ± 2 |
358 ± 12 |
402 ± 6 |
142 ± 7 |
204 ± 21 |
−e |
3 |
ECE50 (nM)b |
181 ± 16 |
591 ± 341 |
1020 ± 477 |
146 ± 40 |
232 ± 143 |
246 ± 121 |
>5000f |
204 ± 42 |
>20 000f |
4 |
ECZ,PSS50 (nM)b,c |
22 ± 2 |
101 ± 18 |
218 ± 54 |
42 ± 12 |
64 ± 13 |
90 ± 55 |
432 ± 41 |
28 ± 2 |
>20 000f |
5 |
n
E
|
2.6 ± 0.5 |
0.6 ± 0.1 |
0.6 ± 0.1 |
1.0 ± 0.2 |
0.6 ± 0.1 |
0.6 ± 0.1 |
—e |
0.8 ± 0.1 |
−e |
6 |
n
Z
|
1.1 ± 0.1 |
1.0 ± 0.2 |
0.6 ± 0.1 |
0.8 ± 0.1 |
0.7 ± 0.1 |
0.7 ± 0.1 |
1.4 ± 0.1 |
1.2 ± 0.1 |
−e |
7 |
K
Z
1/KE1 |
3.1 |
2.8 |
3.0 |
2.7 |
2.6 |
2.9 |
3.0 |
3.1 |
−e |
8 |
ECZ,PSS50/ECE50 |
8.2 |
5.9 |
4.7 |
3.5 |
3.6 |
2.7 |
>10f |
7.3 |
−e |
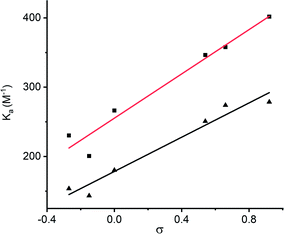 |
| Fig. 4 Plot of K vs. Hammett parameter σ for aryl derivatives of 1E and 1Z. Values taken from ref. 20. | |
Anion transport studies
The anion transport activities of the derivatives of 1 were determined in 1-palmitoyl-2-oleoyl-sn-glycero-3-phosphocholine large unilamellar vesicles (POPC LUVs, lipid concentration 31 μM), loaded with 8-hydroxypyrene-1,3,6-trisulfonate (HPTS) buffered to pH 7.0 in NaCl solution. A pH gradient was applied across the membrane by addition of a base pulse, followed by addition of the carrier as a DMSO solution (<0.5% v/v). The ability of the anionophore to dissipate the pH gradient by transmembrane ion transport was determined by recording the change in the HPTS emission, Irel (λem = 510 nm), with time following excitation at λex = 405/465 nm (Fig. 5A). Detergent (Triton X-100) was added at the end of each experiment to lyse the vesicles for calibration. For the Z isomers, the experiment was run using the 77
:
23 Z/E PSS mixture formed by irradiating the sample with 625 nm light.
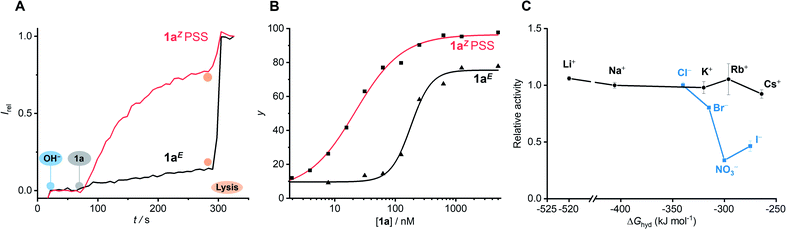 |
| Fig. 5 (A) Change in ratiometric emission (λem = 510 nm; λex1 = 405 nm, λex2 = 460 nm) upon addition of 62.5 nM 1aE (black) and 1aZ (red) in DMSO to POPC LUVs (31 μM) containing 1 mM HPTS, 100 mM internal and external NaCl, buffered with 10 mM HEPES at pH 7.0. A pH gradient is generated by addition of a NaOH base pulse (5 mM), followed by 1a in DMSO. Lysis by Triton X-100 calibrates the assay. (B) Dependence on Irel immediately prior to lysis on concentration of 1aE (▲) and 1aZ (■), and fit to the Hill equation (solid line). (C) Dependence of the activity of 1aZ (62.5 nM) on extra-vesicular ions (100 mM MCl or NaX; inside 100 mM NaCl). Error bars represent standard deviations. Original data for all other compounds is available in the ESI.† | |
The concentration dependence of the activity of the E and Z isomers of each anion carrier was determined by adding increasing concentrations of the transporter in DMSO. The fractional activities (relative intensity just prior to lysis) were plotted as a function of concentration (Fig. 5B) and the dose–response curves fitted to the Hill equation. This is used to describe the dependence of the anion transport activity (reported by Irel) on the n-th power of the carrier concentration, and facilitates comparison of the relative activities of carriers through an effective concentration value (EC50) required to reach 50% activity.
Quantifying anion transport activity
The EC50 and n values for the analogues of 1E, and for the 1Z enriched PSS (Z
:
E 77
:
23) are given in Table 1, entries 3–6; and the corresponding ECZ,PSS50/ECE50 ratios (entry 8). A significant enhancement of activity, up to a maximum of 8-fold for compound 1a, was observed for the Z isomers.21 In general, squaramides with electron withdrawing aryl substituents resulted in efficient transport. The optimal chain length of alkyl derivatives was six carbons, with both ethyl and octyl chains proving detrimental to transport efficiency. The difference in activity between both photo-isomers could be estimated by the ratio ECZ,PSS50/ECE50, which was found to be generally higher for the less active compounds, presumably arising from a decreased ability of the E isomer to bind and transport anions. In contrast, the ratio of 1
:
1 binding constants, KZ1/KE1, remains consistent at ∼3 across the whole family of compounds. We hypothesise that the larger increase in transporter efficiency for the 1Z derivatives, compared to that predicted by the ratio of binding constants alone, arises in part from reduced mobility of the linear E isomer–anion complex in the membrane, and diminished ability to fully encapsulate the anion compared to the Z isomer. It has been previously shown that the relationship between anion binding affinity and EC50 is complex, often without direct correlation, because transport efficiency is also strongly dependent on other factors including molecular size and hydrophobicity.11b The important role of encapsulation in increasing transport affinity has also previously been demonstrated.12
Hill coefficients of ∼1 for 1Z analogues suggests ion complexation with a likely 1
:
1 stoichiometry (1Z·Cl−) in the transport complex, consistent with results from titration experiments and calculation. Enhanced binding interactions in membranes, arising from two-dimensional confinement and the hydrophobic, low polarity environment,22 may also allow for competing formation of the E isomer 2
:
1 transporter–anion complex (1E2·Cl−), as well as hydrogen bonding aggregates of 1E in the membrane. Hill coefficients ranging between ∼0.6–2.6 are indicative of a range of competing equilibria in the membrane for the 1E isomer derivatives, with n < 1 arising from competing formation of inactive complexes and/or precipitation at high concentrations.23 Compound 1a provides a balance between good ECZ50/ECE50 discrimination and overall high activity as the Z isomer (EC50 of 22 nM, 0.07 mol% relative to the lipids), and was used for analysis in subsequent experiments.
Ion selectivity and mechanistic studies
The activity of transporter 1aZ was unaffected by iso-osmolar replacement24 of the external Na+ cation with Li+, K+, Rb+ or Cs+, but was sensitive to replacement of the external Cl− anion with Br−, I− or NO3− (Fig. 5C). No observable transport was detected when chloride was replaced with gluconate, a large hydrophilic anion,12 suggesting that 1aZ is unable to overcome the large dehydration penalty required for OH−/gluconate− antiport (Fig. S138†). Diminished activity with anionic lipids (egg yolk phosphatidylglycerol) was also consistent with the requirement for formation of an anionic complex 1Z·Cl−, due to charge repulsion with the membrane (Fig. S139†). Together, these results demonstrate that the dissipation of the pH gradient reported in the HPTS assay must arise from transmembrane anion transport (OH−/Cl− antiport, or H+/Cl− symport), and not from H+/M+ cation antiport, in agreement with the mode of action of previously reported squaramide anion carriers.17
Anion transport by 1aE and 1aZ in the lipid gel phase of dipalmitoylphosphatidylcholine (DPPC) lipids at 25 °C was inhibited and restored at 45 °C, above the gel–liquid phase transition temperature (Tc = 41 °C) (Fig. 6A). This behaviour is consistent with the proposed mobile carrier mechanism, in which mobility is significantly reduced in the gel phase, and rules out alternative formation of a membrane spanning channel structure whose activity would be independent of the lipid phase. Inactivity in the calcein release assays further rules out carrier-induced non-specific leakage (Fig. 6B).
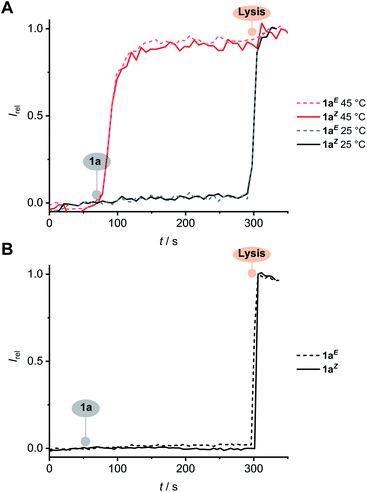 |
| Fig. 6 (A) HPTS assay with DPPC LUVs: 200 nM 1aE (dashed lines) and 62.5 nM 1aZ (solid lines) at 25 °C (black data) and 45 °C (red data). (B) Calcein release assay with 5 μM 1aE (dashed line) and 1aZ (solid line) in POPC LUVs. | |
Reversible photo-switching of anion transport
To investigate in situ, reversible photo-switching of ion transport in vesicles, we measured the transporter-induced chloride efflux from POPC vesicles containing buffered NaCl, suspended in NaNO3 in real time using a chloride ion selective electrode (ISE). Sample illumination was achieved using high powered LEDs (see ESI† for details). Initial studies confirmed that the system could facilitate Cl−/NO3− antiport in this assay, following external addition of the 1aZ-rich PSS, with minimal background transport by 1aE in this assay (Fig. S145†).
Reversible control over ion transport by in situ photo-switching of 1a in the vesicle membrane was achieved by adding 1aE as a DMSO solution (0.5 mol% relative to lipid) to the gently stirred vesicle suspension. Irradiation with red light (625 nm) was used to photo-isomerise the transporter within the lipid bilayer membrane, and turn-on the Cl−/NO3− transmembrane transport process, which could be turned off by subsequent irradiation with blue light (455 nm) (Fig. 7). The rate of ion transport following in situ photo-isomerisation in the vesicles was ∼50% of that achieved by direct addition of 1aZ,PSS.25
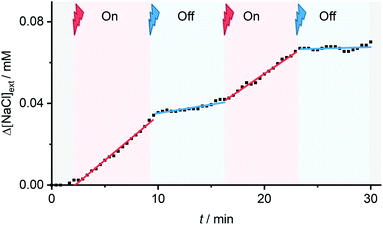 |
| Fig. 7 Photo-triggered ion transport by 1a (0.5 mol% relative to lipid) from POPC vesicles containing 489 mM NaCl, suspended in 489 mM NaNO3, and buffered with phosphate salts at pH 7.0. Chloride efflux from POPC vesicles monitored by a chloride-selective ISE. Colours indicate commencement of sample irradiation using 625 nM (0.9 W, red) or 455 nm (1.1 W, blue) LEDs. Black squares: vesicle exterior Cl− concentration; lines: linear fit. | |
Alternating between 625 and 455 nm light allowed for reversible switching between the transporting “ON” and inactive states “OFF” states, directly coupling photo-isomerisation with ion transport. Inactivity under alternating red and blue light irradiation in the absence of 1a; and the lack of switching behaviour when 1a was replaced with a simple non-photo-responsive squaramide derivative (ESI†), confirmed the role of photo-switch 1a in the photo-switchable transport behaviour (Fig. S147†).
Conclusions
We have demonstrated reversible, visible light control over transmembrane anion transport using a synthetic photo-switchable anion carrier. Fluorescence anion transport assays in combination with ISE experiments demonstrate the ability of the squaramide-substituted azobenzenes to act as mobile anion carriers. Switching between the less active E isomer and more active Z isomer can be achieved with red light, whilst back-switching takes place using blue light; allowing for in situ, temporal control over transmembrane ion transport in lipid bilayer vesicles. We anticipate that these results will provide the foundation for developing sophisticated photo-controlled membrane transport systems, with potential longer term impact in targeted therapeutics and synthetic biology.
Conflicts of interest
There are no conflicts to declare.
Acknowledgements
A. K. thanks the EPSRC Centre for Doctoral Training in Synthesis for Biology and Medicine for a studentship (EP/L015838/1), generously supported by AstraZeneca, Diamond Light Source, Defence Science and Technology Laboratory, Evotec, GlaxoSmithKline, Janssen, Novartis, Pfizer, Syngenta, Takeda, UCB and Vertex. MJL acknowledges funding from the Royal Society, the John Fell Oxford University Press Research Fund, the University of Oxford's EPSRC Capital Award in Support of Early Career Researchers (EP/S017658/1), and SCG Chemicals Co. Ltd. for funding through the SCG-Oxford Centre of Excellence in Chemistry: SCG Innovation Fund. M. J. L. is a Royal Society University Research Fellow.
Notes and references
- G. Miesenböck, Annu. Rev. Cell Dev. Biol., 2011, 27, 731–758 CrossRef PubMed.
-
(a) W. A. Velema, W. Szymanski and B. L. Feringa, J. Am. Chem. Soc., 2014, 136, 2178–2191 CrossRef CAS PubMed;
(b) K. Hüll, J. Morstein and D. Trauner, Chem. Rev., 2018, 118, 10710–10747 CrossRef PubMed.
-
(a) G. Villar, A. D. Graham and H. Bayley, Science, 2013, 340, 48–52 CrossRef CAS PubMed;
(b) Y. Elani, R. V. Law and O. Ces, Ther. Delivery, 2015, 6, 541–543 CrossRef CAS PubMed;
(c) K. P. Adamala, D. A. Martin-Alarcon, K. R. Guthrie-Honea and E. S. Boyden, Nat. Chem., 2017, 9, 431–439 CrossRef CAS PubMed.
- For recent reviews see:
(a) J. T. Davis, O. Okunola and R. Quesada, Chem. Soc. Rev., 2010, 39, 3843–3862 RSC;
(b) S. Matile, A. V. Jentzsch, J. Montenegro and A. Fin, Chem. Soc. Rev., 2011, 40, 2453–2474 RSC;
(c) P. A. Gale, J. T. Davis and R. Quesada, Chem. Soc. Rev., 2017, 46, 2497–2519 RSC.
-
(a) B. Díaz de Greñu, P. I. Hernández, M. Espona, D. Quiñonero, M. E. Light, T. Torroba, R. Pérez-Tomás and R. Quesada, Chem.–Eur. J., 2011, 17, 14074–14083 CrossRef PubMed;
(b) S.-K. Ko, S. K. Kim, A. Share, V. M. Lynch, J. Park, W. Namkung, W. Van Rossom, N. Busschaert, P. A. Gale and J. L. Sessler,
et al.
, Nat. Chem., 2014, 6, 885–892 CrossRef CAS PubMed;
(c) V. Soto-Cerrato, P. Manuel-Manresa, E. Hernando, S. Calabuig-Fariñas, A. Martínez-Romero, V. Fernández-Dueñas, K. Sahlholm, T. Knöpfel, M. García-Valverde and A. M. Rodilla,
et al.
, J. Am. Chem. Soc., 2015, 137, 15892–15898 CrossRef CAS PubMed;
(d) H. Li, H. Valkenier, L. W. Judd, P. R. Brotherhood, S. Hussain, J. A. Cooper, O. Jurček, H. A. Sparkes, D. N. Sheppard and A. P. Davis, Nat. Chem., 2016, 8, 24–32 CrossRef CAS PubMed.
-
(a) J.-Y. Chen and J.-L. Hou, Org. Chem. Front., 2018, 5, 1728–1736 RSC;
(b) P. V. Jog and M. S. Gin, Org. Lett., 2008, 10, 3693–3696 CrossRef CAS PubMed;
(c) T. Liu, C. Bao, H. Wang, Y. Lin, H. Jia and L. Zhu, Chem. Commun., 2013, 49, 10311–10313 RSC;
(d) Y. Zhou, Y. Chen, P.-P. Zhu, W. Si, J.-L. Hou and Y. Liu, Chem. Commun., 2017, 53, 3681–3684 RSC;
(e) V. Gorteau, F. Perret, G. Bollot, J. Mareda, A. N. Lazar, A. W. Coleman, D.-H. Tran, N. Sakai and S. Matile, J. Am. Chem. Soc., 2004, 126, 13592–13593 CrossRef CAS PubMed;
(f) P. Talukdar, G. Bollot, J. Mareda, N. Sakai and S. Matile, Chem. - Eur. J., 2005, 11, 6525–6532 CrossRef CAS PubMed;
(g) C. P. Wilson and S. J. Webb, Chem. Commun., 2008, 34, 4007–4009 RSC;
(h) U. Devi, J. R. D. Brown, A. Almond and S. J. Webb, Langmuir, 2011, 27, 1448–1456 CrossRef CAS PubMed.
-
(a) I. M. Bennett, H. M. V. Farfano, F. Bogani, A. Primak, P. A. Liddell, L. Otero, L. Sereno, J. J. Silber, A. L. Moore and T. A. Moore,
et al.
, Nature, 2002, 420, 398–401 CrossRef CAS PubMed;
(b) N. Busschaert, R. B. P. Elmes, D. D. Czech, X. Wu, I. L. Kirby, E. M. Peck, K. D. Hendzel, S. K. Shaw, B. Chan and B. D. Smith,
et al.
, Chem. Sci., 2014, 5, 3617 RSC;
(c) Y. R. Choi, B. Lee, J. Park, W. Namkung and K.-S. Jeong, J. Am. Chem. Soc., 2016, 138, 15319–15322 CrossRef CAS PubMed;
(d) X. Wu, J. R. Small, A. Cataldo, A. M. Withecombe, P. Turner and P. A. Gale, Angew. Chem., Int. Ed., 2019, 58, 15142–15147 CrossRef CAS PubMed For a visible light responsive cation transporters see:
(e) T. Jin, Mater. Lett., 2007, 61, 805–808 CrossRef CAS;
(f) Y. Rin Choi, G. Chan Kim, H.-G. Jeon, J. Park, W. Namkung and K.-S. Jeong, Chem. Commun., 2014, 50, 15305–15308 RSC;
(g) S. B. Salunke, J. A. Malla and P. Talukdar, Angew. Chem., Int. Ed., 2019, 58, 5354–5358 CrossRef CAS PubMed.
- S. Shinkai, T. Nakaji, T. Ogawa, K. Shigematsu and O. Manabe, J. Am. Chem. Soc., 1981, 103, 111–115 CrossRef CAS.
- For selected recent reviews on molecular machines including those exploiting photo-switches see:
(a) S. Erbas-Cakmak, D. A. Leigh, C. T. McTernan and A. L. Nussbaumer, Chem. Rev., 2015, 115, 10081–10206 CrossRef CAS PubMed;
(b) V. Blanco, D. A. Leigh and V. Marcos, Chem. Soc. Rev., 2015, 44, 5341–5370 RSC;
(c) L. van Dijk, M. J. Tilby, R. Szpera, O. A. Smith, H. A. P. Bunce and S. P. Fletcher, Nat. Rev. Chem., 2018, 2, 1–18 CrossRef.
- A. A. Beharry and G. A. Woolley, Chem. Soc. Rev., 2011, 40, 4422–4437 RSC.
-
(a) V. Saggiomo, S. Otto, I. Marques, V. Félix, T. Torroba and R. Quesada, Chem. Commun., 2012, 48, 5274–5276 RSC;
(b) N. Busschaert, S. J. Bradberry, M. Wenzel, C. J. E. Haynes, J. R. Hiscock, I. L. Kirby, L. E. Karagiannidis, S. J. Moore, N. J. Wells and J. Herniman,
et al.
, Chem. Sci., 2013, 4, 3036–3045 RSC.
- X. Wu, L. W. Judd, E. N. W. Howe, A. M. Withecombe, V. Soto-Cerrato, H. Li, N. Busschaert, H. Valkenier, R. Pérez-Tomás and D. N. Sheppard,
et al.
, Chem, 2016, 1, 127–146 CAS.
- S. J. Edwards, I. Marques, C. M. Dias, R. A. Tromans, N. R. Lees, V. Félix, H. Valkenier and A. P. Davis, Chem. - Eur. J., 2016, 22, 2004–2011 CrossRef CAS PubMed.
- H. M. Dhammika Bandara and S. C. Burdette, Chem. Soc. Rev., 2012, 41, 1809–1825 RSC.
-
(a) A. A. Beharry, O. Sadovski and G. A. Woolley, J. Am. Chem. Soc., 2011, 133, 19684–19687 CrossRef CAS PubMed;
(b) M. J. Hansen, M. M. Lerch, W. Szymanski and B. L. Feringa, Angew. Chem., Int. Ed., 2016, 55, 13514–13518 CrossRef CAS PubMed;
(c) D. Bléger, J. Schwarz, A. M. Brouwer and S. Hecht, J. Am. Chem. Soc., 2012, 134, 20597–20600 CrossRef PubMed;
(d) S. Samanta, A. A. Beharry, O. Sadovski, T. M. McCormick, A. Babalhavaeji, V. Tropepe and G. A. Woolley, J. Am. Chem. Soc., 2013, 135, 9777–9784 CrossRef CAS PubMed;
(e) M. Dong, A. Babalhavaeji, M. J. Hansen, L. Kálmán and G. A. Woolley, Chem. Commun., 2015, 51, 12981–12984 RSC.
- K. Dąbrowa, P. Niedbała and J. Jurczak, Chem. Commun., 2014, 50, 15748–15751 RSC.
- N. Busschaert, I. L. Kirby, S. Young, S. J. Coles, P. N. Horton, M. E. Light and P. A. Gale, Angew. Chem., Int. Ed., 2012, 51, 4426–4430 CrossRef CAS PubMed.
- A. Antoine John and Q. Lin, J. Org. Chem., 2017, 82, 9873–9876 CrossRef CAS PubMed.
- P. Kuzmič, Anal. Biochem., 1996, 237, 260–273 CrossRef PubMed.
- C. Hansch, A. Leo and R. W. Taft, Chem. Rev., 1991, 91, 165–195 CrossRef CAS.
- Note that the measured EC50Z,PSS is an underestimate of the intrinsic transport ability of 1Z, with the actual activity ∼1.25× higher (EC50 0.8× smaller) due to the incomplete isomerisation to the Z isomer in the PSS.
- E. L. Doyle, C. A. Hunter, H. C. Phillips, S. J. Webb and N. H. Williams, J. Am. Chem. Soc., 2003, 125, 4593–4599 CrossRef CAS PubMed.
- Compatibility of the dose–response curves with the Hill equation with Hill coefficients n > 1 reveals endergonic self-assembly (un-stable supramolecules) and provides information on the stoichiometry; n = 1 or n < 1 reveals exergonic self-assembly (stable supramolecules). See S. Bhosale and S. Matile, Chirality, 2006, 18, 849–856 CrossRef CAS PubMed.
- N. Sakai and S. Matile, J. Phys. Org. Chem., 2006, 19, 452–460 CrossRef CAS.
- Similar results were obtained for in situ photo-isomerisation of 1aE in the HPTS assay. See ESI† for further details.
Footnote |
† Electronic supplementary information (ESI) available. See DOI: 10.1039/d0sc02745f |
|
This journal is © The Royal Society of Chemistry 2020 |