DOI:
10.1039/D0SC02230F
(Edge Article)
Chem. Sci., 2020,
11, 7401-7407
Chimeric oligosaccharide conjugate induces opsonic antibodies against Streptococcus pneumoniae serotypes 19A and 19F†
Received
20th April 2020
, Accepted 23rd June 2020
First published on 26th June 2020
Abstract
Streptococcus pneumoniae 19A (ST19A) and 19F (ST19F) are among the prevalent serotypes causing pneumococcal disease worldwide even after introduction of a 13-valent pneumococcal conjugate vaccine (PCV13). Synthetic glycoconjugate vaccines have defined chemical structures rather than isolated polysaccharide mixtures utilized in marketed vaccines. Ideally, a minimal number of synthetic antigens would cover as many bacterial serotypes to lower cost of goods and minimize the response to carrier proteins. To demonstrate that a chimeric oligosaccharide antigen can induce a protective immune response against multiple serotypes, we synthesized a chimeric antigen (ST19AF) that is comprised of a repeating unit of ST19A and ST19F capsular polysaccharide each. Synthetic glycan epitopes representing only ST19A, and ST19F were prepared for comparison. Semisynthetic glycoconjugates containing chimeric antigen ST19AF induced high antibody titers able to recognize native CPS from ST19A and ST19F in rabbits. The antibodies were able to kill both strains of pneumococci. Chimeric antigens are an attractive means to induce an immune response against multiple bacterial serotypes.
Introduction
Invasive pneumococcal diseases (IPD) caused by Streptococcus pneumoniae (Sp), are a major cause of morbidity and mortality in toddlers and older adults worldwide.2,3 Despite the introduction of a 13-valent pneumococcal conjugate vaccine (PCV13) in 2010, ST19A remains a major pathogen worldwide4–8 that is associated with 14% of all IPD cases.9 Since the efficacy of PCV 13 against ST19A is being debated4,10,11 the development of new vaccine approaches covering ST19A and ST19F is desirable.
Currently glycoconjugate vaccines contain polysaccharides isolated from bacterial cell cultures, that are conjugated to proteins to create heterogeneous vaccine compositions.12 The ST19 CPS is among the most labile polysaccharides used for vaccine production as the phosphate diester groups can be cleaved during purification and conjugation resulting in severely suppressed immunogenicity.13,14 Phosphate diesters in the polysaccharide of Salmonella typhimurium and Clostridium difficile PSII are crucial for inducing a strong immune response to the native polysaccharide.15,16 Synthetic oligosaccharide conjugates containing well-defined antigens, have proven very effective in preventing bacterial infections caused by encapsulated bacteria,17–27 and the conjugation process does not impair antigens having phosphate diester groups. The saccharide to protein ratio (glycan loading) is an important parameter for glycoconjugate vaccine development. The novel strategy described here, helps to reduce the amount of protein used and may simplify the formulation of multicomponent vaccines against bacterial pathogens.
The ST19 CPS are homopolymers that are comprised of a common β-D-ManpNAc-(1 → 4)-α-D-Glcp disaccharide linked to C2 (ST19F 1) or C3 of L-Rha (ST19A 2) respectively (Fig. 1A).28,29 Several oligosaccharide antigens related to ST19A30−32 and ST19F33−36 have been synthesized but neither the anomeric phosphate was stereoselectively constructed nor any biological studies were conducted. The synthesis and immunological evaluation of both antigens is important to establish a better fundamental understanding of the exact epitopes responsible for a protective immune response.
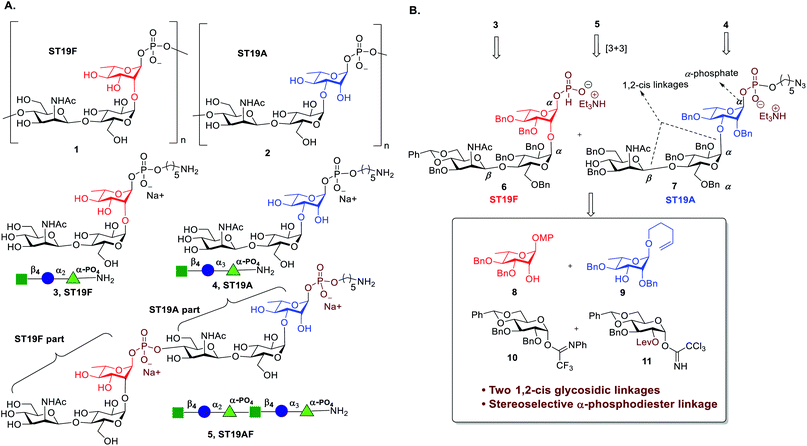 |
| Fig. 1 (A) Structures of the S. pneumoniae CPS repeating units of serotypes ST19F (1), ST19A (2) and synthetic oligosaccharide antigens 3, and 4 that resemble these repeating units as well as chimeric oligosaccharide 5; (B) Retrosynthetic analysis of 3, 4, and 5. | |
Almost 100 S. pneumoniae serotypes can cause IPD.37 Marketed glycoconjugate vaccines contain up to 13 different CPS as new formulations with up to 20 serotypes are under development.38 As more serotypes are included, antigens that can induce a protective immune response against more than one serotype would be particularly valuable. Multivalent vaccines based on glyco-nanoparticles,39 or created by Ugi-multicomponent reactions as well as bivalent unimolecular vaccines have been explored.40 Chimeric antigens that combine the repeating unit sequences of two different serotypes in one oligosaccharide that is then conjugated to a carrier protein have not been reported. We combine ST19F (3) and ST19A (4) via a phosphodiester linkage to form the chimeric oligosaccharide antigen ST19AF (5) (Fig. 1A). A terminal C5-amino linker enables further site-specific conjugation to a carrier protein, to induce protective antibodies against both serotypes using the chimeric antigen (Fig. 1A).
Results and discussion
Antigen construction of 3, 4, 5 requires the stereoselective installation of two 1,2-cis glycosidic linkages and the stereoselective formation of an α-phosphodiester linkage to the trisaccharide (Fig. 1B). Trisaccharides 6 or 7 can be synthesized via a linear synthetic approach from the reducing to the non-reducing end using the building blocks 8,419,4210,43 and 11.44 For the assembly of trisaccharide building block 6, glucosyl imidate 10 was coupled to rhamnosyl acceptor 8 to obtain α-linked disaccharide 12 (1JC–H = 170.5 Hz) in 82% yield (Scheme 1A). Regioselective reductive ring opening of the benzylidene acetal in 12 using triethylsilane and trifluoroacetic acid delivered acceptor 13 in 69% yield. Disaccharide acceptor 13 was glycosylated with glucosyl imidate 11 to afford trisaccharide 14 in 85% yield (doublet, J = 8.0 Hz, 1JC–H = 167.9 Hz). Selective levulinoyl ester (Lev) cleavage (92%) using hydrazine acetate followed by inversion of the 2-hydroxyl group was achieved by conversion of the corresponding sulfonyl diimidazole and subsequent nucleophilic displacement with tetrabutylammonium azide to obtain 16 (82% over two steps). The inversion of stereochemistry was confirmed by examination of the 1H–1H and 13C–1H coupling constants45,46 to H-1 at δ 4.43 (doublet, J = 1.4 Hz) and 1JC–H = 163.2 Hz. Conversion of the azide to the corresponding acetamido group was obtained by treatment with freshly activated Zn–Cu couple to give 17 in 74% yield. Removal of the p-methoxyphenyl group using ceric ammonium nitrite (CAN) gave hemiacetal 18. The installation of a stereoselective H-phosphonate at the reducing end of phosphate diester 19 was achieved with phosphorous acid and an excess of sterically bulky 2-chloro-5,5-dimethyl-2-oxo-1,3,2-dioxaphosphorinane41 in pyridine at 50 °C to obtain thermodynamically more stable α-H-phosphonate 6 (31P NMR: δ 0.91, 1JP,H = 641.8, 3JP,H-1 = 7.5 Hz & 1H NMR: δ 5.70, 1JC,H = 176 Hz). The selectivity may result from conversion of the more reactive β-glycosyl H-phosphonate to the thermodynamically more stable α-phosphonate by SN2 displacement with excess phosphorous acid.47 The protected phosphodiester-linked trisaccharide 19 was prepared by coupling H-phosphonate 6 with 5-azido pentanol using pivaloyl chloride in pyridine as a condensing agent followed by oxidation with iodine in water–pyridine. Hydrogenation of 19 provided deprotected trisaccharide sodium salt 3 after passing through Dowex 50W X4 Na+ resin as a single diastereomer.
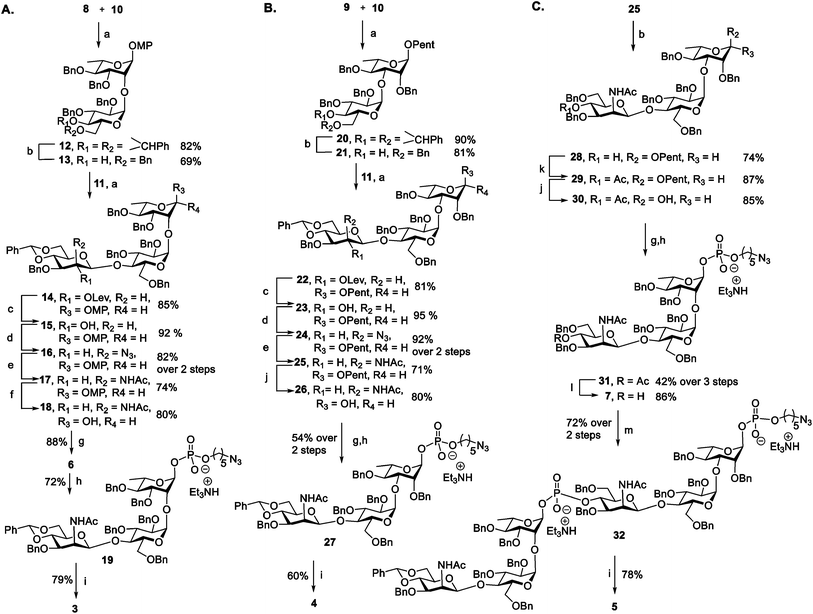 |
| Scheme 1 Synthesis of antigens ST19F 3 (A), ST19A 4 (B) and chimeric ST19AF 5 (C) TMSOTf, CH2Cl2, MS 4 Å, −20 °C, 2 h; (b) Et3SiH, TFA, TFAA, DCM, MS 4 Å, 0 °C – rt; (c) NH2–NH2, AcOH, CH2Cl2–MeOH (3 : 1), rt, 1 h; (d) (1) 1,1-sulfonyldiimidazole, NaH, DMF, −40 °C, 4 h; (2) TBAN3, toluene, 100 °C, 3 h; (e) Zn–Cu Couple, THF : Ac2O : AcOH (3 : 3 : 1), rt, 15 h; (f) CAN, CH3CN–toluene–H2O, rt, 10 h; (g) 2-chloro-1,3,2-benzodioxaphos-yphorin-4-one, H3PO3, Py, rt, 2 h then 50 °C, 36 h; (h) (1) HO(CH2)5N3, PivCl, pyridine, rt, 1.5 h; (2) I2, Py : H2O (18 : 2), −40 °C, 30 min then TEAB; (i) (1) H2, Pd/C, EtOAc : MeOH : H2O, 3 d; (2) Dowex 50W X4, Na+; (j) NBS, CH3CN, 0 °C – rt, 2 h; (k) Ac2O, Py, 0 °C, 1 h; (l) 0.5 M NaOMe, MeOH, rt, 3 h; (m) 6, PivCl, pyridine, rt, 2 h. | |
The synthesis of ST19A trisaccharide 4 followed a similar synthetic approach (Scheme 1B). Orthogonal glycosylation between glucosyl imidate 10 and n-pentenyl acceptor 9 gave α-linked disaccharide 20 (1JC,H = 172.0 Hz), which was subjected to reductive benzylidene acetal cleavage to obtain 21. Disaccharide 21 containing a 4-hydroxyl group was coupled with glycosyl imidate 11 to give trisaccharide 22 (δ 4.42, doublet, J = 8.0 Hz, 1JC–H = 165.2 Hz). Cleavage of the Lev group followed by stereoselective inversion of the C2-hydroxyl group in 23 yielded the corresponding azide 24 (δ 4.29, singlet, 1JC,H = 162.2 Hz). Reduction of the azide to the corresponding acetamido derivative using Zn–Cu couple afforded 25. Hydrolysis of pentenyl glycoside delivered hemiacetal 26, which was converted into the azidopentyl linked phosphonate derivative 27via the H-phosphonate method. Hydrogenation using Pd/C in EtOAc
:
MeOH
:
H2O (3
:
2
:
1) provided deprotected trisaccharide as triethylammonium salt that was converted into corresponding sodium salt using Dowex 50W X4 Na+ resin to obtain white solid 4 as a single diastereomer (δP −1.92, 1JC,H = 174.2 Hz).
With trisaccharides 25 and 6 in hand, the synthesis of the chimeric antigen 5 commenced (Scheme 1C). Reductive cleavage of the benzylidene acetal in trisaccharide 25 was achieved using triethylsilane and TFA to give 28, that was acetylated at the 4-OH to furnish 29. Hydrolysis of the pentenyl glycoside followed by H-phosphonate installation gave solely α-glycosyl phosphate 31 (δP −2.64, 3JP,H-1 = 8.1 Hz, 1JC,H = 176.0 Hz). Acetate ester cleavage furnished acceptor 7 that was condensed with ST19F H-phosphonate 6 to afford hybrid ST19AF 32. Finally, hexasaccharide 32 was deprotected by hydrogenolysis to provide the disodium salt of antigen 5 following exchange with Dowex 50W X4 Na+ resin. The antigens 3, 4, and 5 were obtained as white solids following purification by reverse phase C18-column HPLC and lyophilization.
The synthetic antigens 3–5 were conjugated to CRM197 with an average of seven, five and five oligosaccharides attached per CRM197 molecule respectively as determined by MALDI analysis. Rabbits were immunized with conjugates containing the chimeric ST19AF, or the ST19A or ST19F antigens formulated with aluminum hydroxide. The conjugates induced strong anti-glycan antibody titers after delivery of three vaccine doses at two week intervals. The long term immune response was confirmed following a boost three and a half months after the last immunization (day 133). High antibody titers were faster attained showing that memory B cells were activated and able to generate antibodies against the vaccine antigen (day 144) (Fig. 2). Rabbits immunized with Prevnar13®, a marketed vaccine containing both native CPS serotypes, produced higher antibody titers against the hybrid ST19AF when compared to ST19A and ST19F antigens (Fig. 2). Increased binding to the chimeric oligosaccharide may be a result of an ionic interaction with the phosphate diester connecting the two repeating units. Ionic protein–glycan interactions are known for cell recognition48,49 and antibody–glycan interactions.15
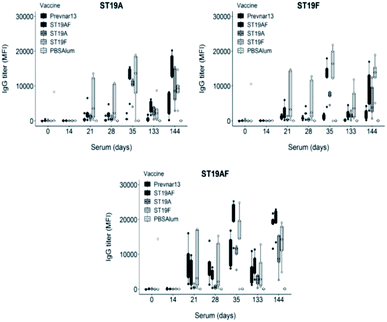 |
| Fig. 2 Semisynthetic glycoconjugates ST19A, ST19F or ST19AF induce antibody production and a long-term memory response. Zika rabbits (n = 5 per group) were immunized four times (days 0, 14, 28, and 133) intramuscularly with semisynthetic glycoconjugate vaccine ST19A, ST19F or ST19AF in aluminum hydroxide as well as positive control Prevnar13® and negative control PBS + aluminum hydroxide. Polysaccharide-specific antibody titers were analyzed by glycan microarray. The 1 : 100 sera dilution was used in the analysis. MFI = mean fluorescence intensity. | |
Rabbit antibodies raised in response to glycoconjugate vaccination were tested against native CPS of ST19A (CPS19A) and ST19F (CPS19F) to determine the cross-reactivity of antibodies produced against synthetic or native antigens. Chimeric ST19AF generated antibodies that recognize both CPS. Anti-CPS19A antibody titer was highest after three doses (day 35). ST19A antigen at the reducing-end of the chimeric structure may favor a better B-cell presentation due to the proximity to the carrier protein presented via MHC class II (Fig. 3).50 ST19F conjugate induced low antibody titers against CPS19F while ST19A did not generate antibodies recognizing native CPS19A although antibodies against the synthetic antigen were detected (Fig. 2). The absence of the phosphate diester in the shorter antigens might impair the generation of antibodies that can recognize native CPS since Prevnar13®, that contains the native repeating units (RU) in varying length, is able to induce a high immune response. Antibodies raised against ST19A and ST19F did not cross react showing the importance of L-rhamnose linkage48,49 in generating specific antibodies against native CPS of different bacteria (Fig. 3).
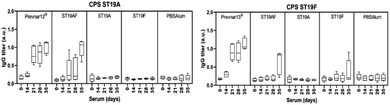 |
| Fig. 3 Antibodies produced in response to semisynthetic glycoconjugate ST19AF recognize native CPS19A and CPS19F. Zika rabbits (n = 5 per group) were immunized three times (days 0, 14, 28) intramuscularly with semisynthetic ST19A, ST19F or ST19AF glycoconjugate formulated with aluminum hydroxide as well as positive control Prevnar13® and negative control PBS + aluminum hydroxide. Polysaccharide-specific antibody titers were analyzed by ELISA. The 1 : 100 sera dilution was used in the analysis. a.u. = absorbance units. | |
Antibody-mediated bacterial killing in vitro, was determined by an opsonophagocytic killing assay (OPKA) with sera of rabbits immunized with three doses of ST19A, ST19F, ST19AF, Prevnar13®, or PBS and aluminium hydroxide (Fig. 4A). The anti-pneumococcal reference serum (007sp) served as standard reference.51 ST19AF glycoconjugate elicits opsonic antibodies able to kill both bacterial serotypes. Anti-ST19F antibodies had a very weak opsonic activity while antibodies induced by the ST19A conjugate were not bactericidal (Fig. 4B), reflecting the weak or absent recognition of native capsular polysaccharide (Fig. 3). The ST19AF serum dilution for 50% bacterial killing is within the range of opsonic activity of the standard sera 007sp. (Fig. 4C). While the antibody titer produced in response to ST19AF was lower than that for Prevnar13® against CPS19F, the opsonic activity of both vaccines were similar. Thus, the quality of antibodies generated with the ST9AF vaccine overcame the reduced antibody titer. Using just one antigen to protect against two serotypes is helpful for reducing the amount of carrier protein in vaccine formulations containing different serotypes.
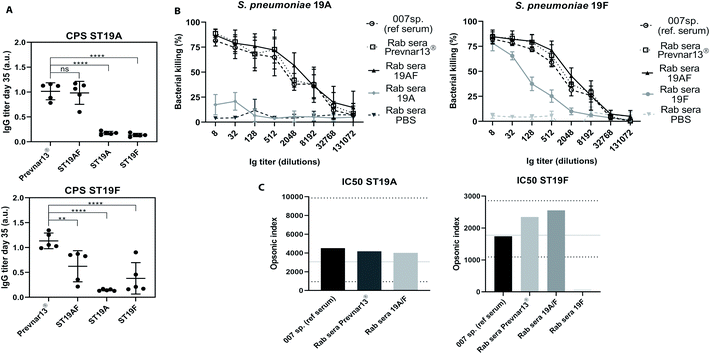 |
| Fig. 4 ST19AF antigen induced antibodies with opsonophagocytic activity. (A) IgG titer of rabbits (n = 5 per group) immunized with Prevnar13® and semisynthetic glycoconjugates ST19AF, ST19A and ST19F after three doses was used in OPKA assay. Antibody titers were compared to the commercially available vaccine Prevnar13® and statistical differences were determined using ANOVA. (B) In vitro OPKA of pooled rabbit sera raised against semisynthetic glycoconjugates ST19A, ST19F and ST19AF. The results were compared to Prevnar13® and to the reference serum 007sp. Rabbits immunized with PBS and aluminum hydroxide was used as a negative control. Data presented as mean ± SD of two independent experiments in duplicate. (C) Opsonic index refers to the sera dilution where 50% of bacteria are killed and was determined by four parameters logistic regression. The OI of ST19AF synthetic glycoconjugate was in accordance to the 007sp. reference serum, where the mean (black line) and 95% CI (dotted lines) are represented.1 a.u. – absorbance unit. ****p < 0.0001, **p < 0.001. | |
Conclusions
We synthesized a chimeric oligosaccharide antigen containing the repeating units of ST19A and ST19F capsular polysaccharides as well as synthetic glycan epitopes resembling ST19A and ST19F. The chimeric antigen glycoconjugate induced an excellent immune response in rabbits. The antibodies produced in response to the chimeric antigen killed ST19A and ST19F bacteria, while the conjugates containing the other glycan epitopes failed to do so. With an increasing number of serotypes to be included in vaccines due to serotype replacement, ideally, each antigen can induce an immune response against more than one serotype, reducing the chemical steps needed for synthetic vaccine production. These findings will expedite glycoconjugate vaccine development as co-formulation studies containing chimeric antigens will help to reduce the amount of carrier protein in semi-synthetic glycoconjugate vaccines.
Materials and methods
Oligosaccharide antigens were synthesized using standard protocols and conjugated to CRM197. Synthetic antigens were printed on NHS-activated microarray slides. Animal experiment (project 49062) was performed in strict accordance with the NIH/OLAW Animal Welfare Assurance, identification number F16-00178 (A5755-01) and was authorized by LALLF MV (Landesamt für Landwirtschaft, Lebensmittelsicherheit und Fischerei Mecklenburg-Vorpommern, Germany) in accordance to TierSchG 7221.3-2-002/19. The immune response was analyzed by glycan microarrays and ELISA. The functional attribute of the immune response was monitored by OPKA using HL-60 cells. Bacteria serotypes ST19A and ST19F were isolates from Charité Infektiologie und Pneumologie department, Universitätsmedizin Berlin. Detailed materials and methods can be found in ESI Appendix.†
Conflicts of interest
None of the authors declare a conflict of interest.
Acknowledgements
We gratefully acknowledge financial support from the Max Planck Society. This work was supported in part by the German Research Foundation (SFB-TR 84, C3 and C6 to M. W. and C8 to P. H. S.), by the German Ministry for Education and Research (e:Med and CAPSyS to M. W.). We thank Prof. Dr Martin Witzenrath and Katrin Reppe from Division of Pulmonary Inflammation, Charité – Universitätsmedizin Berlin for the bacteria serotypes ST19A and ST19F used in the experiments. SRS thanks Maria Bräutigam for helping conjugation. We thank Eva Settels and Olaf Niemeyer for the technical and analytical support.
References
- R. L. Burton, J. Antonello, D. Cooper, D. Goldblatt, K. H. Kim, B. D. Plikaytis, L. Roalfe, D. Wauters, F. Williams, G. L. Xie, M. H. Nahm and M. Akkoyunlu, Clin. Vaccine Immunol., 2017, 24, e00457-16 CrossRef PubMed.
- D. Bogaert, R. De Groot and P. W. Hermans, Lancet Infect. Dis., 2004, 4, 144–154 CrossRef CAS.
- A. E. Bridy-Pappas, M. B. Margolis, K. J. Center and D. J. Isaacman, Pharmacotherapy, 2005, 25, 1193–1212 CrossRef PubMed.
- J. N. Guevara, P. Izurieta and B. Hoet, Expert Rev. Vaccines, 2018, 17, 283–284 CrossRef CAS PubMed.
- Y. C. Hsieh, T. L. Lin, K. Y. Chang, Y. C. Huang, C. J. Chen, T. Y. Lin and J. T. Wang, J. Infect. Dis., 2013, 208, 203–210 CrossRef CAS.
- R. Isturiz, H. L. Sings, B. Hilton, A. Arguedas, R. R. Reinert and L. Jodar, Expert Rev. Vaccines, 2017, 16, 1007–1027 CrossRef CAS.
- S. L. Kaplan, W. J. Barson, P. L. Lin, S. H. Stovall, J. S. Bradley, T. Q. Tan, J. A. Hoffman, L. B. Givner and E. O. Mason Jr, Pediatrics, 2010, 125, 429–436 CrossRef PubMed.
- M. van der Linden, R. R. Reinert, W. V. Kern and M. Imohl, BMC Infect. Dis., 2013, 13, 70 CrossRef PubMed.
- E. Balsells, L. Guillot, H. Nair and M. H. Kyaw, PLoS One, 2017, 12, e0177113 CrossRef.
- M. L. Avila-Aguero, R. Ulloa-Gutierrez, L. H. Falleiros-Arlant and O. Porras, Expert Rev. Vaccines, 2017, 16, 1–4 CrossRef.
- A. Vila-Corcoles, O. Ochoa-Gondar, C. de Diego, E. Satue, M. Aragon, A. Vila-Rovira, F. Gomez-Bertomeu, R. Magarolas, E. Figuerola-Massana, X. Raga, M. O. Perez and F. Ballester, BMC Infect. Dis., 2018, 18, 196 CrossRef.
- C. Jones, An. Acad. Bras. Cienc., 2005, 77, 293–324 CrossRef CAS.
- C. J. Lee, Biologicals, 2002, 30, 97–103 CrossRef CAS.
- N. S. Pujar, N. F. Huang, C. L. Daniels, L. Dieter, M. G. Gayton and A. L. Lee, Biopolymers, 2004, 75, 71–84 CrossRef CAS.
- R. Adamo, M. R. Romano, F. Berti, R. Leuzzi, M. Tontini, E. Danieli, E. Cappelletti, O. S. Cakici, E. Swennen, V. Pinto, B. Brogioni, D. Proietti, C. L. Galeotti, L. Lay, M. A. Monteiro, M. Scarselli and P. Costantino, ACS Chem. Biol., 2012, 7, 1420–1428 CrossRef CAS.
- M. A. Varas, S. Riquelme-Barrios, C. Valenzuela, A. E. Marcoleta, C. Berrios-Pasten, C. A. Santiviago and F. P. Chavez, Front. Cell. Infect. Microbiol., 2018, 8, 8 CrossRef PubMed.
- J. Y. Baek, A. Geissner, D. C. K. Rathwell, D. Meierhofer, C. L. Pereira and P. H. Seeberger, Chem. Sci., 2018, 9, 1279–1288 RSC.
- B. Benaissa-Trouw, D. J. Lefeber, J. P. Kamerling, J. F. Vliegenthart, K. Kraaijeveld and H. Snippe, Infect. Immun., 2001, 69, 4698–4701 CrossRef CAS PubMed.
- M. Emmadi, N. Khan, L. Lykke, K. Reppe, G. P. S, M. P. Lisboa, S. M. Wienhold, M. Witzenrath, C. L. Pereira and P. H. Seeberger, J. Am. Chem. Soc., 2017, 139, 14783–14791 CrossRef CAS.
- G. L. Kim, S. H. Seon and D. K. Rhee, Arch. Pharmacal Res., 2017, 40, 885–893 CrossRef CAS PubMed.
- M. P. Lisboa, N. Khan, C. Martin, F. F. Xu, K. Reppe, A. Geissner, S. Govindan, M. Witzenrath, C. L. Pereira and P. H. Seeberger, Proc. Natl. Acad. Sci. U. S. A., 2017, 114, 11063–11068 CrossRef CAS.
- P. C. McCarthy, A. Sharyan and L. Sheikhi Moghaddam, Vaccines, 2018, 6, 12 CrossRef.
- S. G. Parameswarappa, K. Reppe, A. Geissner, P. Menova, S. Govindan, A. D. J. Calow, A. Wahlbrink, M. W. Weishaupt, B. P. Monnanda, R. L. Bell, L. A. Pirofski, N. Suttorp, L. E. Sander, M. Witzenrath, C. L. Pereira, C. Anish and P. H. Seeberger, Cell Chem. Biol., 2016, 23, 1407–1416 CrossRef CAS PubMed.
- D. Safari, H. A. Dekker, J. A. Joosten, D. Michalik, A. C. de Souza, R. Adamo, M. Lahmann, A. Sundgren, S. Oscarson, J. P. Kamerling and H. Snippe, Infect. Immun., 2008, 76, 4615–4623 CrossRef CAS PubMed.
- B. Schumann, H. S. Hahm, S. G. Parameswarappa, K. Reppe, A. Wahlbrink, S. Govindan, P. Kaplonek, L. A. Pirofski, M. Witzenrath, C. Anish, C. L. Pereira and P. H. Seeberger, Sci. Transl. Med., 2017, 9, eaaf5347 CrossRef.
- R. M. van der Put, T. H. Kim, C. Guerreiro, F. Thouron, P. Hoogerhout, P. J. Sansonetti, J. Westdijk, M. Stork, A. Phalipon and L. A. Mulard, Bioconjugate Chem., 2016, 27, 883–892 CrossRef CAS.
- V. Verez-Bencomo, V. Fernandez-Santana, E. Hardy, M. E. Toledo, M. C. Rodriguez, L. Heynngnezz, A. Rodriguez, A. Baly, L. Herrera, M. Izquierdo, A. Villar, Y. Valdes, K. Cosme, M. L. Deler, M. Montane, E. Garcia, A. Ramos, A. Aguilar, E. Medina, G. Torano, I. Sosa, I. Hernandez, R. Martinez, A. Muzachio, A. Carmenates, L. Costa, F. Cardoso, C. Campa, M. Diaz and R. Roy, Science, 2004, 305, 522–525 CrossRef CAS.
- J. Carlo, Natl. Res. Counc. Can., 1980, 58, 1069–1074 Search PubMed.
- E. Katzenellenbogen and H. J. Jennings, Carbohydr. Res., 1983, 124, 235–245 CrossRef CAS PubMed.
- E. Kaji, Y. Osa, M. Tanaike, Y. Hosokawa, H. Takayanagi and A. Takada, Chem. Pharm. Bull., 1996, 44, 437–440 CrossRef CAS PubMed.
- L. Morelli, S. Fallarini, G. Lombardi, C. Colombo, L. Lay and F. Compostella, Bioorg. Med. Chem., 2018, 26, 5682–5690 CrossRef CAS PubMed.
- L. Panza, F. Ronchetti and L. Toma, Carbohydr. Res., 1988, 181, 242–245 CrossRef CAS.
- E. Bousquet, M. Khitri, L. Lay, F. Nicotra, L. Panza and G. Russo, Carbohydr. Res., 1998, 311, 171–181 CrossRef CAS PubMed.
- M. Nilsson and T. Norberg, J. Chem. Soc., Perkin Trans. 1, 1998, 1699–1704 RSC.
- L. Panza, F. Ronchetti, G. Russo and L. Toma, J. Chem. Soc., Perkin Trans. 1, 1987, 2745–2747, 10.1039/P19870002745.
- G. Soliveri, A. Bertolotti, L. Panza, L. Poletti, C. Jones and L. Lay, J. Chem. Soc., Perkin Trans. 1, 2002, 1, 2174–2181, 10.1039/B205684D.
- K. A. Geno, J. S. Saad and M. H. Nahm, J. Clin. Microbiol., 2017, 55, 1416–1425 CrossRef PubMed.
-
Pfizer, Trial to Evaluate the Safety and Immunogenicity of a 20-valent Pneumococcal Conjugate Vaccine in Pneumococcal Vaccine-naïve Adults, 2020, https://clinicaltrials.gov/ct2/show/NCT03760146?term=NCT03760146%26draw=2%26rank=1, accessed February 20, 2020 Search PubMed.
- M. Vetro, D. Safari, S. Fallarini, K. Salsabila, M. Lahmann, S. Penades, L. Lay, M. Marradi and F. Compostella, Nanomedicine, 2017, 12, 13–23 CrossRef CAS PubMed.
- R. Adamo, Acc. Chem. Res., 2017, 50, 1270–1279 CrossRef CAS PubMed.
- G. Guchhait and A. K. Misra, Tetrahedron: Asymmetry, 2009, 20, 1791–1797 CrossRef CAS.
- C. G. Uriel, A. M. Gómez, J. C. Lopez and B. Fraser-Reid, Synlett, 2003, 2203–2207 CAS.
- L. J. Liotta, R. D. Capotosto, R. A. Garbitt, B. M. Horan, P. J. Kelly, A. P. Koleros, L. M. Brouillette, A. M. Kuhn and S. Targontsidis, Carbohydr. Res., 2001, 331, 247–253 CrossRef CAS PubMed.
- P. Xu, W. Xu, Y. Dai, Y. Yang and B. Yu, Org. Chem. Front., 2014, 1, 405–414 RSC.
- J. Duus, C. H. Gotfredsen and K. Bock, Chem. Rev., 2000, 100, 4589–4614 CrossRef CAS PubMed.
-
L. Nieto and J. Jiménez-Barbero, in Encyclopedia of Biophysics, ed. G. C. K. Roberts, Springer Berlin Heidelberg, Berlin, Heidelberg, 2013, pp. 228–232, DOI:10.1007/978-3-642-16712-6_90.
- L. Morelli and L. Lay, Arkivok, 2012, 2013, 166–184 CrossRef.
- R. J. Blackler, D. W. Evans, D. F. Smith, R. D. Cummings, C. L. Brooks, T. Braulke, X. Liu, S. V. Evans and S. Muller-Loennies, Glycobiology, 2016, 26, 181–192 CrossRef CAS PubMed.
- Q. Zhou, L. Z. Avila, P. A. Konowicz, J. Harrahy, P. Finn, J. Kim, M. R. Reardon, J. Kyazike, E. Brunyak, X. Zheng, S. M. Patten, R. J. Miller and C. Q. Pan, Bioconjugate Chem., 2013, 24, 2025–2035 CrossRef CAS PubMed.
- F. Y. Avci, X. Li, M. Tsuji and D. L. Kasper, Nat. Med., 2011, 17, 1602–1609 CrossRef CAS PubMed.
- D. Goldblatt, B. D. Plikaytis, M. Akkoyunlu, J. Antonello, L. Ashton, M. Blake, R. Burton, R. Care, N. Durant, I. Feavers, P. Fernsten, F. Fievet, P. Giardina, K. Jansen, L. Katz, L. Kierstead, L. Lee, J. Lin, J. Maisonneuve, M. H. Nahm, J. Raab, S. Romero-Steiner, C. Rose, D. Schmidt, J. Stapleton and G. M. Carlone, Clin. Vaccine Immunol., 2011, 18, 1728–1736 CrossRef CAS PubMed.
Footnotes |
† Electronic supplementary information (ESI) available: Experimental procedures of chemistry and biology & spectroscopic data of all new compounds. See DOI: 10.1039/d0sc02230f |
‡ S. R. S. and B. M. S. S. contributed equally to this work. |
|
This journal is © The Royal Society of Chemistry 2020 |