DOI:
10.1039/D0SC00432D
(Edge Article)
Chem. Sci., 2020,
11, 3885-3892
Tandem sequential catalytic enantioselective synthesis of highly-functionalised tetrahydroindolizine derivatives†‡
Received
23rd January 2020
, Accepted 6th March 2020
First published on 12th March 2020
1. Introduction
Pyrrole derivatives are found as a common structural motif within many natural products that can display a wide range of biological activities.1 The related but structurally more complex tetrahydroindolizine framework, which contains a fused pyrrole–piperidine core, is present in a range of naturally-occurring and synthetic bioactive molecules, and has also been utilised within key intermediates for the synthesis of indolizidine alkaloids (Fig. 1).2 A number of catalytic enantioselective methods for synthesising tetrahydroindolizine derivatives have been developed,3 with the majority of enantioselective syntheses focusing on applications towards rhazinilam.2b,3a,b,h,i Catalytic enantioselective methodologies that provide access to polyfunctionalised tetrahydroindolizine architectures from simple starting materials are much less developed, but have the potential to allow greater exploration of 3D chemical space around the tetrahydroindolizine core.4
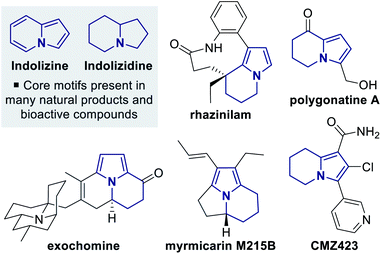 |
| Fig. 1 Tetrahydroindolizine cores present within natural products and bioactive compounds. | |
C(1)-Ammonium enolate catalysis has emerged as a powerful method for the stereocontrolled synthesis of carbocyclic and heterocyclic compounds, through both intra- and intermolecular processes.5 C(1)-Ammonium enolates can be generated in situ from a range of carboxylic acid derivatives and have been most widely applied in formal cycloaddition processes, in which an aldol or Michael addition is followed by a lactonisation or lactamisation event.5a,b Within this field, C(1)-isothiouronium enolates, generated from Lewis basic isothiourea catalysts,6 have been shown to be particularly effective for Michael addition–lactonisation/lactamisation processes (Scheme 1a).7,8 Using either enones or α,β-unsaturated ketimines as the Michael acceptor provides access to dihydropyranone or dihydropyridinone derivatives with excellent stereocontrol. Although a wide variety of Michael acceptors have been applied,7 a limitation for intermolecular processes is the common requirement for the isothiouronium enolate precursor to be an acetic acid derivative bearing an α-aryl or α-alkenyl substituent.
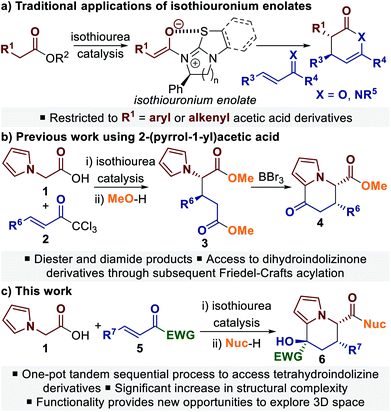 |
| Scheme 1 Applications of isothiouronium enolate catalysis in Michael addition–lactonisation/lactamisation processes. | |
To address this limitation we recently reported the use of 2-(pyrrol-1-yl)acetic acid 1 in an isothiourea-catalysed Michael addition–lactonisation process using trichloromethyl enones 2 (Scheme 1b).9 The dihydropyranone products were unstable, however ring-opening using an alcohol or amine allowed isolation of diester 3 or diamide products with excellent stereoselectivity. Taking advantage of the inherent reactivity of pyrroles, the diester products could be further transformed in a separate reaction step through BBr3-promoted Friedel–Crafts acylation.9,10 Building upon this work, in this manuscript we report the development of a one-pot tandem sequential process11 to generate highly-functionalised tetrahydroindolizine derivatives 6 bearing three stereocentres. It was envisaged that the use of alternative Michael acceptors in an enantioselective Michael addition–lactonisation process, followed by ring-opening of the dihydropyranone to reveal a suitably electrophilic ketone, would allow a spontaneous cyclisation reaction with the pendant pyrrole to take place. Herein we describe the use of trifluoromethyl enones and α-keto-β,γ-unsaturated esters in this process, leading to a range of tetrahydroindolizine derivatives in up to >95
:
5 dr and >99
:
1 er (Scheme 1c).
2. Results and discussion
2.1 Reaction optimisation
Initial studies focussed on optimising the isothiourea-catalysed Michael addition–lactonisation process using 2-(pyrrol-1-yl)acetic acid 1 and trifluoromethyl enone 7 (Table 1).12 Treatment of 2-(pyrrol-1-yl)acetic acid 1 with pivaloyl chloride, to give a mixed anhydride in situ, followed by addition of HyperBTM 11 and enone 7 in MeCN at room temperature gave full conversion to a mixture of three compounds 8–10 (entry 1). Surprisingly, the expected dihydropyranone product 8 was only a minor component (∼20%) and was accompanied by the isomeric dihydropyranone 10 (65%) as the major component as well as β-lactone 9 (∼15%). In previous work using trifluoromethyl enone 7 in combination with aryl acetic acid derivatives,7d,i,n no analogous β-lactone or isomeric dihydropyranone products were observed, indicating significantly different reaction pathways are accessible when using 2-(pyrrol-1-yl)acetic acid 1 as the isothiouronium enolate precursor. Separation of the three compounds was very challenging and therefore conditions for the selective generation of dihydropyranone 8 were targeted. An extensive solvent screen of 25 solvents was conducted, with only selected results summarised in Table 1 (see ESI‡ for full details). Conducting the reaction in DMF provided complete conversion to the isomeric dihydropyranone 10, which was isolated in 97% and 80
:
20 er (entry 2).13 The use of CH2Cl2 at room temperature gave a 20
:
50
:
30 mixture of the three compounds, however conducting the reaction at −60 °C suppressed formation of the isomeric dihydropyranone 10 to give only 8 and 9 in a 50
:
50 ratio (entries 3 and 4). β-Lactone 9 was obtained from this mixture in 40% yield, 95
:
5 dr and 96
:
4 er,14 however dihydropyranone 8 could not be isolated. Ethereal solvents provided significantly enhanced selectivity for dihydropyranone 8 (entries 5–7), with the use of cyclopentylmethyl ether (CPME) giving 8 and 9 in an 85
:
15 ratio (entry 7). From this mixture, dihydropyranone 8 was isolated for the first time, albeit in low yield (18%), but with highly promising stereoselectivity (80
:
20 dr, 94
:
6 er). The low isolated yield was attributed to instability of the product on silica. At this point alternative isothiourea catalysts 12 and 13 were tested, however lower conversion and product selectivity was observed (entries 8 and 9). Further solvent screening using HyperBTM 11 as catalyst identified that acetates were also good solvents (entries 10 and 11), with the use of i-PrOAc providing a 90
:
5
:
5 ratio of 8
:
9
:
10 and allowing isolation of 8 in 20% yield, 80
:
20 dr and 95
:
5 er (entry 11). Although the precise effect of solvent in this reaction cannot be definitively stated, the formation of isomeric dihydropyranone 10 appears to be favoured in solvents with higher dielectric constants (ε ≥ 10).
Table 1 Reaction optimisation I: selectivity for formation of dihydropyranone 8
Intrigued by the formation of β-lactone 9 and isomeric dihydropyranone 10 in these reactions, further studies were conducted to investigate their formation and stability under the reaction conditions. First, a diastereo- and enantioenriched sample of dihydropyranone 8 was treated with HyperBTM 11 and i-Pr2NEt in DMF at room temperature. The isomeric dihydropyranone 10 was obtained as the sole product and isolated in 55% yield and 80
:
20 er (Scheme 2a). Formation of this product can be rationalised by isomerisation of dihydropyranone 8 through a series of tautomerisations. The enantioenriched nature of isomeric dihydropyranone 10 indicates that protonation at C(6) is diastereoselective, with control presumably imparted by the stereocentre at C(3) (Scheme 2a, grey box). This isomerisation process would be expected to be more facile in solvents with higher dielectric constants, consistent with the trends in product ratio observed during reaction optimisation (see Table 1). Next, an isolated sample of racemic β-lactone (±)-9 was treated with HyperBTM 11 and i-Pr2NEt in DMF at room temperature. Once again the isomeric dihydropyranone 10 was obtained as the sole product and isolated in 60% yield and 73
:
27 er (Scheme 2b). Generation of the isomeric dihydropyranone 10 in enantioenriched form may be rationalised by initial transformation of β-lactone (±)-9 to an enantioenriched sample of dihydropyranone 8, followed by the proposed tautomerisation process. The proposed isomerisation of racemic β-lactone (±)-9 to enantioenriched dihydropyranone 8 may be explained by a HyperBTM-promoted formal retro-[2 + 2] cycloaddition to regenerate the isothiouronium enolate and enone 7, which may then recombine to give enantioenriched dihydropyranone 8.
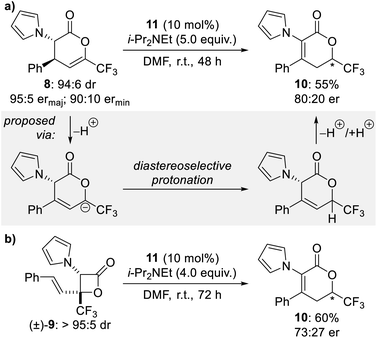 |
| Scheme 2 Control experiments. | |
Having identified CPME and i-PrOAc as the optimal solvents for the generation of enantioenriched dihydropyranone 8, optimisation of the one-pot tandem sequential process to give tetrahydroindolizine derivatives was targeted. Upon completion of the isothiourea-catalysed reaction, methanol and catalytic DMAP was added to initiate the proposed ring-opening–cyclisation process (Table 2). Pleasingly, tetrahydroindolizine 14 was obtained in both solvents in good yield (71–88%) and with high enantioselectivity for the major diastereoisomer (entries 1 and 2). Only two diastereoisomers were observed, indicating high diastereocontrol in the generation of the new stereocentre at C(8). Conducting the reaction at −40 °C provided enhanced stereoselectivity (entries 3 and 4), with the use of i-PrOAc proving optimal to give tetrahydroindolizine 14 in 83% yield, 90
:
10 dr, 98
:
2 er for the major diastereoisomer and 96
:
4 er for the minor diastereoisomer (entry 4).
Table 2 Reaction optimisation II: formation of tetrahydroindolizine 14
The absolute (5S,6S,8R)-configuration of the major diastereoisomer of tetrahydroindolizine was confirmed by single crystal X-ray crystallographic analysis of derivative 21.15 Based on previous literature precedent, the observed configurations at C(5) and C(6) are consistent with those expected to be generated in the isothiourea-catalysed Michael addition–lactonisation process.7d,i,n The absolute configuration generated at C(8) may be rationalised by cyclisation of the ring-opened product 15 proceeding through a chair-like transition state, in which both the phenyl and CF3 groups occupy pseudo-equatorial positions (Fig. 2).16
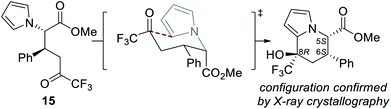 |
| Fig. 2 Rationale for configuration of the major diastereoisomer of tetrahydroindolizine. | |
2.2 Reaction scope and limitations
2.2.1 Trifluoromethyl enone Michael acceptors.
The scope of the transformation was investigated using different nucleophiles to promote ring-opening–cyclisation, and by applying a range of aryl- and heteroaryl-substituted trifluoromethyl enones (Table 3). Variation of the nucleophile demonstrated that amines were equally applicable, with amide-functionalised tetrahydroindolizines 16 and 17 obtained in good yield and with excellent stereoselectivity. The scope with respect to the trifluoromethyl enone was investigated using methanol as nucleophile for the ring-opening–cyclisation sequence. Incorporation of both electron-withdrawing and -donating groups at the 4- and 3-positions of the β-aryl ring of the trifluoromethyl enone was tolerated, with tetrahydroindolizines 18–22 obtained in 40–68% yield, and with good diastereoselectivity and excellent enantioselectivity (96
:
4 to 99
:
1 er). Incorporation of a sterically-imposing ortho-tolyl substituent at the β-position of the enone resulted in a significantly lower yield of tetrahydroindolizine 23 (25%), however excellent enantioselectivity (>99
:
1 er) for the major diastereoisomer was still observed. Electron neutral 1-naphthyl- and 2-naphthyl-substituted enones were also tolerated, giving tetrahydroindolizines 24 and 25 in good yield and excellent er (both 98
:
2 er). Incorporation of a 2-furyl group at the β-position of the enone also led to tetrahydroindolizine 26 in excellent yield and er (95%, 97
:
3 ermajor).
Table 3 Reaction scope I: trifluoromethyl enone Michael acceptorsa
dr determined by 1H and 19F NMR spectroscopic analysis of the crude reaction product mixture, with values rounded to nearest 5; er determined by chiral stationary phase HPLC analysis.
ND = could not be determined.
|
|
2.2.2 α-Keto-β,γ-unsaturated ester Michael acceptors.
Extension of this catalytic tandem sequential approach to tetrahydroindolizine derivatives was targeted by using α-keto-β,γ-unsaturated esters as the Michael acceptor.7a,i A brief optimisation was first conducted (Schemes 3 and 4). Treatment of 2-(pyrrol-1-yl)acetic acid 1 with pivaloyl chloride, to give a mixed anhydride in situ, followed by addition of HyperBTM 11 and α-keto-β,γ-unsaturated ester 27 in MeCN at room temperature led to full conversion to pyranone 28 and diene 29 (Scheme 3a). In contrast to the analogous experiment using trifluoromethyl enone 7 (Table 1, entry 1), no dihydropyranone or β-lactone products were observed. It was suspected that pyranone 28 arose from oxidation of a transiently-formed dihydropyranone, whilst diene 29 most likely originated from decarboxylation of a β-lactone. Conducting the reaction at −40 °C suppressed formation of pyranone 28, with dihydropyranone 30 and diene 29 obtained in a 91
:
9 ratio, however dihydropyranone 30 proved unstable to chromatographic purification (Scheme 3b). The use of alternative solvents provided no further improvement in product distribution.
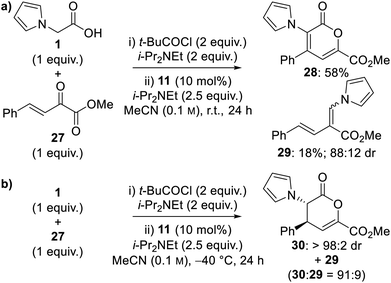 |
| Scheme 3 Initial optimisation using α-keto-β,γ-unsaturated ester 27. | |
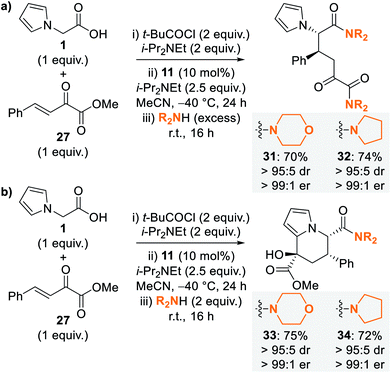 |
| Scheme 4 Optimisation of ring-opening–cyclisation process using amines. | |
Due to the difficulties in isolating dihydropyranone 30, optimisation of the nucleophile-promoted ring-opening–cyclisation process to give tetrahydroindolizine derivatives was probed. Following completion of the isothiourea-catalysed reaction, addition of methanol as nucleophile provided a complex mixture of products. In contrast, the use of excess secondary amines (morpholine and pyrrolidine) provided diamides 31 and 32 as the sole products in high yield and with excellent stereoselectivity (>95
:
5 dr, >99
:
1 er) (Scheme 4a). To favour cyclisation to give tetrahydroindolizine derivatives, the equivalents of amine nucleophile were reduced, with the use of 2 equivalents proving optimal to give tetrahydroindolizines 33 and 34 in high yield and excellent stereocontrol (>95
:
5 dr, >99
:
1 er) (Scheme 4b).
The scope of the transformation was probed using a range of α-keto-β,γ-unsaturated esters, and employing morpholine (2 equiv.) as the nucleophile in the ring-opening–cyclisation process (Table 4). In all cases the tetrahydroindolizine products were obtained with consistently excellent diastereo- and enantioselectivity (>95
:
5 dr and >99
:
1 er in each case). Variation of the ester of the α-keto-β,γ-unsaturated ester to ethyl and isopropyl ester derivatives was tolerated, giving tetrahydroindolizines 35 and 36 in good yield (57% and 64%). Variation of the γ-aryl substituent demonstrated that the incorporation of both electron-donating and -withdrawing groups was well tolerated and provided tetrahydroindolizines 37–43 in moderate to excellent yield (50–98%). The incorporation of ortho-substituted and heteroaromatic γ-aryl substituents was also successful to give 44 and 45 in 58% and 62% yield, respectively. Suitable single crystals could not be obtained to unambiguously assign the configuration of these tetrahydroindolizine products, however based on literature precedent7a,i and similarities in 1H coupling constants,12 the absolute and relative configurations were assigned by analogy to the trifluoromethyl-substituted tetrahydroindolizine series.
Table 4 Reaction scope II: α-keto-β,γ-unsaturated ester Michael acceptorsa
dr determined by 1H NMR spectroscopic analysis of the crude reaction product mixture, with values rounded to nearest 5; er determined by chiral stationary phase HPLC analysis.
CH2Cl2 used as solvent due to low solubility of α-keto-β,γ-unsaturated ester in MeCN.
|
|
2.3 Proposed mechanism
The mechanism of this reaction can be proposed based on previous related studies7 and the results presented in this manuscript (Scheme 5). The isothiourea-catalysed Michael addition–lactonisation is initiated by N-acylation of the Lewis basic isothiourea by mixed anhydride 46, which is generated in situ from 2-(pyrrol-1-yl)acetic acid 1 and pivaloyl chloride, to give acyl ammonium 47 (Scheme 5, Step 1). Deprotonation to give ammonium enolate 48 is most likely facilitated by the pivalate counterion,7d,14 with i-Pr2NEt operating as an auxiliary base to buffer the pivalic acid by-product. Ammonium enolate 48 then undergoes stereoselective Michael addition to enone 5 to give zwitterionic intermediate 49, which following lactonisation gives dihydropyranone 50 and regenerates the isothiourea catalyst. The stereochemical outcome of the reaction is determined in the Michael addition step, and can be rationalised through C–C bond formation between the Si face of the ammonium enolate and the Si face of the enone (Scheme 5, top right box). Reaction on the Si face of the ammonium enolate can be explained through three factors: (i) generation of the (Z)-ammonium enolate; (ii) a stabilising 1,5-O⋯S interaction favouring a syn-coplanar orientation between the enolate O and the S embedded within the catalyst;17,18 and (iii) the pseudo-axial phenyl substituent of the catalyst blocking the Re face of the enolate. The diastereoselectivity can be explained by adoption of a staggered transition state in which non-bonding steric contacts are minimised (Scheme 5, top right box). Following the catalytic reaction, the ring-opening–cyclisation process is initiated through addition of a nucleophilic amine or alcohol to give intermediate 51 (Scheme 5, Step 2). Spontaneous cyclisation of the pyrrole onto the electrophilic ketone within intermediate 51 is proposed to proceed through a chair-like transition state, in which both the R1 group and ketone substituent occupy pseudo-equatorial positions, to give the final tetrahydroindolizine product 6.
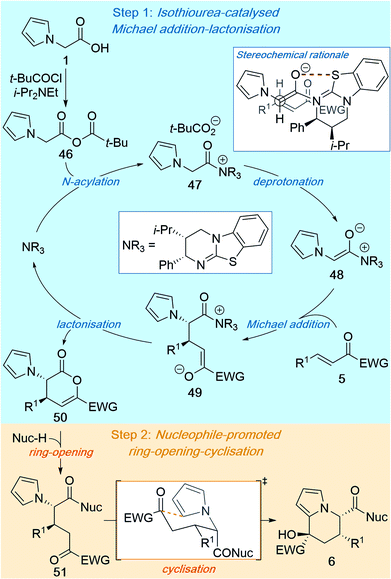 |
| Scheme 5 Proposed mechanism. | |
3. Conclusions
In conclusion, an isothiourea-catalysed one-pot tandem sequential process has been developed for the enantioselective synthesis of highly-functionalised tetrahydroindolizine derivatives. The application of 2-(pyrrol-1-yl)acetic acid, with a suitable Michael acceptor, in an enantioselective Michael addition–lactonisation process was followed by ring-opening with an alcohol or amine. The transient ring-opened intermediate underwent spontaneous cyclisation of the pyrrole onto the pendant ketone to give tetrahydroindolizine products bearing 3 stereogenic centres. The use of trifluoromethyl enones as the Michael acceptor led to 12 tetrahydroindolizine products, bearing a CF3 group at a stereogenic centre, in 25–95% yield and up to 95
:
5 dr and 99
:
1 er. The use of α-keto-β,γ-unsaturated esters provided a further 12 tetrahydroindolizines in 50–98% yield, with each product obtained essentially as a single stereoisomer (>95
:
5 dr, >99
:
1 er). Current work is focused on further applications using 2-(pyrrol-1-yl)acetic acid, and other α-amino acetic acid derivatives, in isothiourea-catalysed enantioselective transformations.
Author contributions
S. Z. conducted all synthetic work. M. D. G. prepared graphics and wrote the manuscript. A. M. Z. S. conducted X-ray crystal structure analysis and refinement. A. D. S. conceptualised and supervised the project, and provided critical feedback during manuscript preparation.
Conflicts of interest
There are no conflicts to declare.
Acknowledgements
The research leading to these results (S. Z.) has received funding from the ERC under the European Union's Seventh Framework Programme (FP7/2007–2013)/ERC grant agreement no 279850. A. D. S. thanks the Royal Society for a Wolfson Research Merit Award. We also thank the EPSRC UK National Mass Spectrometry Facility at Swansea University.
Notes and references
-
(a) I. S. Young, P. D. Thornton and A. Thompson, Nat. Prod. Rep., 2010, 27, 1801–1839 RSC;
(b) V. Bhardwaj, D. Gumber, V. Abbot, S. Dhiman and P. Sharma, RSC Adv., 2015, 5, 15233–15266 RSC.
-
(a)
J. P. Michael, in The Alkaloids: Chemistry and Biology, ed. H.-J. Knölker, Elsevier Inc., Amsterdam, 2016, ch. 1, pp. 1–498 Search PubMed;
(b) I. Kholod, O. Vallet, A.-M. Buciumas and R. Neier, Heterocycles, 2011, 82, 917–948 CAS;
(c) A. Dinsmore, K. Mandy and J. P. Michael, Org. Biomol. Chem., 2006, 4, 1032–1037 RSC;
(d) M. Timmermans, J.-C. Braekman, D. Daloze, J. M. Pasteels, J. Merlin and J.-P. Declercq, Tetrahedron Lett., 1992, 33, 1281–1284 CrossRef CAS;
(e) Q. Huang, A. B. Attygalle, J. Meinwald, M. A. Houck and T. Eisner, J. Nat. Prod., 1998, 61, 598–601 CrossRef CAS PubMed;
(f) A. E. Ondrus and M. Movassaghi, Chem. Commun., 2009, 4151–4165 RSC;
(g) R. Snoeck, G. Andrei, B. Bodaghi, L. Lagneaux, D. Daelemans, E. de Clercq, J. Neyts, D. Schols, L. Naesens, S. Michelson, D. Bron, M. J. Otto, A. Bousseau, C. Nemecek and C. Roy, Antiviral Res., 2002, 55, 413–424 CrossRef CAS.
-
(a) M. G. Banwell, D. A. S. Beck and A. C. Willis, ARKIVOC, 2006, 163–174 Search PubMed;
(b) Z. Liu, A. S. Wasmuth and S. G. Nelson, J. Am. Chem. Soc., 2006, 128, 10352–10353 CrossRef CAS PubMed;
(c) J. C. Conrad, J. Kong, B. N. Laforteza and D. W. C. MacMillan, J. Am. Chem. Soc., 2009, 131, 11640–11641 CrossRef CAS PubMed;
(d) N. Ortega, D.-T. D. Tang, S. Urban, D. Zhao and F. Glorius, Angew. Chem., Int. Ed., 2013, 52, 9500–9503 CrossRef CAS PubMed;
(e) X.-W. Du, A. Ghosh and L. M. Stanley, Org. Lett., 2014, 16, 4036–4039 CrossRef CAS PubMed;
(f) J. Day, B. McKeever-Abbas and J. Dowden, Angew. Chem., Int. Ed., 2016, 55, 5809–5813 CrossRef CAS PubMed;
(g) Y. Zhou, X.-W. Liu, Q. Gu and S.-L. You, Synlett, 2016, 27, 586–590 CrossRef CAS;
(h) V. Magné, C. Lorton, A. Marinetta, X. Guinchard and A. Voituriez, Org. Lett., 2017, 19, 4794–4797 CrossRef PubMed;
(i) A. Shemet and E. M. Carreira, Org. Lett., 2017, 19, 5529–5532 CrossRef CAS PubMed;
(j) D. Zhang, L. Lin, J. Yang, X. Liu and X. Feng, Angew. Chem., Int. Ed., 2018, 57, 12323–12327 CrossRef CAS PubMed.
-
(a) F. Lovering, J. Bikker and C. Humblet, J. Med. Chem., 2009, 52, 6752–6756 CrossRef CAS PubMed;
(b) F. Lovering, MedChemComm, 2013, 4, 515–519 RSC.
-
(a)
K. N. Van, L. C. Morrill, A. D. Smith and D. Romo, in Lewis Base Catalysis in Organic Synthesis, ed. E. Vedejs and S. E. Denmark, Wiley-VCH, Weinheim, 2016, ch. 13, vol. 2, pp. 527–653 Search PubMed;
(b) L. C. Morrill and A. D. Smith, Chem. Soc. Rev., 2014, 43, 6214–6226 RSC;
(c) M. J. Gaunt and C. C. C. Johansson, Chem. Rev., 2007, 107, 5596–5605 CrossRef CAS PubMed.
- J. Merad, J.-M. Pons, O. Chuzel and C. Bressy, Eur. J. Org. Chem., 2016, 2016, 5589–5610 CrossRef CAS.
-
(a) D. Belmessieri, L. C. Morrill, C. Simal, A. M. Z. Slawin and A. D. Smith, J. Am. Chem. Soc., 2011, 133, 2714–2720 CrossRef CAS PubMed;
(b) C. Simal, T. Lebl, A. M. Z. Slawin and A. D. Smith, Angew. Chem., Int. Ed., 2012, 51, 3653–3657 CrossRef CAS PubMed;
(c) D. Belmessieri, D. B. Cordes, A. M. Z. Slawin and A. D. Smith, Org. Lett., 2013, 15, 3472–3475 CrossRef CAS PubMed;
(d) L. C. Morrill, J. Douglas, T. Lebl, A. M. Z. Slawin, D. J. Fox and A. D. Smith, Chem. Sci., 2013, 4, 4146–4155 RSC;
(e) D. Belmessieri, A. de la Houpliere, E. D. D. Calder, J. E. Taylor and A. D. Smith, Chem.–Eur. J., 2014, 20, 9762–9769 CrossRef CAS PubMed;
(f) P.-P. Yeh, D. S. B. Daniels, D. B. Cordes, A. M. Z. Slawin and A. D. Smith, Org. Lett., 2014, 16, 964–967 CrossRef CAS PubMed;
(g) S. R. Smith, S. M. Leckie, R. Holmes, J. Douglas, C. Fallan, P. Shapland, D. Pryde, A. M. Z. Slawin and A. D. Smith, Org. Lett., 2014, 16, 2506–2509 CrossRef CAS PubMed;
(h) L. C. Morrill, D. G. Stark, J. E. Taylor, S. R. Smith, J. A. Squires, A. C. A. D'Hollander, C. Simal, P. Shapland, T. J. C. O'Riordan and A. D. Smith, Org. Biomol. Chem., 2014, 12, 9016–9027 RSC;
(i) L. C. Morrill, L. A. Ledingham, J.-P. Couturier, J. Bickel, A. D. Harper, C. Fallan and A. D. Smith, Org. Biomol. Chem., 2014, 12, 624–636 RSC;
(j) P.-P. Yeh, D. S. B. Daniels, C. Fallan, E. Gould, C. Simal, J. E. Taylor, A. M. Z. Slawin and A. D. Smith, Org. Biomol. Chem., 2015, 13, 2177–2191 RSC;
(k) D. G. Stark, L. C. Morrill, D. B. Cordes, A. M. Z. Slawin, T. J. C. O'Riordan and A. D. Smith, Chem.–Asian J., 2016, 11, 395–400 CrossRef CAS PubMed;
(l) D. G. Stark, P. Williamson, E. R. Gayner, S. F. Musolino, R. W. F. Kerr, J. E. Taylor, A. M. Z. Slawin, T. J. C. O'Riordan, S. A. MacGregor and A. D. Smith, Org. Biomol. Chem., 2016, 14, 8957–8965 RSC;
(m) D. G. Stark, C. M. Young, T. J. C. O'Riordan, A. M. Z. Slawin and A. D. Smith, Org. Biomol. Chem., 2016, 14, 8068–8073 RSC;
(n) C. M. Young, D. G. Stark, T. H. West, J. E. Taylor and A. D. Smith, Angew. Chem., Int. Ed., 2016, 55, 14394–14399 CrossRef CAS PubMed;
(o) J. Izquierdo and M. A. Pericàs, ACS Catal., 2016, 6, 348–356 CrossRef CAS;
(p) R. M. Neyyappadath, D. B. Cordes, A. M. Z. Slawin and A. D. Smith, Chem. Commun., 2017, 53, 2555–2558 RSC;
(q) S. Wang, J. Izquierdo, C. Rodríguez-Escrich and M. A. Pericàs, ACS Catal., 2017, 7, 2780–2785 CrossRef CAS;
(r) C. M. Young, J. E. Taylor and A. D. Smith, Org. Biomol. Chem., 2019, 17, 4747–4752 RSC;
(s) L.-L. Li, D. Ding, J. Song, Z.-Y. Han and L.-Z. Gong, Angew. Chem., Int. Ed., 2019, 58, 7647–7651 CrossRef CAS PubMed;
(t) T. Fan, Z.-J. Zhang, Y.-C. Zhang and J. Song, Org. Lett., 2019, 21, 7897–7901 CrossRef CAS PubMed.
- For an example of a higher-order formal cycloaddition, see: S. Wang, C. Rodríguez-Escrich and M. A. Pericàs, Angew. Chem., Int. Ed., 2017, 56, 15068–15072 CrossRef CAS PubMed.
- S. Zhang, J. E. Taylor, A. M. Z. Slawin and A. D. Smith, Org. Lett., 2018, 20, 5482–5485 CrossRef CAS PubMed.
- R. I. J. Amos, B. S. Gourlay, P. P. Molesworth, J. A. Smith and O. R. Sprod, Tetrahedron, 2005, 61, 8226–8230 CrossRef CAS.
- S. E. Denmark and A. Thorarensen, Chem. Rev., 1996, 96, 137–165 CrossRef CAS PubMed.
- See the ESI‡ for more details.
- The absolute configuration of the isomeric dihydropyranone 10 could not be confirmed.
- The relative and absolute configuration of β-lactone 9 was tentatively assigned by analogy to a previous isothiourea-catalysed synthesis of perfluoroalkylated β-lactones, see: D.-J. B. Antúnez, M. D. Greenhalgh, A. C. Brueckner, D. M. Walden, P. Elías-Rodríguez, P. Roberts, B. G. Young, T. H. West, A. M. Z. Slawin, P. H.-Y. Cheong and A. D. Smith, Chem. Sci., 2019, 10, 6162–6173 RSC.
- Crystallographic data for (5S,6S,8R)-21 (CCDC 1978281).‡.
- Suitable single crystals could not be obtained for the minor diastereoisomer, however comparison of 1H coupling constants for both diastereoisomers indicates a difference in relative configuration between C(5) and C(6). See the ESI‡ for further information.
-
(a) D. J. Pascoe, K. B. Ling and S. L. Cockroft, J. Am. Chem. Soc., 2017, 139, 15160–15167 CrossRef CAS PubMed;
(b) P. Liu, X. Yang, V. B. Birman and K. N. Houk, Org. Lett., 2012, 14, 3288–3291 CrossRef CAS PubMed;
(c) E. R. T. Robinson, D. M. Walden, C. Fallan, M. D. Greenhalgh, P. H.-Y. Cheong and A. D. Smith, Chem. Sci., 2016, 7, 6919–6927 RSC;
(d) T. H. West, D. M. Walden, J. E. Taylor, A. C. Brueckner, R. C. Johnston, P. H.-Y. Cheong, G. C. Lloyd-Jones and A. D. Smith, J. Am. Chem. Soc., 2017, 139, 4366–4375 CrossRef CAS PubMed;
(e) C. M. Young, A. Elmi, D. J. Pascoe, R. K. Morris, C. McLaughlin, A. M. Woods, A. B. Frost, A. de la Houpliere, K. B. Ling, T. K. Smith, A. M. Z. Slawin, P. H. Willoughby, S. L. Cockroft and A. D. Smith, Angew. Chem., Int. Ed., 2020, 59, 3705–3710 CrossRef CAS PubMed.
- For reviews on the nature and use of chalcogen–chalcogen interactions see:
(a) A. J. Mukherjee, S. S. Zade, H. B. Singh and R. B. Sunoj, Chem. Rev., 2010, 110, 4357–4416 CrossRef CAS PubMed;
(b) B. R. Beno, K.-S. Yeung, M. D. Bartberger, L. D. Pennington and N. A. Meanwell, J. Med. Chem., 2015, 58, 4383–4438 CrossRef CAS PubMed;
(c) R. Gleiter, G. Haberhauer, D. B. Werz, F. Rominger and C. Bleiholder, Chem. Rev., 2018, 118, 2010–2041 CrossRef CAS PubMed;
(d) J. Bamberger, F. Ostler and O. G. Mancheño, ChemCatChem, 2019, 11, 5198–5211 CrossRef CAS PubMed.
|
This journal is © The Royal Society of Chemistry 2020 |